Abstract
An Aerosol Focusing-Laser Induced Breakdown Spectroscopy (Aerosol Focusing-LIBS) with a sheath air focusing and an aerodynamic lens focusing was developed to determine elemental composition of fine and ultrafine metal aerosols. Data showed that with a sheath air focusing, the LIBS qualitatively detected various metals (Al, Ca, Cd, Cr, Cu, K, Mg, Na, Ni, Zn) in submicrometer to micrometer aerosols, but that detection of ultrafine particles smaller than 100 nm was not successful due to weak intensity of emitted light. Also, the hitting rate was so low for particles at low number concentration and the single particle detection approach was only valid when aerosol loading is low. Thus, we concentrated aerosols on to a collection substrate by using the aerodynamic lens focusing system, resulting in the strong emission light from the generated plasma even for nanoparticles and the better quantification performance by the LIBS. We found the linear relationship between LIBS signal response and metal mass concentration. For example, as Cu metal concentration increased, peak area of LIBS emission line for Cu increased. The resulting correlation coefficient was 0.94 and the LOD for Cu mass concentration was found to be ∼80 ng/m3, which can be further lowered by extending current collection time (∼5 min). A similar linear relationship was found for Cd and Ni ultrafine metal aerosols. We also successfully detected internally mixed metal aerosols. When particles were collected on a substrate with the aerodynamic lens for 5 min prior to analysis of the deposit it was possible to analyze particles as small as 60 nm.
INTRODUCTION
Fine (<2.5 μm) and ultrafine (<100 nm) particulate matters (PM) in the ambient atmosphere are of current interest due to their effects on the earth's radiation balance (CitationIntergovernmental Panel on Climate Change 2007), cloud formation, visibility impairment, and human health (CitationDockery et al. 1994; CitationOberdörster 2000). Those particles are directly produced by various sources or formed in the ambient atmosphere by gas-to-particle conversion process. Formation and growth of ultrafine and fine particles in the ambient usually occurred in a short time scale. Thus, to find their sources and formation pathways and to better understand their effects on atmospheric environments, climate change, and human health, it is essential to determine physical and chemical properties of particles in real time. Sulfate, nitrate, ammonium, organic carbon, elemental carbon, and metals/minerals have been known to be major chemical components of atmospheric aerosols (CitationMcMurry 2000; CitationMcMurry et al. 2004). Although metals constitute a small fraction of PM mass, the exceeding concentration and/or long exposure of metals could cause severe adverse effects on human health due to their high toxicity level. Especially, ultrafine metal particles which provide high surface area-to-volume ratio may lead to higher toxicity on human heaths (CitationDockery et al. 1994; CitationPeters et al. 1997; CitationOberdörster 2000). Typically, filter-based techniques with subsequent analyses such as Inductively Coupled Plasma-Mass Spectrometry (ICP-MS) and Atomic Absorption Spectroscopy (AAS) have been used to determine metal concentrations of atmospheric aerosols. However, these techniques require a long sampling time (12–24 h) and many sample preparation steps including chemical extraction by acid solutions, and they also suffer from various sampling artifacts caused by filter sampling (CitationMader et al. 2001; CitationPark et al. 2006).
A Laser Induced Breakdown Spectroscopy (LIBS), which has been used for in-situ determination of elemental composition of samples in solid, liquid, and gas phases, can be applied for aerosol particles (CitationHahn 1998; CitationCheng 2000; CitationHahn et al. 2000; CitationHahn et al. 2001; CitationLombaert et al. 2004; CitationHettinger et al. 2006; CitationVors et al. 2006). The LIBS technique uses a laser-induced microplasma to vaporize and dissociate particles. The resulting excited atoms in the plasma volume emit specific atomic emissions that can be used to identify elemental composition of aerosol particles. Since the LIBS technique does not require high vacuum system like aerosol mass spectrometry (CitationSuess et al. 1999), it is less expensive and highly field portable. Also, it can have flexible probe configuration with the use of fiber optics, applicable for various hazardous environments (CitationCheng 2000). Cremers and Radziemski (1985), CitationCarranza and Hahn (2002), and CitationVors and Salmon (2006) showed that the LIBS technique can detect aerosol particles less than 2–10 μm, which is around the upper size limit for complete vaporization within laser-induced plasma. However, the LIBS technique has showed low sensitivity especially for small particles (CitationHettinger et al. 2006) due to their weak emission lights. With the uses of aerosol focusing systems, conditional single particle data analysis, or double pulse laser, the sensitivity has been improved for submicrometer to micrometer particles (CitationCheng 2000; CitationHahn et al. 2000; CitationCarranza et al. 2002). However, the lowest detectable particle size for the LIBS technique has been reported to be 200–400 nm (CitationHettinger et al. 2006). To our best knowledge, no attempt was made to detect ultrafine particles and nanoparticles less than 100 nm using the LIBS technique.
In this study we develop an Aerosol Focusing-LIBS system to determine elemental composition of fine and ultrafine metal aerosol particles. Especially, we challenge to lower the detectable size limit in the LIBS. A sheath air focusing system was used to measure elemental composition of particles from submicrometer to micrometer, while for detection of ultrafine particles less than 100 nm, the aerodynamic lens focusing system (CitationLiu et al. 1995a; CitationLiu et al. 1995b) with a collection substrate was employed in the LIBS system. Relationship between metal concentration and LIBS emission response was determined using various laboratory-generated metal aerosols (Al, Ca, Cd, Cr, Cu, K, Mg, Na, Ni, Zn) in the size range of 60 nm and 3 μm, and we attempt to quantify metal concentration of aerosols.
EXPERIMENTAL
The Aerosol Focusing-LIBS system mainly consists of a pulsed laser, optics, a spectrometer, and a sampling chamber with aerosol focusing systems as shown in . A high power pulsed Nd:YAG laser (650 mJ/pulse, 10 Hz, Continuum Inc., USA) at the wavelength of 1064 nm and a pulse width of 5 ns was used to create a microplasma onto particles. The laser beam was tightly focused in center of the six-way cross sampling chamber using a plano convex lens (focal length = 75 mm). The power density at the focal point is around 109∼ 1010 W/cm2. After each laser shot, the emitted light was collected through a fiber optic cable equipped with a collecting lens (focal length = 150 mm) at the front end of the cable. The fiber optic cable was connected to the Broadband spectrometer (LIBS + 2000, Ocean Optics Inc., USA) which is able to measure the emitted light at the wavelengths of 200–980 nm, simultaneously, with a spectral resolution of 0.1 nm. A delay generator (BNC565, Berkeley Inc., USA) which is coupled to a Q-switched laser trigger signal was used to control the delay time which decides when the spectrometer receives the emitted light after the laser fires. Since the optimum delay time and laser energy giving the maximum emitted light usually depends on elements examined, we determined them for each element tested in this study. Analyses of emission lines were made by commercial softwares (OOILIBS, and Thermo Galactic Grams/AI 7.02), which use the NIST Atomic Spectra database to assign the observed spectrum peak to specific element. Since each element emits lights at many different wavelengths, we selected emission lines with little interference.
FIG. 1 A schematic of the Aerosol-LIBS system with (a) a sheath air focusing, (b) an aerodynamic lens focusing, and (c) an aerodynamic lens focusing with a collection substrate used in this study.
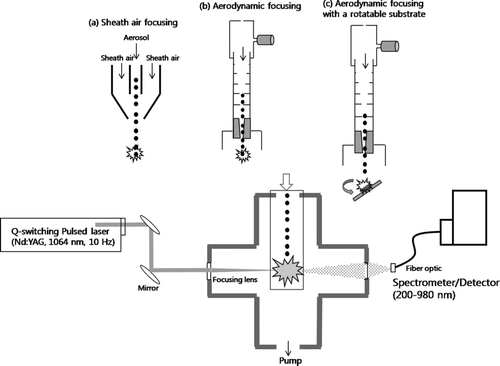
Various metal aerosols with different sizes were generated by an atomizer (Model 3079, TSI Inc., USA) and a dust feeder (MF-2, Sibata Inc., Japan). Metals aerosols tested in this study are summarized in . For internally mixed metal aerosols, we used an ICP-MS standard solution (PerkinElmer, USA). The atomizing system is to aerosolize a metal solution into droplets containing particles. Those droplets are subsequently dried out by using two diffusion driers in series and the remaining metal particles were sent into the LIBS sampling chamber. The dust feeder aerosolizes metal powders on the vibrating substrate and sends the suspended particles into the LIBS sampling chamber. Aerosol particles produced by above methods are simultaneously counted with a respect to particle size using the combination of a differential mobility analyzer (DMA) (Model 3081, TSI Inc., USA) and a condensation particle counter (CPC) (Model 3022A CPC, TSI Inc., USA) in the size range of 20–600 nm, and using the Particle size distribution analyzer (PSD) (Model 3603, TSI Inc., USA) in the size range of 300–10,000 nm. The DMA separates particles according to their electrical mobility size, and then the number of particles of the given size is counted with the CPC in a continuous manner. This provides mobility size distribution of particles in the size range of 20–600 nm with 64 or 128 bins in ∼2 min. By integrating the size distribution, total number or mass concentration of particles in 20–600 nm assuming particle density and shape can be calculated (CitationPark et al. 2003). Also, the DMA was used to generate monodisperse particles to investigate the size-effect of particles on the performance of the LIBS. The PSD measures the particle time-of-flight between two continuous lasers, providing the number of particles having a certain aerodynamic particle size, which is applicable for relatively large particles (>300 nm).
TABLE 1 Metal aerosols tested in this study
Metal aerosols produced and characterized by above methods were sent into the LIBS sampling chamber through three aerosol focusing systems as shown in , , and . A sheath air focusing system () uses a converging nozzle through which an aerosol flow passed. The aerosol flow was surrounded by a sheath flow to maintain the aerosol flow into the centerline. The smallest particle beam diameter which was measured with optical microscopy after collecting them onto filter at the distance of 4 mm is around ∼3 mm. By controlling the nozzle diameter and the ratio of aerosol flow to sheath flow, we are able to tightly focus aerosol particles into the center line, leading to increase the efficiency of particles hit by the laser. Here we used an aerosol flow rate of 3 lpm and a sheath flow rate of 0.3 lpm with a nozzle diameter of 3 mm, which provided the tightest particle beam. Previous research reported that the generated plasma volume was estimated to be ∼2.5*10–4 cm3 with an equivalent sphere of ∼725 μm diameter at the laser energy of 400 mJ/pulse (CitationHahn 1998; CitationHahn et al. 2000).
An aerodynamic lens focusing system as shown in can be used to tightly focus especially ultrafine/nano-particles with much smaller particle beam diameter than the sheath air focusing system. The aerodynamic lens consists of a series of orifices with decreasing size as particles pass through it (CitationLiu et al. 1995a; CitationLiu et al. 1995b). We designed the aerodynamic lens system based on “Aerodynamic lens calculator” to focus particles in the size range of 50–500 nm (CitationWang et al. 2006). In our aerodynamic lens system, the X-Y control stage was used to more accurately control particle beam (CitationPark et al. 2005). The particle beam diameter of aerosol particles (80–200 nm) after focused by the aerodynamic lens was less than 1 mm, which was measured with scanning electron microscopy after collecting them onto filter at the distance of 50 mm. When the aerodynamic lens system was used, the pressure inside the sampling chamber was maintained at 1–2 Torr to focus particles in the size range of 50–500 nm. For the third focusing configuration, we place a rotatable substrate at the 50 mm distance from the end of the aerodynamic lens and collect particles onto the substrate, which is made by quartz, glass, or ceramic as shown in . This leads to concentrate samples within a narrow spot. The particle beam spot on the substrate was adjusted using the X-Y stage in the aerodynamic lens. The substrate was placed on the sample holder designed for the accurate controls of rotational and linear motions. After collection of particles for a certain time (seconds to minutes), the sampling holder was rotated and then the laser beam was focused onto the collected samples to create a microplasma. The substrate was rotated by a known angle for the particle beam spot to be matched with the laser beam spot. This was pre-determined by repeating measurements to find the exact location of particle beam spot with the fixed laser beam spot (the laser focal point was ∼1 mm above the sampling substrate) in the exactly same experimental conditions. This enabled us not to adjust the focus again during main experiments. The laser energy used with this configuration was relatively small (e.g., ∼50 mJ/pulse), which still provided a strong LIBS signal intensity. Also, by controlling the linear position of the sample holder, we can provide a clean substrate for sampling particles. The laser spot size was about 100 um and the particle beam diameter was 300–1000 um at the distance of 50 mm from the end of aerodynamic lens. The LIBS response for the blank substrate was measured to exclude any effects of substrate on the determination of elemental composition of the sample. This configuration is useful especially for ultrafine and nanoparticles that emitted weak light in the plasma volume due to their small mass.
RESULTS AND DISCUSSION
LIBS with a Sheath Air Focusing System
We determined elemental compositions of various metal aerosols generated by the atomizer and dust feeder by using the LIBS with a sheath air focusing system. Size distributions of such metal aerosols were simultaneously measured with the DMA and CPC or PSD. Geometric mean diameter (GMD), laser energy, gate delay time, and wavelength lengths of emission lines for tested aerosols were summarized in . The GMD for metal aerosols tested ranged from 90 nm to 2.7 μm. showed the resulting LIBS spectra of metal aerosols (Al, Ca, Cd, Cr, Cu, K, Mg, Na, Ni, and Zn) (100 shot average). Distinct emissions with different wavelengths were clearly identified for different types of metal aerosols in the LIBS spectra. Laser energy, collecting lens distance, and gate delay time were optimized for each type of metal, which provided the strong emission lines with low background noises (i.e., highest peak-to-base ratio). Peak area in the LIBS spectrum was used to quantify the amount of each element, which will be discussed later. Our data showed that the LIBS with a sheath air focusing system is able to qualitatively detect various metal aerosols in submicrometer to micrometer size.
FIG. 2 LIBS spectra for metal aerosols (Al, Ca, Cd, Cr, Cu, K, Mg, Na, Ni, and Zn) measured with the Aerosol-LIBS with a sheath air focusing system (100 shot average).
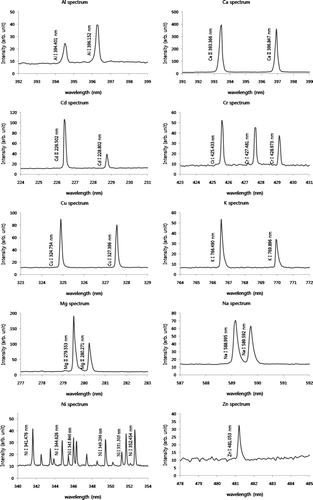
Existence of a single particle at the laser focal point (i.e., in the generated plasma volume) makes it easy for quantification of metal concentration. It was reported that the single particle detection was only valid when the aerosol number concentration was low (CitationCheng 2000; CitationHahn et al. 2000; CitationCheng 2003). To investigate whether our current LIBS detection with a sheath air focusing is based on detection of single particle or multiple particles in the generated plasma volume, we tested CaCl2 aerosols with varying number concentration at a constant mobility size using the DMA (i.e., 200 nm monodisperse CaCl2 aerosols). This will eliminate the effect of particle size on the LIBS response. Effect of doubly charged particles on the LIBS signal response would be small because number concentration of CaCl2 particles above 200 nm significantly decreased. The LIBS signal response (i.e., hereafter we consider it as peak area of the identified peak) and hitting rate (i.e., the fraction of laser pulse that hit a particle for the given laser shot) for 200 nm CaCl2 particles as a function of particle number concentration are measured as shown in . When peak area of Ca emission line at the wavelength of 393.366 nm in the LIBS spectrum was examined for particles having a number concentration lower than ∼80,000 #/cm3, the LIBS signal was constant independent of number concentration, while above ∼80,000 #/cm3, the LIBS signal increased with increasing number concentration as shown in . This suggests that under high aerosol loading condition (i.e., >80,000 #/cm3), multiple particles exist in the plasma volume, resulting in the increased LIBS emission signals. showed the hitting rate for 200 nm CaCl2 particles as a function of particle number concentration. Note that the hitting rate should increase with increasing number concentration when the single particle detection is valid. We found that the hitting rate increased with increasing number concentration below the number concentration of ∼80,000 #/cm3, while above ∼80,000 #/cm3, the hitting rate was close to one as shown in . The steadily increasing hitting rate regime also exists, suggesting that there is a transition regime for detection of multiple particles and single particle. Both and demonstrate that depending on particle number concentration different detection modes (single particle detection vs. multiple particle detection) exist in the LIBS with a sheath air focusing system. The shear air focusing system provided a particle beam diameter of ∼3 mm. With the smaller particle beam diameter, probability for the existence of multiple particles in the laser focal volume can be reduced.
FIG. 3 (a) LIBS signal response (peak area) and (b) hitting rate for 200 nm CaCl2 particles as a function of particle number concentration (1000 shot average).
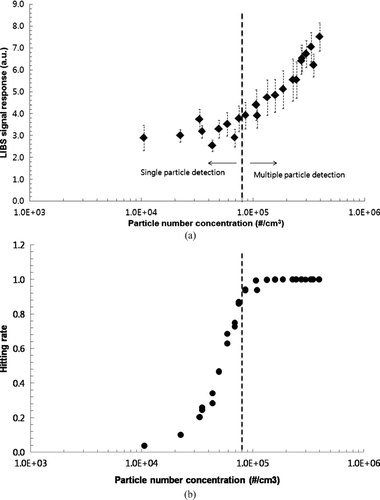
Although the LIBS with the sheath air focusing was able to qualitatively detect metals in submicrometer to micrometer aerosols, detection of ultrafine particles smaller than 100 nm was not successful with the sheath air focusing system. This occurred because the intensity of emission light from ultrafine particles was too weak to detect. Also, the hitting rate was very low for particles having a low number concentration. Lithgow and Buckley (2005) showed that in the single particle detection approach, the variation in particle location relative to the focal volume of the collecting optics had a strong influence on the LIBS signal due to not uniform emission in the plasma volume (CitationLithgow et al. 2005a; CitationLithgow et al. 2005b). Particles located at the edge of plasma, which may be not fully vaporized, or multiple particles present in the plasma, may increase uncertainty in the quantification of element by the LIBS. This suggests that more accurate control of particle beam diameter is required to obtain better quantification performance by the LIBS.
LIBS with an Aerodynamic Focusing Lens System
An aerodynamic lens focusing system as shown in (b) can be used to tightly focus especially ultrafine/nano particles with much smaller particle beam diameter compared to the sheath air focusing system. The low pressure in the chamber (1–2 Torr) with the operation of the aerodynamic lens led to reduce background noises in the LIBS signal (CitationCremers et al. 2007). However, with only aerodynamic lens configuration, we hardly detected nanoparticles (i.e., the hitting efficiency was very low (1–2 hits per 1000 shots) under current free firing laser condition (2–4 Hz laser repetition rate). A laser triggering system that has been often used in single particle mass spectrometry can be employed here to increase the hitting efficiency (CitationJohnston 2000; CitationNoble et al. 2000; CitationNash et al. 2006). However, even if the particle was hit by the laser, the emitted light from nanoparticles was too weak to give valid LIBS signals. Thus, in this study we place a collection substrate at the end of the aerodynamic lens with a distance of 50 mm to concentrate ultrafine/nanoparticles for seconds to minutes as shown in , and then laser was fired on to collected samples to determine elemental composition of those particles. Typically we fired several laser shots onto the sample above the collection substrate (i.e., the laser was focused at the point above the substrate). For our LIBS analysis, we used the spectra obtained from first several laser shots before any elements from blank substrate were detected. Since the sampling time ranges from seconds to minutes, we are able to still maintain a high time resolution. Also, since the aerodynamic lens focusing system can focus a wide dynamic size range of particles, the current system can be applicable for submicrometer to micrometer particles by adjusting the focusing size range. This is different from the previous method that detected polydisperse metal aerosols sampled on quartz filter filters (CitationPanne et al. 2001). The laser focused onto the surface of filter, creating a crater (∼220 um) on the filter surface. In this method, particles penetrated into the inside filter and inhomogeneous distribution of particles may exist. For detection of elemental composition of size-resolved ultrafine and nanoparticles, it will require a long sampling time to obtain valid LIBS signals using the filter method without concentrating such small particles into the narrow spot.
showed the SEM image for CuCl2 particles collected on the collection substrate by using the aerodynamic focusing lens system. The hole in the might be formed by particles taken off during the SEM measurements or preparation steps. After collection, the substrate was rotated and hit by the laser, leading to generate microplasma onto the sample. After the laser hit the sample, the SEM image of remaining spot was also shown in . The size of particle deposit cone depends on the collection time and mass concentration of particles. With constant laser energy, focal size, and a sampling time, the LIBS will ablate the fixed amount of samples, and the intensity of the emitted lights from plasma will be proportional to mass concentration of elements in the sample. Depending on particle concentration, the sampling time was determined to provide a constant amount of samples in the laser focal point. This was done by repeated LIBS measurements with different sampling times at a constant concentration.
FIG. 4 (a) SEM image for CuCl2 nanoparticles collected on the substrate before laser shot and (b) SEM image for the same spot after laser shot.
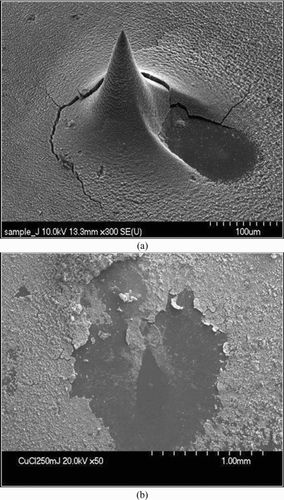
shows the LIBS spectra for 100 nm CuCl2 particles with varying Cu mass concentrations. To obtain desired Cu mass concentration, we diluted the generated CuCl2 aerosols with a clean filtered air. The Cu mass concentration was calculated from the measured particle size and number assuming spherical particles. As shown in , we observed that as Cu mass concentration increased, the Cu emission lines at wavelengths of 324.754 nm and 327.396 nm in the LIBS spectrum increased. The collection time was 5 min for all data. Using data, the relationship between LIBS signal response (peak area) and Cu mass concentration was determined as shown in . The resulting correlation coefficient (r) was ∼0.94 with a linear regression slope of 0.011. The limit-of-detection (LOD) for Cu mass concentration was 80 ng/m3 at current sampling time (5 min). It is possible to achieve the lower the LOD by increasing the collection time (i.e., we can collect more samples under low aerosol loading condition). This relationship can be used to determine Cu mass concentration from the given LIBS signal response. We also tested other metals (Cd, Ni) for determination of the relationship between the LIBS signal response and the amount of metal mass as shown in . In this case, we adjusted the collection time to obtain different masses of metals. Peak areas of emission lines at the wavelength of 226.502 nm and 221.648 nm in the LIBS spectra were used for quantification of Cd and Ni, respectively. We also found a linear correlation (r = 0.96–0.98) between the LIBS signal response and the amount of metal mass. Different regression lines suggested that the calibration lines should be determined for each element.
FIG. 5 LIBS spectra for 100 nm CuCl2 particles with varying Cu mass concentration measured with the Aerosol-LIBS with an aerodynamic focusing lens.
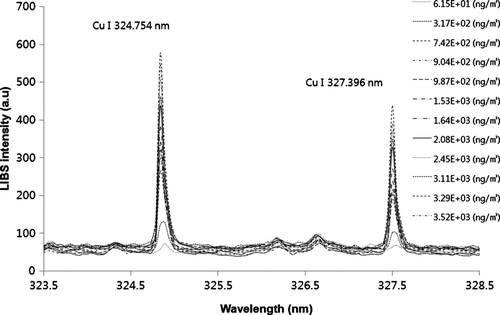
FIG. 7 Relationship between LIBS signal response (peak area) and Cd or Ni mass (228.502 nm emission line for Cd and 221.648 nm emission line for Ni in LIBS spectra).
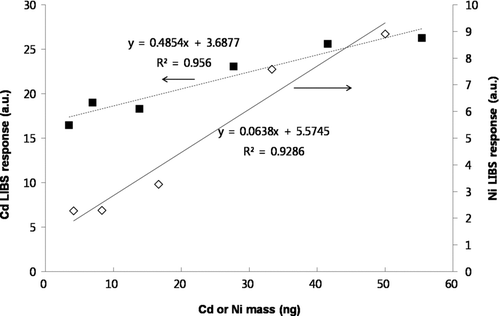
To find the lowest particle size that can be detected by the LIBS, we tested CuCl2 nanoparticles less than 100 nm. The monodisperse 60 and 80 nm CuCl2 nanoparticles generated by the DMA were collected on to the substrate through the aerodynamic lens and subsequently analyzed. The results for 60 nm and 80 nm nanoparticles are shown in and , respectively. Also, the relationship between the LIBS response and metal mass was shown in . We conclude that when particles were collected on a substrate using the aerodynamic lens system prior to the analysis of the deposit, we were able to detect nanoparticles as small as 60 nm. With a conventional impactor method or a sheath air focusing method, particles as small as 60 nm cannot be focused and collected onto a substrate in such a short time. Only with the aerodynamic lens focusing, it is possible to concentrate such small nanoparticles to be detected by the LIBS in a short time (i.e., to provide sufficient mass in the narrow laser spot position). By employing an aerodynamic lens system that is able to focus nanoparticles smaller than the current limit, the detectable size can be lowered. Also, by extending the upper size limit of the aerodynamic lens system, the LIBS can be used to detect coarse particles.
FIG. 8 LIBS spectra for (a) 60 nm and (b) 80 nm CuCl2 nanoparticles, and (c) relationship between LIBS signal response (peak area) and Cu mass.
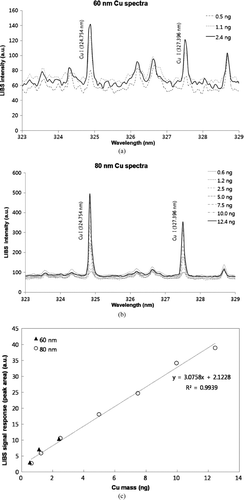
We tested internally mixed metal aerosols containing As, Be, Ca, Cd, Cr, Fe, Cu, Li, Mg, Mn, Mo, Ni, Pb, Sb, Se, Sr, Ti, Tl, V, and Zn atomized from ICP-MS standard solution. The LIBS successfully detected Be, Ca, Cd, Cr, Cu, Li, Mg, Mn, Sb, Sr, Ti, Tl, and Zn simultaneously as shown in . We also observed that the LIBS response increased with increasing the collection time, which is proportional to mass of each metal. The sensitivity of LIBS responses to different masses varied with elements (i.e., different regression slopes between LIBS response and metal mass). As discussed earlier, the optimum delay time and laser energy giving the maximum peak-to-base ratio were determined for each type of element. For the mixed aerosols here, we used the optimum laser energy (100 mJ) and gate delay time (1.5 us) based on Ca emission line. Different values of laser energy and gate delay time will provide not only different LIBS signal intensity, but also different sensitivity to varying metal mass. Thus, for application of the LIBS for determination of metals in atmospheric aerosols, which are typically internally-mixed, optimum laser energy and gate delay time should be determined first. It was demonstrated that mixed elements in particles can bring about changes in local plasma conditions such as plasma temperature, electron density and ionization fraction, affecting the matrix-dependent LIBS response (CitationDiwakar et al. 2007). More extensive research on matrix effect by different elements in the mixed particles will be examined for future work.
CONCLUSIONS
The LIBS with a sheath air focusing system qualitatively detected various metals (Al, Ca, Cd, Cr, Cu, K, Mg, Na, Ni, and Zn) in submirometer to micrometer aerosols. However, it was limited for detection of metals in ultrafine and nanoparticles below 100 nm due to weak intensity of emitted light, and it was difficult to quantify metal concentration due to existence of multiple particles in the plasma volume under high aerosol loading condition as well as low hitting rate under low aerosol loading condition. Thus, the LIBS with an aerodynamic lens focusing system having a collection substrate was developed to concentrate the sample on the laser focal point, resulting in strong emission lines and providing more quantitative relationship between LIBS signal and metal content. Data showed that peak areas of Cu, Cd, and Ni emission lines in the LIBS spectrum linearly increased with increasing their mass. Also, we successfully detected internally-mixed metal aerosols (Be, Ca, Cd, Cr, Cu, Li, Mg, Mn, Sb, Sr, Ti, Tl, and Zn) simultaneously. We found that the lowest particle size that can be detected by the current Aerosol Focusing-LIBS was ∼60 nm, when particles were collected on a substrate for 5 min through the aerodynamic lens prior to analysis of the deposits.
Acknowledgments
The research described in this article was supported by Korea Science and Engineering Foundation (KOSEF) (No. R01-2007-000-10391-0) and partially supported by Korea Research Foundation Grant (KRF-2007-331-D00222).
REFERENCES
- Carranza , J. E. and Hahn , D. W. 2002 . Sampling Statistics and Considerations for Single-Shot Analysis Using Laser-Induced Breakdown Spectroscopy . Spectroc. Acta. Pt. B-Atom. Spectr. , 57 ( 4 ) : 779 – 790 .
- Cheng , M. D. 2000 . Real-Time Measurement of Trace Metals on Fine Particles by Laser-Induced Plasma Techniques . Fuel. Process. Technol. , 65–66 : 219 – 229 .
- Cheng , M. D. 2003 . Field Measurement Comparison of Aerosol Metals Using Aerosol Beam Focused Laser-Induced Plasma Spectrometer and Reference Methods . Talanta , 61 ( 2 ) : 127 – 137 .
- Cremers , D. A. and Radziemski , L. J. 2007 . Handbook of Laser-Induced Breakdown Spectroscopy , New York : John Wiley & Sons .
- Diwakar , P. K. , Jackson , P. B. and Hahn , D. W. 2007 . The Effect of Multi-Component Aerosol Particles on Quantitative Laser-Induced Breakdown Spectroscopy: Consideration of Localized Matrix Effects . Spectroc. Acta. Pt. B-Atom. Spectr. , 62 ( 12 ) : 1466 – 1474 .
- Dockery , D. W. and Pope , C. A. 1994 . Acute Respiratory Effects of Particulate Air Pollution . Ann. Rev. Publ. Health , 15 : 107 – 132 .
- Hahn , D. W. 1998 . Laser-Induced Breakdown Spectroscopy for Sizing and Elemental Analysis of Discrete Aerosol Particles . Appl. Phys. Lett. , 72 ( 23 ) : 2960 – 2962 .
- Hahn , D. W. , Carranza , J. E. , Arsenault , G. R. , Johnsen , H. A. and Hencken , K. R. 2001 . Aerosol Generation System for Development and Calibration of Laser-Induced Breakdown Spectroscopy Instrumentation . Rev. Sci. Instrum. , 72 : 3706 – 3713 .
- Hahn , D. W. and Lunden , M. M. 2000 . Detection and Analysis of Aerosol Particles by Laser-Induced Breakdown Spectroscopy . Aerosol Sci. Tech. , 33 ( 1–2 ) : 30 – 48 .
- Hettinger , B. , Hohreiter , V. , Swingle , M. and Hahn , D. W. 2006 . Laser-Induced Breakdown Spectroscopy for Ambient Air Particulate Monitoring: Correlation of Total and Speciated Aerosol Particle Counts . Appl. Spectrosc. , 60 ( 3 ) : 237 – 245 .
- Intergovernmental Panel on Climate Change . 2007 . “ Climate Change 2007 ” . New York : Cambridge Univ. Press .
- Johnston , M. V. 2000 . Sampling and Analysis of Individual Particles by Aerosol Mass Spectrometry . J. Mass Spectrom. , 35 : 585 – 595 .
- Lithgow , G. A. and Buckeley , S. G. 2005a . Effects of Focal Volume and Spatial Inhomogeneity on Uncertainty in Single-Aerosol Laser-Induced Breakdown Spectroscopy Measurements . Appl. Phys. Lett. , 87 ( 1 ) : 11501 – 11501 .
- Lithgow , G. A. and Buckley , S. G. 2005b . Influence of Particle Location Within Plasma and Focal Volume on Precision of Single-Particle Laser-Induced Breakdown Spectroscopy Measurements . Spectroc. Acta. Pt. B—Atom. Spectr. , 60 ( 7–8 ) : 1060 – 1069 .
- Liu , P. , Ziemann , P. J. , Kittelson , D. B. and McMurry , P. H. 1995a . Generating Particle Beams of Controlled Dimensions and Divergence: I. Theory of Particle Motion in Aerodynamic Lenses and Nozzle Expansions . Aerosol Sci. Technol. , 22 : 293 – 313 .
- Liu , P. , Ziemann , P. J. , Kittelson , D. B. and McMurry , P. H. 1995b . Generating Particle Beams of Controlled Dimensions and Divergence: II. Experimental Evaluation of Particle Motion in Aerodynamic Lenses and Nozzle Expansions . Aerosol Sci. Technol. , 22 : 314 – 324 .
- Lombaert , K. , Morel , S. , Le Moyne , L. , Adam , P. , De Maleissye , J. T. and Amouroux , J. 2004 . Nondestructive Analysis of Metallic Elements in Diesel Soot Collected on Filter: Benefits of Laser Induced Breakdown Spectroscopy . Plasma Chem. Plasma Process. , 24 ( 1 ) : 41 – 56 .
- Mader , B. T. and Pankow , J. F. 2001 . Gas/solid Partitioning of Semivolatile Organic Compounds (SOCs) to Air Filters. An Analysis of Gas Adsorption Artifacts in Measurements of Atmospheric SOCs and Organic Carbon (OC) When Using Teflon Membrane Filters and Quartz Fiber Filters . Environ. Sci. Technol. , 35 : 3422 – 3432 .
- McMurry , P. H. 2000 . A Review of Atmospheric Aerosol Measurements . Atmos. Environ. , 34 ( 12–14 ) : 1959 – 1999 .
- McMurry , P. H. , Shepherd , M. and Vickery , J. 2004 . Particulate Matter Science for Policy Makers: A NARSTO Assessment , New York : Cambridge University Press .
- Nash , D. G. , Baer , T. and Johnstonm , M. V. 2006 . Aerosol Mass Spectrometry: An Introductory Review . Int. J. Mass. Spectrom. , 258 ( 1–3 ) : 2 – 12 .
- Noble , C. A. and Prather , K. A. 2000 . Real-Time Single Particle Mass Spectrometry: A Historical Review of a Quarter Century of the Chemical Analysis of Aerosols . Mass Spectrom. Rev. , 198 : 248 – 274 .
- Oberdörster , G. 2000 . Toxicology of Ultrafine Particles: in vivo Studies . Philosophical Transactions of the Royal Society of London Series A—Mathematical Physical and Engineering Sciences. , 358 ( 1775 ) : 2719 – 2740 .
- Panne , U. , Neuhauser , R. E. , Theisen , M. , Fink , H. and Niessner , R. 2001 . Analysis of Heavy Metal Aerosols on Filters by Laser-Induced Plasma Spectroscopy . Spectroc. Acta. Pt. B—Atom. Spectr. , 56 ( 6 ) : 839 – 850 .
- Park , K. , Chow , J. C. , Watson , J. G. , Trimble , D. L. , Doraiswamy , P. , Arnott , W. P. , Stroud , K. R. , Bowers , K. , Bode , R. , Petzold , A. and Hansen , A. D. A. 2006 . Comparison of Continuous and Filter Based Carbon Measurements at the Fresno Supersite . J. Air and Waste Manag. Assoc. , 56 : 474 – 491 .
- Park , K. , Kittelson , D. B. and McMurry , P. H. 2003 . A Closure Study of Aerosol Mass Concentration Measurements: Comparison of Values Obtained with Filters and by Direct Measurements of Mass Distributions . Atmos. Environ. , 37 : 1223 – 1230 .
- Park , K. , Lee , D. , Rai , A. , Mukherjee , D. and Zachariah , M. R. 2005 . Size-Resolved Kinetic Measurements of Aluminum Nanoparticle Oxidation with Single Particle Mass Spectrometry . J. Phys. Chem. B , 109 : 7290 – 7299 .
- Peters , A. , Wichmann , H. E. , Tuch , T. , Heinrich , J. and Heyder , J. 1997 . Respiratory Effects are Associated with the Number of Ultrafine Particles . Am. J. Respir. Crit. Care Med. , 155 : 1376 – 1383 .
- Suess , D. T. and Prather , K. A. 1999 . Mass Spectrometry of Aerosols . Chem. Rev. , 99 : 3007 – 3025 .
- Vors , E. and Salmon , L. 2006 . Laser-Induced Breakdown Spectroscopy (LIBS) for Carbon Single Shot Analysis of Micrometer-Sized Particles . Anal. Bioanal. Chem. , 385 : 281 – 286 .
- Wang , X. L. and McMurry , P. H. 2006 . A Design Tool for Aerodynamic Lens Systems . Aerosol Sci. Tech. , 40 ( 5 ) : 320 – 334 .