Abstract
We measured the size distribution of positively and negatively charged nanoparticles generated abundantly in the gas phase during the syntheses of ZnO nanowires by a carbothermal reduction process using a differential mobility analyzer. Under the conditions where these charged nanoparticles were not generated, no nanowires could be grown. The evolution of ZnO nanostructures on the substrate was studied with in-situ measurements of the size distribution of charged nanoparticles with varying reactor temperature and oxygen flow rate. The results suggest that the electrostatic energy arising from charged nanoparticles would play a critical role in the growth of ZnO nanowires.
INTRODUCTION
ZnO nanostructures have stimulated great interest for its catalytic, electrical, optoelectronic and photochemical properties (CitationKong et al. 2003). Various mechanisms such as vapor-liquid-solid (VLS) growth, vapor-solid (VS) growth and redox growth were proposed to understand their growth (CitationFu et al. 2007). However, the mechanisms have not been fully understood in spite of intense research over the past decade. Recently, a new growth mechanism of ZnO nanostructures based on the electrostatic energy was suggested. CitationKong et al. (2004) reported the charging model for the ZnO nanobelts and nanorings. They suggested that the electrostatic energy plays a critical role in the growth of ZnO nanorings by a spontaneous self-coiling process of polar nanobelts (CitationWang 2004). CitationParkansky et al. (2006) reported a novel method for the growth of ZnO nanorods by applying an electric field to the ZnO film at low temperature.
On the other hand, by photon correlation spectroscopy, CitationMichael et al. (1999) confirmed the existence of the crystalline zinc particles that were produced from a heater in a vacuum chamber containing argon. And in the plasma-enhanced CVD process CitationLiu et al. (2004) observed that ZnO rods grew only when the nanoparticles existed on the sapphire substrate, which served as seeds for the growth. Recently, CitationKim et al. (2007) showed that the charged nanoparticles were generated during the syntheses of silicon films, ZnO nanowires and carbon nanotubes (CNTs) by thermal CVD by measuring the size distribution using a differential mobility analyzer (DMA). These measurements were done based on the previous study of CitationHwang et al. (Hwang et al. 1996; CitationHwang et al. 2000; CitationHwang & Kim 2004; CitationHuh et al. 2005; CitationSong et al. 2007), who suggested that these charged nanoparticles should be a main flux for the growth of films, nanowires, and nanotubes. The generation of charged nanoparticles in the gas phase was experimentally confirmed in various CVD processes (CitationCheong et al. 1999; CitationJeon et al. 1999; CitationJeon et al. 2000; CitationJeon et al. 2001; CitationAhn et al. 2002; CitationKim et al. 2006). A concept similar to the two-step growth was suggested by CitationOstrikov (2005) in the silane plasma CVD process. It is well known that charged nanoparticles are formed in the gas phase and suspended during plasma CVD, which is called “dusty plasma” (CitationOstrikov 2005; CitationOstrikov and Murphy 2007). CitationOstrikov (2005) made an extensive review in the incorporation of gas phase nuclei into nanostructures for a reactive-plasma-assisted nanoassembly process and indicated the importance of detection and control for a building block.
In order to explain the one-dimensional growth of nanowires and nanotubes prepared by CVD in the absence of catalytic metal particles, CitationHwang et al. (Hwang et al. 2000; CitationHwang & Kim 2004) suggested the electrostatic energy arising from the charged clusters or nanoparticles generated in the CVD processes as a main driving force. Considering these results, the electrostatic energy that was suggested to play a critical role in the growth of ZnO nanostructures such as nanobelts and nanorings (CitationKong et al. 2004) might be originated from the charge carried by the nanoparticles. The first step of this possibility would be to confirm the generation of charged ZnO nanoparticles during the synthesis of ZnO nanowires. Motivated by this background, here we tried to confirm experimentally the generation of charged nanoparticles in the gas phase during the synthesis of ZnO nanowires by the carbothermal reduction process using a differential mobility analyzer (DMA) connected to the reactor.
EXPERIMENT
ZnO nanowires were synthesized using a typical carbothermal reduction process. 2 g of ZnO (99.9%) powder and 2 g of graphite powder (99.99%) were mixed. 4 g of the resultant mixture in an alumina boat was placed at the center of a quartz-tube furnace. Above the alumina boat, a silicon substrate coated with 50Å-thick gold was loaded. High purity nitrogen gas (99.9999%) was supplied as a carrier gas together with four different oxygen flow rates of 0, 2, 10, and 90 standard cubic centimeter per minute (sccm). The total flow rate was fixed as 1000 sccm. For in-situ measurements of the size distribution of charged nanoparticles, we set up a differential mobility analyzer (DMA; TSI model 3085) system (CitationKnutson & Whitby 1975; CitationMoisala et al. 2005) designed to measure the size range of 1 ∼100 nm. The size-classified charged particles are detected as a current on a Faraday cup electrometer (FCE). Normally, particles are made to be electrically charged by the artificial charging system before DMA measurements because a DMA can measure only charged particles. However, our measuring system did not use an artificial charger. Using this DMA-FCE system, the size distribution of the charged nanoparticles was measured in-situ during the synthesis of ZnO nanowires as a function of reaction temperature and oxygen flow rate at atmospheric pressure. In the experiment, the aerosol flow rate of the DMA was 900 sccm and the excess aerosol flow was purged, while the sheath air flow rate of the DMA was maintained at 15000 sccm. The schematic diagram of DMA-FCE system connected to the CVD reactor is shown in . The deposited nanostructures were examined by scanning electron microscopy (SEM, JEOL JSM-7401F).
RESULTS AND DISCUSSION
In a carbothermal reduction process, it has been reported that ZnO nanowires can be fabricated at a furnace temperature above 900°C (Kong et al. 2006; CitationWang et al. 2005; CitationPark & Park 2005). Thus, to investigate the effect of furnace temperature on the formation of ZnO nanoparticles in the gas phase and on the deposited ZnO nanostructures, the furnace temperature was varied from 800 to 1000°C at a total gas flow rate of 1000 sccm with an oxygen flow rate of 10 sccm. shows the FE-SEM images of ZnO nanostructures formed at different furnace temperatures. At a furnace temperature of 800°C, no nanowire was produced as shown in , which shows the formation of gold nanoparticles from the film deposited by sputtering. When the furnace temperature increased to 900°C (), nanowires were produced with a diameter of ∼100 nm. As the furnace temperature increased to 1000°C (), nanosheets began to form along the basal nanowires but the diameter of nanowires was almost the same as that formed at 900°C.
FIG. 2 FE-SEM images of ZnO nanowires at furnace temperatures of (a) 800°C, (b) 900°C, and (c) 1000°C and at an oxygen flow rate of 10 sccm.
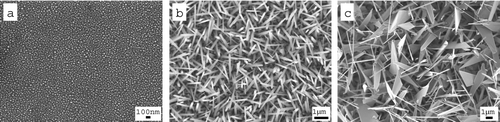
During the synthesis of ZnO nanostructures, in-situ measurements of charged nanoparticles were made by the DMA-FCE system. shows the size distribution for ZnO charged nanoparticles generated in the gas phase at various furnace temperatures. Both positively and negatively charged nanoparticles are generated under the typical processing conditions of ZnO nanostructures such as nanowires. At the furnace temperature below 850°C, charged nanoparticles were not detected. The detection limitation of our measuring system could be one reason for no detection of charged nanoparticles at furnace temperature of 800°C. shows that both positively and negatively charged nanoparticles were generated at the furnace temperature of 850°C and had a single peak at 4 ∼ 5 nm. With increasing furnace temperature above 900°C, both positively and negatively charged nanoparticles tend to have a bimodal size distribution. Most nanoparticles are smaller than 10 nm. At all temperatures, the number concentration of negatively charged nanoparticles was higher than that of the positively charged ones. The particle number concentration of the negatively charged nanoparticles increased with increasing furnace temperature, while that of the positively charged ones tended to decrease, passing through a minimum as the furnace temperature increased. However, the particle diameter at the peak did not change sensitively with the furnace temperature.
FIG. 3 The size distribution of (a) positively and (b) negatively charged ZnO nanoparticles at various furnace temperatures and at an oxygen flow rate of 10 sccm.
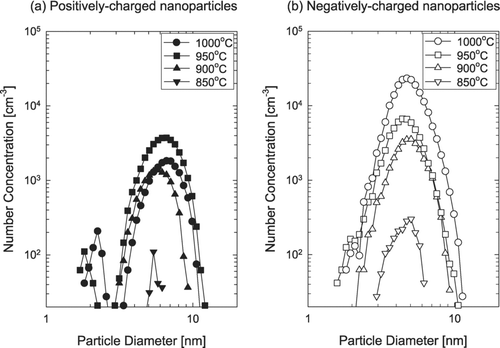
In the synthesis of ZnO nanowires, the morphology of ZnO nanowires is known strongly to depend on the oxygen flow rate (CitationFu et al. 2007) and various fascinating ZnO nanostructures (CitationPark & Park 2006)were produced by controlling the oxygen flow rate. In order to examine the effect of oxygen flow rate on the evolution of ZnO nanostructures and on the size distribution of charged nanoparticles, depositions were done on the silicon substrate with in-situ measurements of charged nanoparticles by DMA-FCE under oxygen flow rates of 0, 2, 10, and 90 sccm at a furnace temperature of 1000°C. When the oxygen gas was not additionally supplied into a reactor, only nanowires were formed as shown in . When the oxygen gas was supplied at 2 and 10 sccm, nanosheets started to form as shown in and . When the flow rate was increased to 90 sccm, very rugged structure was formed as shown in .
FIG. 4 FE-SEM images of ZnO nanostructures at oxygen flow rates of (a) 0, (b) 2, (c) 10, and (d) 90 sccm and at the furnace temperature of 1000°C.
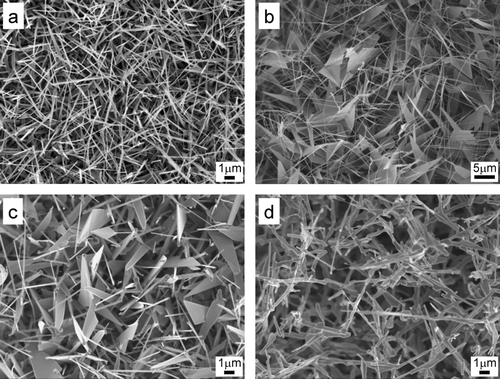
shows the size distribution of charged ZnO nanoparticles for different oxygen flow rates at a furnace temperature of 1000°C. As the oxygen content increased, the size and the number concentration of charged nanoparticles decreased appreciably. It is possible that the increase in size and number concentration of charged nanoparticles in the absence of additional supply of oxygen comes from the enhanced evaporation rate for Zn in the gas phase. This aspect is in agreement with the expectation that the rate of the reduction reaction, ZnO + C → Zn + CO, should be increased at low oxygen partial pressure. Considering these, the nanowires and the charged nanoparticles formed without the additional oxygen supply would be oxygen-deficient compared to those formed with the additional oxygen supply.
FIG. 5 The size distribution of (a) positively and (b) negatively charged ZnO nanoparticles at oxygen flow rates of 0, 2, 10, and 90 sccm and at the furnace temperature of 1000°C.
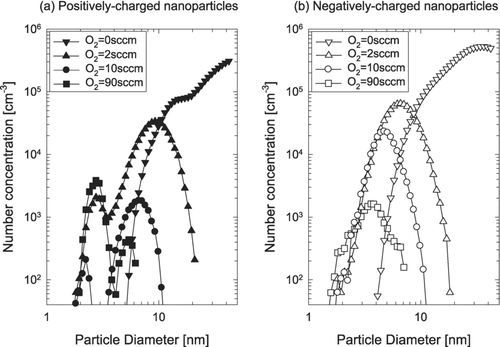
The change of ZnO nanostructures with varying oxygen flow rate might be related with two factors. One is the change of electrical properties of ZnO with the stoichiometry change by oxygen content. The other is the change in the size and the number concentration of charged nanoparticles. Further systematic studies are needed to understand the effect of the oxygen flow rate on the evolution of ZnO nanostructures.
There are two possible mechanisms for the formation of charged nanoparticles measured in and . One is that ions are formed first by surface ionization in the reactor and nanoparticles are formed in the gas phase by ion-induced nucleation, which is the case of formation of charged nanoparticles in hot filament CVD (CitationHwang et al. 1996; CitationHwang et al. 2000; CitationHwang & Kim 2004; CitationKim et al. 2006) or plasma CVD (CitationGirshick et al. 1996; CitationHaaland et al. 1996; CitationBleecker et al. 2004). The temperature of the reactor is so low that the formation of ions would be negligible. The other possible mechanism is that nucleation takes place first and then the nuclei undergo surface ionization on any surface such as the quartz tube of the reactor (CitationHwang & Kim 2004). CitationMagnusson et al. (1999) reported similar unusual charging of gold nanoparticles during reshaping at high temperature. However, further theoretical and experimental studies are needed to understand the charging mechanism in the syntheses of ZnO nanowires.
It should be noted that the actual number concentrations in the reactor are higher than the number concentrations shown in and , considering the loss of charged nanoparticles on the way up to the particle detection point. Considering the surface area of such numerous charged nanoparticles formed in the gas phase, most supersaturation for precipitation of ZnO would be consumed on the formation of charged nanoparticles and the remaining supersaturation on the substrate would be given by the Gibbs-Thompson effect of charged nanoparticles. This means that the flux for the formation of nanostructure on the substrate is mainly from the charged nanoparticles formed in the gas phase.
Charged ZnO nanoparticles should land preferentially on the conducting gold rather than on the insulating natural oxide layer on the silicon substrate. This preferential landing on a conducting area might be related to the selective deposition in CVD widely used in the fabrication of microelectronics (CitationGinsberg et al. 1990; CitationGao & Edgar 1997). Even after ZnO nanowires start to grow mediated by gold nanoparticles, the charged nanoparticles would land preferentially at the tip, where the gold nanoparticle is placed, over the side of ZnO nanowires. Such selective landing seems to result in extensive one-dimensional growth of nanowires (CitationKrinke et al. 2002; CitationBayer et al. 2007). Without such selective landing arising from electrostatic energy, ZnO nanoparticles would land on the side of the nanowires, making the side very rough.
It is not clear whether the growth of nanosheets shown in and is mediated by gold or not. CitationBarnard et al. (2006) reported the relationship between size, structure and orientation in ZnO nanobelts. They suggested that the growth in the width of nanostructures is a decisive factor in determining the orientation. It indicates that the processing conditions are important in the formation of the initial nanostructure morphology. The two-dimensional growth of nanosheets is also expected to come from the electrostatic energy originated from the charge carried by ZnO nanoparticles. Besides, the electrostatic energy previously suggested to explain the growth mechanism of the self-coiling process of ZnO nanorings and nanobelts (CitationWang 2004; CitationKong et al. 2004) and silicon nanowires (CitationHwang et al. 2000; CitationHwang & Kim 2004) should come from the charge carried by nanoparticles such as those shown in and . And the charged nanoparticles seem to be also involved in the one-dimensional growth of oxides such as Nb2O5 (CitationCvelbar and Mozetič 2007) and V2O5 (Mozetič et al. 2005). CitationRutkevych et al. (2007) reported further details of this phenomenon.
CONCLUSIONS
The generation of charged nanoparticles was experimentally confirmed under the typical growth condition of ZnO nanowires. No nanowires were produced in the absence of these charged nanoparticles in the gas phase. The size distributions of charged nanoparticles are affected by reactor temperature and oxygen flow rate. The results suggest that the electrostatic energy originated from charged nanoparticles would be an important factor for the growth of ZnO nanowires.
This work was supported by the Korea Science and Engineering Foundation (KOSEF) through the National Research Lab. Program funded by the Ministry of Science and Technology (No. M10600000159-06J0000-15910) and the Nano-Systems Institute-National Core Research Center (NSI-NCRC) program of the KOSEF.
REFERENCES
- Ahn , H. S. , Park , H. M. , Kim , D. Y. and Hwang , N. M. 2002 . Observation of Carbon Clusters of a Few Nanometers in the Oxyacetylene Diamond CVD Process . J. Cryst. Growth , 234 : 399 – 403 .
- Barnard , A. S. , Xiao , Y. and Cai , Z. 2006 . Modelling the Shape and Orientation of ZnO Nanobelts . Chem. Phys. Lett. , 419 : 313 – 316 .
- Bayer , K. , Dick , K. A. , Krinke , T. J. and Deppert , K. 2007 . Targeted Deposition of Au Aerosol Nanoparticles on Vertical Nanowires for the Creation of Nanotrees . J. Nanoparticle Res. , 9 : 1211 – 1216 .
- Bleecker , K. D. , Bogaerts , A. , Gijbels , R. and Goedheer , W. 2004 . Numerical Investigation of Particle Formation Mechanisms in Silane Discharges . Phys. Rev. E , 69 : 056409
- Cheong , W. S. , Hwang , N. M. and Yoon , D. Y. 1999 . Observation of Nanometer Silicon Clusters in the Hot-Filament CVD Process . J. Cryst. Growth , 204 : 52 – 61 .
- Cvelbar , U. and Mozetič , M. 2007 . Behaviour of Oxygen Atoms near the Surface of Nanostructured Nb2O5 . J. Phys. D: Appl. Phys. , 40 : 2300 – 2303 .
- Fu , H. , Li , H. , Jie , W. and Zhang , C. 2007 . The O2-dependent Growth of ZnO Nanowires and Their Photoluminescence Properties . Ceram. Inter. , 33 : 1119 – 1126 .
- Gao , Y. and Edgar , J. H. 1997 . Selective Epitaxial Growth of SiC . J. Electrochem. Soc. , 144 : 1875 – 1880 .
- Girshick , S. L. , Rao , N. P. and Kelkar , M. 1996 . Model for Ion-induced Nucleation Based on Properties of Small Ionic Clusters . J. Vac. Sci. Technol. A , 14 : 529 – 534 .
- Ginsberg , B. J. , Burghartz , J. , Bronner , G. B. and Mader , S. R. 1990 . Selective Epitaxial Growth of Silicon and Some Potential Applications . IBM J. Res. Dev. , : 816 – 827 .
- Haaland , P. , Garscadden , A. and Ganguly , B. 1996 . Ionic and Neutral Growth of Dust in Plasmas . Appl. Phys. Lett. , 69 : 904 – 906 .
- Huh , J. M. , Yoon , D. Y. , Kim , D. Y. and Hwang , N. M. 2005 . Effect of Substrate Materials in the Low-Pressure Synthesis of Diamond: Approach by Theory of Charged Clusters . Z. Metallk. , 3 : 225 – 232 .
- Hwang , N. M. , Hahn , J. H. and Yoon , D. Y. 1996 . Charged Cluster Model in the Low Pressure Synthesis of Diamond . J. Cryst. Growth , 162 : 55 – 68 .
- Hwang , N. M. , Cheong , W. S. , Yoon , D. Y. and Kim , D. Y. 2000 . Growth of Silicon Nanowires by Chemical Vapor Deposition: Approach by Charged Cluster Model . J. Cryst. Growth , 218 : 33 – 39 .
- Hwang , N. M. and Kim , D. Y. 2004 . Charged Clusters in Thin Film Growth . Int. Mater. Rev. , 49 : 171 – 190 .
- Jeon , I. D. , Gueroudji , L. and Hwang , N. M. 1999 . Deposition of Yttria Stabilized Zirconia by the Thermal CVD Process . Kor. J. Ceram. , 5 : 131 – 136 .
- Jeon , I. D. , Park , C. J. , Kim , D. Y. and Hwang , N. M. 2000 . Experimental Confirmation of Charged Carbon Clusters in the Hot Filament Diamond Reactor . J. Cryst. Growth , 213 : 79 – 82 .
- Jeon , I. D. , Park , C. J. , Kim , D. Y. and Hwang , N. M. 2001 . Effect of Methane Concentration on Size of Charged Clusters in the Hot Filament Diamond CVD Process . J. Cryst. Growth , 223 : 6 – 14 .
- Kim , C. S. , Chung , Y. B. , Youn , W. K. and Hwang , N. M. 2007 . Generation of Charged Nanoparticles during Synthesis of Thin Films, Nanowires and Nanotubes by Chemical Vapor Deposition . Abstracts from 16th European Conference on Chemical Vapor Deposition , : 133 – 134 .
- Kim , J. Y. , Kim , D. Y. and Hwang , N. M. 2006 . Spontaneous Generation of Negatively Charged Clusters and Their Deposition as Crystalline Films during Hot-Wire Silicon Chemical Vapor Deposition . Pure Appl. Chem. , 78 : 1715 – 1722 .
- Kong , X. , Sun , X. and Li , Y. 2003 . Synthesis of ZnO Nanobelts by Carbothermal Reduction and Their Photoluminescence Properties . Chem. Lett. , 32 : 546 – 547 .
- Kong , X. Y. , Ding , Y. , Yang , R. and Wang , Z. L. 2004 . Single-crystal Nanorings Formed by Epitaxial Self-coiling of Polar Nanobelts . Science , 303 : 1348 – 1351 .
- Knutson , E. O. and Whitby , K. T. 1975 . Aerosol Classification by Electric Mobility: Apparatus, Theory, and Applications . J. Aerosol Sci. , 6 : 443 – 451 .
- Krinke , T. J. , Deppert , K. , Magnusson , M. H. and Fissan , H. 2002 . Nanostructured Deposition of Nanoparticles from the Gas Phase . Part. Part. Syst. Charact. , 19 : 321 – 326 .
- Liu , X. , Wu , X. , Cao , H. and Chang , R. P. H. 2004 . Growth Mechanism and Properties of ZnO Nanorods Synthesized by Plasma-enhanced Chemical Vapor Deposition . J. Appl. Phys. , 95 : 3141 – 3147 .
- Magnusson , M. H. , Deppert , K. , Malm , J. O. , Bovin , J. O. and Samuelson , L. 1999 . Gold Nanoparticles: Production, Reshaping, and Thermal Charging . J. Nanoparticle Res. , 1 : 243 – 251 .
- Michael , B. P. , Nuth , J. A. , Lilleleht , L. U. , Bussoletti , E. , Colangeli , L. and Palumbo , P. 1999 . A Nucleation Experiment in Low Gravity Conditions: Monitoring and Collection of Mg and Zn Particles . Adv. Space Res. , 24 : 1273 – 1277 .
- Moisala , A. , Nasibulin , A. G. , Shandakov , S. D. , Jiang , H. and Kauppinen , E. I. 2005 . On-line Detection of Single-walled Carbon Nanotube Formation during Aerosol Synthesis Methods . Carbon , 43 : 2066 – 2074 .
- Mozetič , M. , Cvelbar , U. , Sunkara , M. K. and Vaddiraju , S. 2007 . A Method for the Rapid Synthesis of Large Quantities of Metal Oxide Nanowires at Low Temperatures . Adv. Mater. , 17 : 2138 – 2142 .
- Ostrikov , K. 2005 . Colloquium: Reactive Plasmas as a Versatile Nanofabrication Tool . Rev. Mod. Phys. , 77 : 489 – 511 .
- Ostrikov , K. and Murphy , A. B. 2007 . Plasma-aided Nanofabrication: Where is the Cutting Edge? . J. Phys. D: Appl. Phys. , 40 : 2223 – 2241 .
- Parkansky , N. , Shalev , G. , Alterkop , B. , Goldsmith , S. , Boxman , R. L. , Barkay , Z. , Glikman , L. , Wulff , H. and Quaas , M. 2006 . Growth of ZnO nanorods by Air Annealing of ZnO Films with an Applied Electric Field . Surf. Coat. Technol. , 201 : 2844 – 2848 .
- Park , J. H. and Park , J. G. 2005 . Hierarchical Evolution of Arrayed Nanowires, Nanorods, and Nanosheets in ZnO . Appl. Phys. A , 80 : 43 – 46 .
- Park , J. H. and Park , J. G. 2006 . Synthesis of Ultrawide ZnO Nanosheets . Curr. Appl. Phys. , 6 : 1020 – 1023 .
- Rutkevych , P. P. , Ostrikov , K. and Xu , S. 2007 . Two-dimensional Simulation of Nanoparticle Deposition from High-density Plasma on Microstructured Surface . Phys. Plasmas , 14 : 043502
- Song , J. H. , Jo , W. and Hwang , N. M. 2007 . Non-Uniform Deposition in the Early Stage of Hot-Wire Chemical Vapor Deposition . Thin Solid Films , 515 : 7446 – 7450 .
- Wang , J. , Sha , J. , Yang , Q. , Ma , X. , Zhang , H. , Yu , J. and Yang , D. 2005 . Carbon-assisted Synthesis of Aligned ZnO Nanowires . Mater. Lett. , 59 : 2710 – 2714 .
- Wang , Z. L. 2004 . Zinc Oxide Nanostrucrures: Growth, Properties and Applications . J. Phys. Cond. Matter , 16 : R829 – R858 .