Abstract
This article describes SPLAT II, a new aircraft compatible single particle mass spectrometer that provides significantly improved performance when compared with SPLAT, or any other existing single particle mass spectrometer. SPLAT II detects and characterizes 100% of spherical particles and ∼30% of aspherical particles with diameters between 125 nm and 600 nm. It also brings significant increase in temporal resolution, sizing over 500 particles per second, while characterizing the composition of up to 100 of them. The increase in sensitivity to small particles makes it possible, under most conditions, to use a differential mobility analyzer upfront SPLAT II in order to simultaneously measure in addition to individual particle size and composition, a number of other particle attributes, such as density or effective density, dynamic shape factor, fractal dimension, and even hygroscopicity. SPLAT II provides sizing precision on the order of a monolayer, and makes it possible to distinguish between spherical and aspherical particles. SPLAT II uses a two-step, two-laser process to generate ions. The mass spectra of the semivolatile fraction are generated by ionization in the gas phase, reducing fragmentation and yielding highly reproducible mass spectra, while the mass spectra of the refractory fraction are simultaneously generated by ablation. The instrument control board generates size-dependent delays for laser triggers to eliminate a size-dependent hit-rate and mass spectra are recorded with 14-bit resolution. Data analysis is facilitated by SpectraMiner and ClusterSculptor; two visually driven software packages specifically designed to analyze the large datasets that this instrument produces.
INTRODUCTION
Single Particle Mass Spectrometers (SPMS) have been developed and deployed within the past two decades to characterize, in real-time, the size and chemical compositions of individual ambient particles. A number of instrumental designs have been developed and deployed throughout the years in the laboratory and in the field, each bringing about unique features. Deployment of SPMS on aircraft, presents opportunity to measure the temporal and spatial evolutions of the atmospheric particles in air-masses of interest as they are advected, by carefully choosing aircraft routes. It also provides the opportunity to quantify the properties of particles as a function of altitude. However, deployment of SPMS on aircraft represent significant additional challenges to instrument design and performance (CitationThomson et al. 2000). Aircraft instruments must comply with stringent requirements that relate to their structural integrity, safety, power consumption, dimensions, weight, and sensitivity to vibrations, while also having to face the greatly increased demands on instrument performance. For example, to characterize air-masses in urban environments, which commonly originate from multiple and diverse sources, aircraft based instruments must have temporal resolution on the order of 10 seconds (CitationKleinman et al. 2008), which can only be achieved through improvements in instrument detection sensitivity. It is also important to keep in mind that since the vast majority of atmospheric particles are smaller than 150 nm in diameter a proper representation of atmospheric particles requires sufficient sensitivity to small particles.
Deployments of the various SPMS designs in the laboratory and in the field have undeniably proven to be extremely valuable in improving our understanding of the properties and evolution of ambient particles. Detailed descriptions and comparisons between many of these instruments are given in a number of recent reviews and tutorials (CitationHinz and Spengler 2007; CitationJohnston 2000; CitationMcMurry 2000; CitationMurphy 2007; CitationNash et al. 2006; CitationNoble and Prather 2000; CitationSuess and Prather 1999; CitationSullivan and Prather 2005), and will therefore not be presented here. This article focuses on SPLAT II, a new single particle mass spectrometer that is designed to comply with the unique and stringent requirements imposed on aircraft compatible instrumentation. In addition to being aircraft compatible, SPLAT II offers higher sampling rates and hence improved temporal resolution, significantly improved detection sensitivity to small particles, and extremely precise particle sizing. SPLAT II uses a two-laser, two-step evaporation ionization to generate reproducible single particle mass spectra with reduced fragmentation (CitationZelenyuk et al. 2009a), has mass spectral dynamic range that is 128 times higher than its predecessor, SPLAT (CitationZelenyuk and Imre 2005), and deploys a novel laser triggering approach that yields size-independent hit-rate (CitationZelenyuk et al. 2009b).
The vast majority of field deployable SPMS rely on light scattering for particle detection, particle sizing and for triggering an ablation laser to generate ions for mass spectrometric analysis. Since the intensity of the light scattered by particles decreases extremely rapidly with particle size (CitationCross et al. 2007), the detection efficiencies of particles that are smaller than ∼150 nm in diameter tend to be very low (CitationSu et al. 2004; CitationZelenyuk and Imre 2005). Some SPMS overcome their inability to detect small particles by randomly firing the ablation laser. Unfortunately, this approach yields a hit-rate that is lower than 0.01% (CitationMurphy 2007) and provides no information about particle size (CitationErdmann et al. 2005; CitationReents et al. 1995) unless particles are size-classified prior to sampling (CitationRhoads et al. 2003), which further decreases instrument sampling rate and sensitivity. SPLAT II offers significantly improved optical detection and characterization sensitivity of small particles: It detects and characterizes at least 1 particle per second, if the concentration of spherical particles that are between 125 nm and 600 nm in diameter are as low as 1 particle per cm3. The sensitivity of SPLAT II to spherical 100 nm particles is a factor of 40 higher than the first generation instrument (CitationZelenyuk and Imre 2005) and 2 orders of magnitude higher than the only other SPMS that is capable of optically detecting and sizing individual particles smaller than 100 nm (CitationSu et al. 2004). In SPLAT II the detection probability of aspherical particles is only a factor of 3 smaller than that of spherical particles of the same size. The significant increase in instrument sensitivity yields high sampling rates that are being taken advantage of by sizing over 500 particles per second and recording up to 100 individual particle mass spectra per second. This improvement represents an increase in temporal resolution by a factor of 10 over SPLAT or any other existing SPMS (CitationAllen et al. 2006).
The capability to detect particles with a wide range of sizes poses a new challenge that, if unmet, results in a size-biased hit-rate, forcing the instrument operator to choose either to characterize small or large particles (CitationSu et al. 2004). We have identified the origin of this effect and developed an instrument control board (ICB) that generates size-dependent delays for laser triggers to eliminate, in SPLAT II, the size-dependent hit-rate (CitationZelenyuk et al. 2009b).
To cover the rich composition spectrum of particles found in many fields requires a complex and flexible ion formation approach. In atmospheric science alone, particle compositions include fragile organic molecules mixed with soot, dust, and inorganic compounds. Most laser-based SPMS rely on ablation to generate ions for mass spectral analysis (CitationGard et al. 1997; CitationRhoads et al. 2003; CitationThomson et al. 2000). Consequently the mass spectra that are generated by this highly non-linear, multi-photon process are strongly influenced by matrix and charge transfer effects (CitationCai et al. 2006; CitationGe et al. 1998; CitationGross et al. 2000; CitationReilly et al. 2000). As a result, single particle mass spectra of many atmospheric particles are dominated by high intensity the ion peaks of K or Na. Ablation also causes extensive fragmentation of organic molecules and significant particle-to-particle fluctuations of peak intensities. Ablation is often the leading cause of irreproducible, and difficult to interpret mass spectra.
Separation between particle evaporation and ion formation was shown to greatly improve the analytical capability of SPMS (CitationBaer et al. 1999; CitationCabalo et al. 2000; CitationMorrical et al. 1998; CitationSykes et al. 2002; CitationWoods et al. 2001; CitationZelenyuk and Imre 2005; CitationZelenyuk et al. 2009a). In SPLAT II the mass spectra are generated by a two-step process, in which an infrared (IR) laser pulse is used to evaporate the semivolatile fraction and, under most circumstances, a time-delayed UV laser is used to ionize the evaporating plume and ablate the remaining nonvolatile fraction. This approach yields mass spectra of the semivolatile and nonvolatile fractions, reduces fragmentation, and improves mass spectral reproducibility (CitationZelenyuk et al. 2009a). Moreover, ionization in the gas phase yields significantly more ions, thereby increasing the quality of the mass spectra of small particles (CitationZelenyuk et al. 2009a). This two-step IR/UV approach also greatly diminishes matrix and charge transfer effects that have hampered the quantitative capabilities of SPMS. Depending on the application, this two-laser approach also offers the flexibility to configure the IR and UV lasers in a manner that yields either mass spectra of both; the nonvolatile and the semivolatile fractions of each particle, or only the semivolatile part (CitationZelenyuk et al. 2009a).
SPLAT II uses a data acquisition system with a 14 bit dynamic range—an increase of a factor of 128 over the previous instrument (CitationZelenyuk and Imre 2005). This allows the instrument to record mass spectra of internally mixed particles containing substances with a wider range of ionization cross sections or abundance.
High instrument sensitivity makes it possible to use a Differential Mobility Analyzer (DMA) or a Tandem DMA (TDMA) to classify and characterize particles with narrow distributions of sizes, while maintaining sampling rates that are sufficiently high to provide statistically robust datasets. We have shown that when SPLAT II or even its predecessor SPLAT are combined with a DMA or TDMA upfront the system can simultaneously measure, in-situ, in real-time individual particle composition, size, and for spherical particles, density (CitationZelenyuk et al. 2005; CitationZelenyuk et al. 2007; CitationZelenyuk et al. 2008c). For aspherical particles the same measurements yield particle effective density that can be used for some particle systems to calculate the particle dynamic shape factors (CitationZelenyuk et al. 2006a; CitationZelenyuk et al. 2008d). The combined system also provides information on particle asphericity (CitationZelenyuk et al. 2008c), asymmetry (CitationZelenyuk and Imre 2007), and morphology (CitationZelenyuk et al. 2008d). When applied to soot particles the same measurements of particle mobility and vacuum aerodynamic diameters are used to calculate the particles fractal dimension (CitationZelenyuk et al. 2006b). We also showed that when a hydrated tandem DMA is combined with SPLAT or SPLAT II it provides in addition to the list above, particle hygroscopicity (CitationZelenyuk et al. 2007; CitationZelenyuk et al. 2008a).
To take full advantage of the vast amounts of detailed data SPLAT II generates calls for unconventional approaches. To accomplish this task we have developed two dedicated software packages called ClusterSculptor (CitationNam et al. 2007; CitationZelenyuk et al. 2008b) and SpectraMiner (CitationZelenyuk et al. 2006c). The former offers a wide variety of intuitive, interactive, and visually driven tools that are combined with scientific knowledge to steer the data classification process, while the latter is a data visualization and mining program that adopts a very different approach from any other in the field (CitationHinz et al. 1999; CitationMurphy et al. 2003; CitationPhares et al. 2001; CitationRebotier and Prather 2007; CitationSong et al. 1999; CitationTrimborn et al. 2000). SpectraMiner makes it possible to mine datasets of millions of particles without loss of information and explore relationships between different particle classes and between particles and any other data that were concurrently collected.
EXPERIMENTAL
Particle Generation and Handling
The majority of laboratory particles presented in this paper were generated with an atomizer (TSI Inc., Model 3076), by aerosolizing the corresponding suspensions, neat compounds, and solutions. To avoid effects of surfactants and agglomeration Polystyrene latex (PSL) beads (Duke Scientific) were generated from water suspensions, whose volume fractions were lower than 10−5. Dioctyl phthalate (DOP) (Sigma-Aldrich, 99% purity) was aerosolized neat; NaCl (Sigma-Aldrich, >99.5% purity), NaNO3 (Fisher Scientific, >99.5% purity), and trans-sobrerol (trans-p-menth-6-ene-2,8-diol, Sigma Aldrich 99% purity) were dissolved in ultrapure deionized water and aerosolized. The aerosol flow was dried by two diffusion dryers (TSI Inc., Model 3062), connected in series and diluted by mixing with particle-free, dry air.
Secondary organic aerosol (SOA) particles were formed by ozonolysis of α-pinene. The reaction was performed in a ∼100 L Teflon bag at low relative humidity (RH < 1%). α-Pinene (Aldrich, 98% purity) was warmed and volatilized before its introduction into the Teflon bag at a concentration of 0.3 ppmv. The initial concentration of O3 was twice as high as that of α-pinene. Particles were formed by homogeneous nucleation and their size distributions were determined using a Scanning Mobility Particle Sizer (SMPS, TSI Inc., Model 3936).
In some experiments presented here a DMA (TSI Inc., Model 3081) was used to classify particles with narrow mobility size distributions prior to their characterization by SPLAT II.
SPLAT II Design Elements
shows a schematic representation of SPLAT II. The instrument is constructed by machining a single aluminum block into a 50.8 × 15.2 × 15.2 cm rectangular box that comprises two differentially pumped sections and the main, high vacuum chamber. A single lid is used to seal all three sections. With the lid removed unobstructed access to all relevant instrument elements becomes available.
The aerodynamic lens inlet is attached to the instrument using an adjustable mount that provides means to align or adjust the particle beam while the instrument is running. The nozzle side of the aerodynamic lens is held in a lockable two-dimensional homemade gimbal mount with fine adjustments. Particles enter into the aerodynamic lens inlet (CitationZelenyuk and Imre 2005) through a 100 μm flow-calibrated critical orifice at a rate of ∼0.1 l/min. The lens forms a particle beam that proceeds into the high vacuum section through two skimmers. The distances between the aerodynamic lens and the first skimmer and the first and second skimmers are 1.27 and 2.54 cm, respectively. Both skimmers are 1 mm in diameter.
The pumping system includes four 250 l/sec turbomolecular pumps (Varian Inc., Model Turbo-V 301), one at each differential pumping section and two others in the main, high vacuum section. Two scroll pumps (Edwards, Model XDS10) are used as backing pumps. This pumping scheme yields a pressure of ∼270 Pa at the entrance to the aerodynamic lens inlet and ∼3·10−4 Pa in the mass spectrometer region.
The aerodynamic lens is used to form a very narrow particle beam, with low divergence and transport the particles into the instrument with extremely high efficiency (CitationJayne et al. 2000; CitationLiu et al. 1995a, Citationb; CitationLiu et al. 2007; CitationZelenyuk and Imre 2005). This inlet imparts particles with very narrow velocity distributions that are related to their vacuum aerodynamic diameters, making it possible to determine their size with high precision (CitationZelenyuk and Imre 2005). Furthermore, we have recently shown that for DMA classified particles there is a robust relationship between particle shape and the width of their vacuum aerodynamic diameter distributions, making it possible to easily distinguish between spherical and aspherical particles (CitationZelenyuk et al. 2008c).
Once the particles pass through the aerodynamic lens inlet and the two skimmers, they enter the main, high vacuum section, where their presence is detected by light scattering in two optical detection stages, marked as ODS1 and ODS2 in .
Two 300 mW, frequency doubled diode-pumped continues wave (CW) Nd:YAG lasers operated at 532 nm (CrystaLaser, Model GCL-300-L) are focused to ∼330 μm spots at the 1st focal point of the elliptical reflectors in each of the two optical detection stages. The elliptical reflectors (Opti-Forms, Model E102-1, F# 0.91) are used to collect more than 60% of the light scattered by each particle and to refocus it at the 2nd focal point located in front of the photomultiplier counting heads (PMT) (Hamamatsu, Model H6180-01). The signals from the PMTs are processed to produce TTL pulses for photon counting to be compared to an operator set discrimination thresholds.
As in any other instrument, the detection limit in SPLAT II is determined by the signal to noise ratio: here the signal is the number of detected photons scattered by a particle and the noise is determined by background, which in this case is dominated by stray laser light. The signal in SPLAT II was increased by a factor of ∼12 over SPLAT by increasing the laser power, removing the 532 nm laser-line filters in front of the PMTs, and precisely positioning the components that comprise the optical detection stages. At the same time the background was decreased by using higher quality windows, blackening the entire instrument, and using a precisely constructed light baffling system.
The particle detection threshold is set by the operator to assure that zero particles are detected when no particles enter the instrument. Note that keeping this threshold at a minimum is critical to achieving high detection sensitivity for small particles. In SPLAT II the particle detection threshold is typically set to 10 photons per two microseconds as compared to 5 photons per 0.5 microseconds in SPLAT.
During instrument operation the signals at both optical detection stages are continuously monitored and recorded. Once the threshold condition is met in ODS1 a clock is turned on, and if the threshold condition is met in ODS2 within a prescribed time interval, the time of detection and the time that elapsed between the two detection events are recorded. This particle time of flight (PTOF) between ODS1 and ODS2 is used to obtain the particle velocity, from which the particle vacuum aerodynamic diameter is calculated. The operator has an option to set the range of acceptable PTOF, thereby selecting the particles size range to be studied.
The particle detection signals are also used to generate triggers for the IR and UV lasers. The distances between ODS1 and ODS2 and ODS2 and the center of the ionization region of the angular reflectron time-of-flight mass spectrometer (TOF-MS, R. M. Jordan, Inc., Model D-850) are 10.5 cm. The laser trigger signals delays are proportional to PTOF, with proportionality coefficients that are close to 1 and set precisely by the operator during instrument alignment to assure that the particle is hit by the evaporation and ionization lasers with high probability.
The enormous difference between the light scattering signals generated by small and large particles leads to a size dependent hit-rate (CitationSu et al. 2004; CitationZelenyuk et al. 2009b). The light scattering signal from large particles reaches threshold well before the particles arrive at the center of the green laser beam, whereas to reach threshold conditions small particles must pass almost through the entire laser beam. These spatial differences between large and small particles amount to a difference of ∼3 μs in the optimum laser timing, and thus greatly impact the probability with which the evaporating and/or ionizing lasers hit particles of different sizes. If this problem is untreated the operator has to choose, during instrument alignment, the laser trigger delay parameters to optimize the hit-rate for particles in the size range of interest, which in turn means having to accept a lower hit-rate for other sizes (CitationSu et al. 2004). In SPLAT II this issue is addressed with a built-in option to set multiple proportionality coefficients that depend on particle size (CitationZelenyuk et al. 2009b). As particles are detected and sized, the instrument selects, on the fly, the appropriate laser trigger delays on the basis of the measured PTOFs. A study describing the phenomenon and the implemented solution is described in detail in a separate publication (CitationZelenyuk et al. 2009b).
The CO2 laser (Edinburgh Instruments, Model MTL-3, 50 ns pulse length) is operated at 10.6 μm, with energy of 110 mJ/pulse and is focused to a spot that is 1 mm in diameter. The UV excimer laser (GAM Lasers, Model EX5/300, 8 ns pulse length) is operated at 193 nm, with energy between 0.5 to 2 mJ/pulse and is focused to a 550 × 750 μm focal spot. The laser beams are typically aligned to assure that each particle first passes through the 1 mm CO2 laser focal spot and that it travels for a few additional microseconds before it crosses the focal spot of the UV laser. The delay time between the two lasers is chosen to provide sufficient time for the semivolatile compounds to evaporate before they are being ionized, in the gas phase. The exact delay depends on the relative position of the two laser beam foci and on the specifics of the experiment. Positioning the IR and UV lasers and choosing the laser-trigger delay coefficients is an integral part of instrument alignment. The operator has the option to choose either a configuration, in which the UV laser pulse precisely hits the particle center-of-mass to yield mass spectral signatures of both, the semivolatile and the nonvolatile particle fractions, or of the semivolatile fraction only. Most often the UV laser trigger pulse is timed to hit the particle center-of-mass to assure that nonvolatile fraction, if present, is ablated and that the semivolatile fraction is ionized in the gas phase. This mode provides for each particle mass spectral signatures of both fractions.
The optimal operation of SPLAT II relies heavily on proper alignment of the aerodynamic lens inlet, the skimmers, the elliptical reflectors, the baffles, the two green lasers, and the IR and UV lasers. This task is accomplished by deploying a two-prong approach: The positions of the light baffles and the two skimmers are determined by high precision machining and alignment pins that assure proper repositioning during the instrument assembly, while the rest of the instrument elements are adjusted by the operator during instrument assembly and/or alignment. Each reflector is mounted in a manner that affords three dimensional and rotational adjustments. The alignment of the particle beam and each of the green lasers are accomplished by positioning them in the adjustable and lockable mounts. The alignment of the IR and UV laser beams is accomplished with two mirrors for each laser beam. Once the alignment procedure is completed all the lens and mirror mounts are locked into their positions.
Because instrument alignment plays a central role in determining its performance, in SPLAT II the operator follows a strict assembly and alignment procedure that was developed to yield consistent results. While the instrument collects data its performance is routinely monitored by examining the particles detection rates in ODS1 and ODS2, the rate with which PTOFs are recorded, and the particle hit-rate. Experience shows that once aligned, SPLAT II operates for many weeks without the need to realign or adjust any of the elements. The ellipsoidal reflectors, for example, were not realigned since the instrument's very first assembly. Similarly, throughout the entire month-long field campaign that included 27 flights and over 100 flight hours the instrument operated continuously and remained perfectly aligned, requiring no adjustments.
Instrument Frames
is a photograph of SPLAT II assembled in the aircraft compatible configuration, which consist of two detachable frames, labeled Frame 1 and Frame 2 in . Both frames are constructed from a heavy duty aluminum framing system (AMCO, IMS Engineered Products LLC). Once on board, the two frames are connected to each other and mounted as a single unit to the aircraft floor followed by a final instrument alignment. This two-frame design makes it possible to install SPLAT II onto smaller aircraft, like the DOE's G-1 that requires a tight turn near the aircraft entrance. Frame 1 contains electronic equipment only and Frame 2 supports a second, internal frame that is marked in blue and labeled I Frame in . The internal frame carries the vacuum chamber, lasers, and optics. It is suspended on a set of vibration dampening mounts to isolate it from the vibrations of the aircraft and the mechanical pumps. The overall instrument dimensions, including the two aircraft compatible frames, are 143.5 × 69.9 × 125.5 cm (W × D × H), it weighs nearly 400 kg, and consumes 3500 W.
Instrument Control Board
The instrument control board (ICB) is used to open gates, examine PMT signals, generate laser triggers, and control data recording. The functions for this board are implemented by a Xilinx Virtex-4 FPGA (field-programmable gate array) device, which contains programmable logic components and an embedded dedicated power PC processor. The ICB control software provides the user with a simple graphical interface through which operational instrument parameters are transferred to the FPGA board.
Before data are acquired the operator defines a number of parameters: lengths of integration windows and thresholds for PMT1 and for PMT2, the PTOF range that is defined by maximum and minimum PTOFs, PTOF dependent laser trigger delay coefficients for the IR and UV lasers, A/D converter settings, maximum lasers triggering rate, etc. The signals generated from the 1st and 2nd PMTs are continuously acquired, processed, and compared by the ICB to the user set thresholds and PTOF range, to discriminate particles from background. A detection event in ODS1 generates a timestamp that is placed in a FIFO (First In, First Out). When a particle is detected in ODS2 the last recorded timestamp is read from the FIFO and compared to the current timestamp, with the difference being a PTOF, which is immediately compared to the user specified PTOF range. If it is found to fall inside the range, the PTOF is recorded with its timestamp. At this point the particle's precise position in the instrument is determined and its velocity is known. This information is used to generate the triggers for the IR and UV lasers and a signal to acquire the particle mass spectrum with the A/D converter board (Gage Applied Technologies, Inc., Model CompuScope 14200). The precise timings of the IR and UV laser pulses are set by the user during instrument alignment by inputting a set of proportionality coefficients for a corresponding set of ranges of particle PTOFs (CitationZelenyuk et al. 2009b).
is a flow chart of the ICB logic, which shows the main modules, where operator settings and signal inputs are being processed to determine the presence of a particle, calculate and record its PTOF, and generate the properly timed trigger pulses for the lasers and the A/D converter board (CompuScope).
During data acquisition a file containing the measured PTOFs, their timestamps, the rates with which particles are detected in ODS1, ODS2, and the coincidence rate are recorded. The ratio of the two particle counting rates in ODS1 and ODS2 provides a measure of particle beam divergence, which depends on particles shape and size (CitationHuffman et al. 2005; CitationSalcedo et al. 2007). When SPLAT II samples spherical particles that are larger than 125 nm in diameter, the ratio of the rate of particle detection in ODS1 and ODS2 is between 1.0 and 1.1. But when the particles under study are aspherical, the particle beam divergence increases and the rate of particle detection in ODS1 is a factor of ∼2 higher than that in ODS2. In other words, the ratio of the rate of particle detection in the two optical detection stages can be used to distinguish between spherical and aspherical particles. A study illustrating this approach on laboratory and field data will be presented in a separate publication.
To account for the fact that the rate of particle detection and sizing often exceed the rate at which the IR and UV lasers can be fired, the file also contains for each particle a marker that signifies whether laser triggers were produced.
The mass spectral data are saved in a 16-bit format, with zeroes padding for two of the least significant bits, since the A/D converter has 14-bit resolution. Each mass spectrum is accompanied by a timestamp, the PTOF, and the A/D voltage scale. When a data folder is closed the parameters that were entered into the user interface are also saved in the same folder. This allows the user to easily find the correct parameters that were associated with each experiment, and to reload those parameters in subsequent experiments.
The section below provides a few examples of measurements conducted with this new instrument in the laboratory that illustrate its capabilities, followed by a brief description of its performance on board the Canadian National Research Council Convair 580 aircraft during Indirect and Semi-Direct Aerosol Campaign (ISDAC).
RESULTS AND DISCUSSION
Calibration and Measurements of Particle Vacuum Aerodynamic Diameter
The velocities and hence PTOFs of particles exiting the aerodynamic lens are directly related to their vacuum aerodynamic diameters. The measured PTOFs are converted to vacuum aerodynamic diameter using a calibration curve that is generated by measuring the PTOFs of particles of known vacuum aerodynamic diameters. Here we illustrate the process using NIST certified size standards, spherical polystyrene latex (PSL) beads of known diameters. is a plot of the measured PTOF distributions of PSL beads with 13 different sizes from 50 nm to 993 nm. shows a calibration curve of the particle diameters vs. their measured PTOFs. A logarithmic scale for both coordinates was used to illustrate that a simple power relationship between the two variables is valid down to 50 nm. Calibration curves can also be generated using particles of known density that are classified by a DMA.
FIG. 4 (a) Distributions of particle time of flights for PSL particles with 13 different sizes from 50 nm to 993 nm; (b) A calibration curve used to convert particle time of flight to vacuum aerodynamic diameter; (c) vacuum aerodynamic diameter distributions of different types of monodisperse particles (as marked).
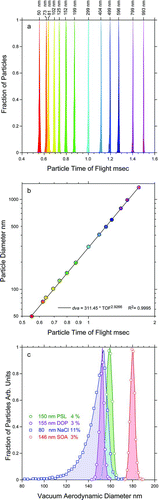
We find that when using PSL beads for instrument calibration one must take extreme care to avoid using suspensions with high concentrations of PSL beads. The tendency to overcome low detection probabilities by increasing suspension concentration yields aerosol that are dominated by agglomerates and/or aspherical PSL particles with surfactants-containing nodules (CitationCai et al. 2006; CitationZelenyuk et al. 2005; CitationZelenyuk et al. 2006a). This is particularly true for the smaller PSL particles, for which the manufacturer supplied suspensions have higher number concentrations and most instruments have lower sensitivities.
provides a few illustrative examples of the line-shapes of vacuum aerodynamic size distributions and the precision with which their positions are measured with SPLAT II. The green line, peaking at 159.5 nm is for 152 nm PSL particles. It shows a line-shape with full width at half maximum (FWHM) of 4% that is consistent with the manufacturer specified size distribution of 3.4% and its peak position yields a density of 1.05 gcm−3, which is also in agreement with the known value. The purple line in shows the measured size distribution of dioctyl phthalate (DOP) particles classified by the DMA at 155 nm. The width of the measured vacuum aerodynamic size distribution of these particles is 3% (FWHM) and the line position of 153 nm yields a density of 0.99 gcm−3, in agreement with the known DOP density of 0.986 gcm−3. The red size distribution represents secondary organic aerosol (SOA) particles formed by the ozonolysis of α-pinene and classified by the DMA at 146 nm. The measured vacuum aerodynamic diameter of these 146 nm particles is 180 nm, yielding a density of 1.23 gcm−3. Their size distribution has a FWHM of 3%, on the basis of the which we conclude that they are spherical (CitationZelenyuk et al. 2008c).
The observed line-shapes of the vacuum aerodynamic size distributions of DMA classified particles can be used to calculate the precision with which particle diameter can be measured with the SPLAT II/DMA system. Since the signal to noise ratio here is significantly higher than 10, the average particle vacuum aerodynamic diameter of a size distribution whose FWHM is 3% can be determined with precision that is better than 0.3%. For a 150 nm particle this translates to 0.45 nm. Since the addition or removal of a monolayer that is ∼0.5 nm thick changes particle diameter by 1 nm, it can easily be measured by the SPLAT II/DMA system. The data in and in another recent publication (CitationZelenyuk et al. 2008c) also demonstrate that the use of the SPLAT II/DMA system yields, for spherical particles, the capability to measure their density with an accuracy of 0.5%.
For aspherical particles simultaneous measurements of particle mobility and vacuum aerodynamic diameters yield particle effective density and can for some systems be used to calculate their dynamic shape factors with high precision (CitationDeCarlo et al. 2004; CitationZelenyuk et al. 2006a). The blue line in represents the measured vacuum aerodynamic size distribution of NaCl particles that were classified with the DMA at 80 nm. This distribution is asymmetric and has FWHM of 11%. The observed line broadening and asymmetric line-shape are consistent with the fact that NaCl particles are aspherical (CitationZelenyuk et al. 2008c). The measured effective density for these particles is 1.9 gcm−3 as compared with the known NaCl bulk material density of 2.165 gcm−3. The mobility and vacuum aerodynamic diameters together with the known material density can be used to calculate a dynamic shape factor of 1.07 for these particles, a value consistent with cubic particles with slightly rounded edges.
The distinct differences between the line-shapes of spherical and aspherical particles with similar mobility size distributions are reflection of the orientation-dependent particle dynamic shape factors and can be used to distinguish in real-time between spherical and aspherical particles (CitationZelenyuk et al. 2008c). As we noted above, the narrow size distribution of the DMA selected SOA particles clearly indicates that they are spherical.
Detection Probabilities as a Function of Particle Size and Shape
This section presents the results of measurements of particle detection probability as function of size for three particle types: spherical PSL beads, DMA selected spherical sodium nitrate, and aspherical sodium chloride. Instrument detection probability is defined as the ratio of particles successfully detected and properly sized to the number of particles entering the instrument, as measured by the condensational particle counter (TSI Inc., Model 3786). Defined in this manner the detection probability depends on the inlet's transmission efficiency, the divergence of the particle beam, and on the optical detection sensitivity. It is therefore directly related to the instrument performance. shows that the detection of spherical particles with diameters between 125 nm and 600 nm is very close to 100%. The detection of particles larger than 600 nm decreases slowly because of the decrease in particles' transmission efficiency through the aerodynamic lens inlet. The detection probability of smaller particles decreases to 40% for 100 nm particles and to 1.5% for 70 nm particles. The rapid decrease in detection efficiency of small particles reflects a decrease in light scattering signal and an increase in particle beam divergence.
FIG. 5 A plot of the detection efficiency of sodium nitrate, PSL, and NaCl particles as a function of particle mobility diameter. The inset is a “zoom in” view of ultrafine particles.
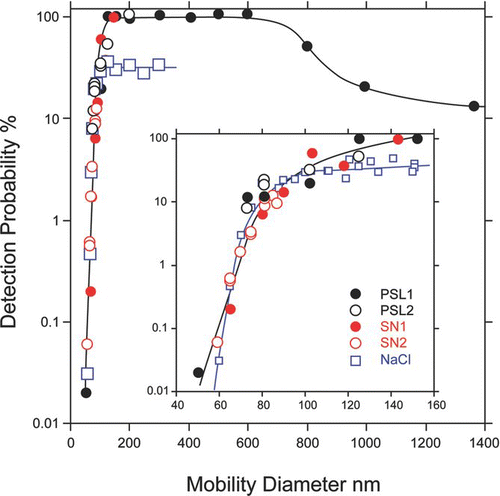
To illustrate the reproducibility of the instrument performance shows, for the two spherical particle types, the results of four separate measurements, each conducted following instrument realignment. The data clearly demonstrate that the instrument detection probabilities as a function of particle size are highly reproducible.
Note also that the detection probabilities of sodium nitrate and PSL particles, as a function of particle size, show that the two particle types form identical trends, indicating that the detection probability is mostly a function of the particle physical diameter and not its vacuum aerodynamic diameter.
Because aspherical particles form more divergent aerosol beams they are harder to detect (CitationHuffman et al. 2005; CitationMurphy 2007; CitationZelenyuk and Imre 2005). To quantify the effect of particle shape on the detection probabilities, NaCl particles with narrow size distributions were generated and their detection probabilities as a function of size were measured. The results of these measurements are also presented in . These data show that for larger particles the detection efficiency of aspherical particles drops from 100% to 30%. But, the detection probabilities of NaCl particles that are smaller than 100 nm are virtually indistinguishable from those of spherical particles. Since particle beam divergence depends on particle shape, the detection probabilities of aspherical particles are expected to somewhat vary depending on particle shape.
As mentioned above, in SPLAT II particle detection rates in the ODS1 and ODS2 are continuously monitored and recorded. The ratios of these two rates also provide a measure of particle beam divergence and hence information about particle asphericity.
Since the vertical dimensions of the IR and UV laser beams (1 mm and 770 μm, respectively) are significantly larger than those of green laser beams, reduction in sensitivity due to particle asphericity is completely determined by particle detection probabilities.
Ion Generation and TOF-MS
Once a particle is detected and properly sized, two laser triggers are generated: the first fires the CO2 laser and the second is used to fire the excimer laser. As described above, the timing of the triggers for these lasers are set by the operator during instrument alignment. And, to assure that the hit-rate is size independent, the laser trigger delay coefficients for both lasers depend on particle size.
The particle is hit first with the CO2 laser and the semivolatile components are allowed to evaporate for a few microseconds, at which point the excimer laser is fired, ions are generated, and an individual particle mass spectrum is acquired. shows an example of a mass spectrum of a single 100 nm particle composed of trans-sobrerol, one of the products formed by α-pinene oxidation at high relative humidity (CitationYu et al. 2008a; CitationYu et al. 2008b). In this case the excimer laser was triggered 5 μsec after the CO2 laser pulse. For comparison shows the reference 70 eV electron impact ionization mass spectrum of its stereoisomer from the NIST Standard Reference Database.
FIG. 6 (a) Mass spectrum of a single 100 nm particle composed of trans-sobrerol produced by SPLAT II; (b) Reference NIST mass spectrum of trans-sobrerol stereoisomer.
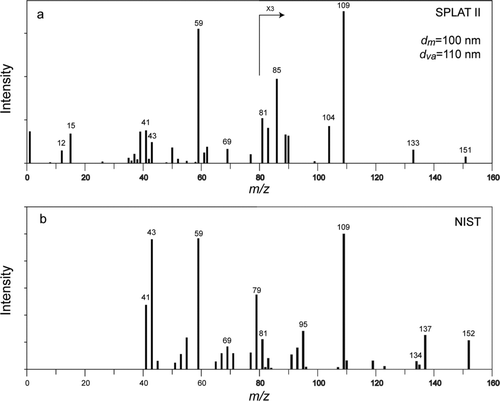
When ions are generated in the two-step IR/UV mode the operator has the option to set the delay between the evaporating and ionizing lasers to reveal different fractions of the particle composition. This option is particularly of interest when individual particles are composed of a mixture of semivolatile and nonvolatile compounds. In this case the IR laser evaporates the semivolatile compounds while the nonvolatile fraction remains in the condensed phase. By controlling the delay between the two lasers it is possible to trigger the excimer laser early - before the particle center-of-mass reaches the excimer laser beam, or late- after the center-of-mass passes the excimer laser beam. In either of these cases, since the excimer laser misses the particle center-of-mass, the only generated ions are from the evaporating plume of the semivolatile fraction. When, on the other hand, the excimer laser is triggered to hit the particle center-of-mass, both fractions are ionized and the mass spectrum exhibits signatures from both.
Figures 7a and 7b show an example of such an experiment. Here, dry NaCl particles were classified by a DMA at 146 nm and then coated with DOP to form spherical particles (CitationZelenyuk et al. 2008d). A second DMA was used to classify coated particles with diameter of 257 nm. On the basis of the measured dynamic shape factor of the NaCl core, its volume equivalent diameter is calculated to be 139 nm, yielding a DOP coat thickness of 59 nm. Here, coat thickness is defined as the difference between the volume equivalent radii of the NaCl core and that of the coated particle. The calculated volume and weight fractions of NaCl in these particles are 0.16 and 0.2, respectively, yielding a calculated density of 1.17 gcm−3, which is in very good agreement with the measured particle density of 1.164 gcm−3.
shows an average mass spectrum of these DOP coated NaCl particles that was obtained by delaying the excimer laser sufficiently long to allow the center-of-mass, containing the nonvolatile NaCl core to pass by. In this case the excimer laser missed the nonvolatile NaCl core and ionized the back end of the evaporated DOP plume. As expected, this average mass spectrum shows virtually no trace of NaCl. shows that when the delay between the two lasers was decreased to the point where the excimer laser hit the particle center-of-mass, the NaCl mass spectral peaks became clearly visible. Note that the mass spectrum in is not completely dominated by the Na+ peak, as often is the case for ablation generated mass spectra (CitationCai et al. 2006; CitationZelenyuk et al. 2008d).
FIG. 7 Average mass spectra of DOP coated NaCl particles, obtained in the IR/UV mode with the excimer laser delayed to miss the particle center-of-mass (a) and hit the particle center-of-mass (b).
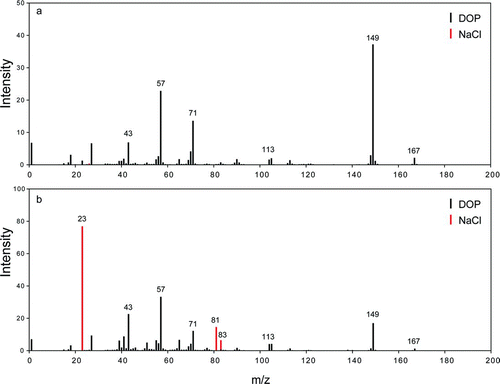
When studies are conducted in the laboratory with particles of known compositions, it might be possible, even when a single UV laser is used, to carefully tune the ablation laser power and generate reasonably high quality mass spectra (CitationWenzel and Prather 2004; CitationZelenyuk et al. 2009a). However, when SPMSs are deployed in the field, they have to be operated at sufficiently high laser powers to assure good detection probabilities of particles composed of a wide range of compounds, including those that are difficult to ionize, of which sulfates are the most common example. At these, higher laser powers organic compounds tend to fragment to the point where their identification becomes almost impossible. These problems are eliminated when the organic compounds are first evaporated with the IR laser pulse.
We have recently conducted a detailed comparative study between mass spectra of individual organic particles generated by UV laser ablation and in the two-step IR/UV mode, the results of which are presented elsewhere (CitationZelenyuk et al. 2009a). Here we provide, in an illustrative example from that study. shows the average mass spectra of 334 nm DOP particles that were generated in the IR/UV mode () and by UV ablation (). The UV laser fluence, of 0.93 Jcm−2 used in this set of experiments was chosen to assure efficient ionization of ammonium sulfate. The mass spectrum generated in the IR/UV mode exhibits significant intensity in mass spectral peaks at m/z= 149 (C6H4(CO)2OH+) and m/z= 167 (C6H4(CO)2(OH)2H+) that are characteristic of phthalates. Moreover, we find that the relative peak intensities of the individual particle mass spectra generated in the IR/UV mode vary only slightly, even when the UV laser fluence is changed by as much as a factor of 10. In contrast, the ablation generated mass spectrum shown in exhibits a significant degree of fragmentation and cannot be used to identify the presence of phthalate in these particles. The most intense peaks in this mass spectrum are those of C+ 1, C+ 2, and C+ 3. An investigation of the dependence of the mass spectral pattern on the ablation laser power shows that ablation generated mass spectra of DOP particles exhibit significant intensity in the typical phthalate peaks at m/z= 149 and 167 when the ablation laser power is reduced to 0.07 Jcm−2, at which point sulfate and many other atmospheric constituents cannot be detected.
FIG. 8 Average mass spectra of 334 nm particles composed of DOP obtained in the IR/UV mode (a) and by UV laser ablation (b). Bar graph representations of the classification results for the IR/UV mode (c) and for UV ablation (d).
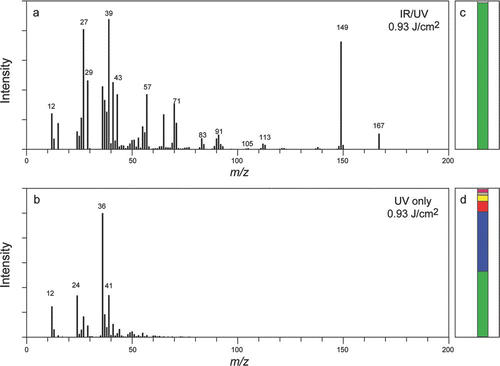
We find that in addition to the loss of compound characteristic mass spectral peaks ablation-generated mass spectra exhibit strong dependence on laser power and large particle-to-particle variability. Since in SPMS individual particle mass spectra are first classified and only then averaged, particle-to-particle variability in mass spectral intensity patterns plays a critical role. Whether two particles with identical compositions are properly identified as such, and classified together depends on how similar their mass spectra are. and provide a glimpse into this issue. The bar graphs in these figures indicate the fraction of mass spectra that were classified into separate clusters. shows that mass spectra that were generated in the IR/UV mode yield significantly improved results, where 98% of the mass spectra were classified into the same cluster. shows that ablation generated mass spectra were classified, under the very same conditions, into seven clusters. Moreover, the average mass spectrum of the largest cluster in this example contains very little information aside the pure carbon atom progression.
Aircraft Performance
In preparation for ISDAC, SPLAT II was flown on two short test-flights on board of DOE's G-1 research aircraft over the Pacific Northwest. In both of these flights the instrument characterized thousands of particles and performed without a flaw. Shortly thereafter, SPLAT II was installed on the Canadian National Research Council Convair 580 aircraft for participation in ISDAC—a month-long field campaign at the North Slope of Alaska.
One of ISDAC's main scientific objectives was to improve our understanding of the processes that control cloud formation with special emphasis on ice-clouds. To this end the aircraft was equipped with two aerosol inlets: a Counterflow Virtual Impactor (CVI) inlet that was used to sample particles that were activated as cloud condensation nuclei and ice nuclei, and an aerosol inlet that was used to sample all small particles in clear sky.
During ISDAC SPLAT II participated in 27 flights that lasted slightly over 100 h. It measured the size of tens of millions of particles and characterized the composition of ∼3 million of them. Since the main goal of this campaign was to characterize cloud properties, a significant fraction of the data was collected through the CVI inlet, which significantly limited the number of sampled particles. The instrument remained aligned throughout the entire field campaign and operated on each of the flights.
During ISDAC SPLAT II measured a wide range of particle compositions, including sulfates mixed with organics, nitrates mixed with organic, organics, soot, processed and freshly emitted sea-salt, dust particles, and a large number of biomass burning particles. Many of these particle types appeared in aerosol layers that had horizontal and vertical filamentous structures.
shows two examples of individual particle mass spectra recorded during ISDAC. The mass spectrum in is of a biomass burning particle. These particles were rather prevalent over the North Slope of Alaska during the campaign. Satellite data and back trajectories indicate that they were transported from Asia. The ice-clouds sampled in ISDAC had typically ice crystal concentrations that were lower than 50 crystals per liter. Correspondingly, when sampling through the CVI inlet the numbers of characterized particles dropped precipitously. Despite the low number concentrations SPLAT II was able to measure the size and composition of thousands of ice-nuclei during the campaign. shows a mass spectrum of a Ca-dominated dust particle containing a small amount of sulfate that served as an ice-cloud nucleus, sampled through the CVI inlet. Preliminary analysis of the data indicates that ice nuclei were mostly crustal or metallic particles.
FIG. 9 Mass spectra of two particles recorded onboard of Convair 580 aircraft during ISDAC. (a) A Ca-dominated dust particle containing a small amount of sulfate that served as an ice-cloud nucleus; (b) A biomass burning particle.
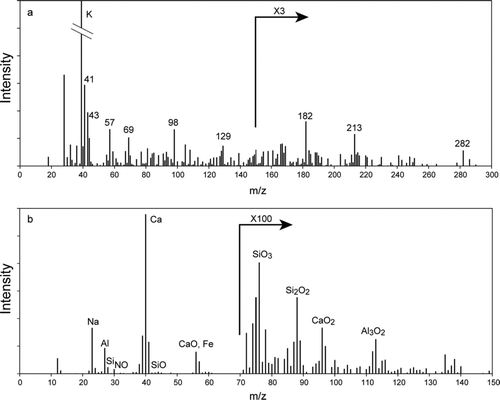
Since the CVI inlet transmits, in addition to ice crystals, liquid droplets, SPLAT II was also able to characterize a large number of particles that served as cloud condensation nuclei as well. The data from this field campaign are in the very early stages of analysis.
CONCLUSIONS
We presented SPLAT II, a new aircraft compatible single particle mass spectrometer. The footprint and weight of this instrument are significantly smaller than those of SPLAT, yet it is significantly more robust and user-friendly. Many of the new design elements in this instrument are intended to make it aircraft compatible. SPLAT II is significantly easier to align in a reproducible manner and it remains aligned for long periods of time even on an aircraft. It was successfully operated on all the flights during a month-long aircraft based field campaign, remaining aligned for the entire duration.
SPLAT II represents significant improvements in instrument sensitivity and sampling rate. Spherical 100 nm particles are detected with 40% efficiency and those that are larger than 125 nm and smaller than 600 nm are detected with 100% efficiency. The detection efficiency of aspherical particles is only a factor of ∼3 lower, representing large improvements over SPLAT. The instrument temporal resolution has also been significantly increased. SPLAT II can size over 500 particles per second and record up to 100 individual particle mass spectra per second. It has sizing precision that is better than 0.5%, making it possible to measure the density of spherical particles and the effective density of aspherical particles classified by a DMA with precision of 0.5%. The line-shape of the vacuum aerodynamic size distribution of DMA classified particles can be used to distinguish between spherical and aspherical particles. For some particle systems the dynamic shape factors and fractal dimensions can also be determined.
The individual particle mass spectra are generated in SPLAT II in a two-step process, in which an IR laser is used to evaporate the particle and a time-delayed UV laser pulse is used to create the ions. The instrument can be used to characterize the compositions of particles containing semivolatile and nonvolatile compounds. This two-step IR/UV approach generates reproducible mass spectra with significantly reduced fragmentation and matrix effects. The mass spectra are recorded with a 14-bit A/D data acquisition board.
The new instrument control board provides an intuitive graphical interface for instrument alignment and operations, and uses a triggering scheme that takes into account particle size to eliminate a commonly observed size dependent hit-rate.
Acknowledgments
We thank James Ewing, John Hubbe, and the crews of Convair 580 and G-1 aircrafts for their incredible help in making SPLAT II aircraft compatible. We also thank Dan Sisk for his help and support in developing the instrument control board. This work was supported by the U.S. Department of Energy Office of Basic Energy Sciences, Chemical Sciences Division, and Energy Efficiency and Renewable Energy. This research was performed in the Environmental Molecular Sciences Laboratory, a national scientific user facility sponsored by the Department of Energy's Office of Biological and Environmental Research at Pacific Northwest National Laboratory (PNNL). PNNL is operated by the US Department of Energy by Battelle Memorial Institute under contract No. DE-AC06-76RL0 1830.
REFERENCES
- Allen , J. O. , Bhave , P. V. , Whiteaker , J. R. and Prather , K. A. 2006 . Instrument Busy Time and Mass Measurement Using Aerosol Time-of-Flight Mass Spectrometry . Aerosol Sci. Technol. , 40 : 615 – 626 .
- Baer , T. , Cabalo , J. , Zelenyuk , A. and Miller , R. E. 1999 . Two-Color Laser Evaporation and Laser Ionization Mass Spectrometry of Aerosol Particles . Abstracts of Papers of the American Chemical Society , 217 : U145 – U145 .
- Cabalo , J. , Zelenyuk , A. , Baer , T. and Miller , R. E. 2000 . Two-Color Laser Induced Evaporation Dynamics of Liquid Aerosols Probed by Time-of-Flight Mass Spectrometry . Aerosol Sci. Technol. , 33 : 3 – 19 .
- Cai , Y. , Zelenyuk , A. and Imre , D. 2006 . A High Resolution Study of the Effect of Morphology on the Mass Spectra of Single PSL Particles with Na-Containing Layers and Nodules . Aerosol Sci. Technol. , 40 : 1111 – 1122 .
- Cross , E. S. , Slowik , J. G. , Davidovits , P. , Allan , J. D. , Worsnop , D. R. , Jayne , J. T. , Lewis , D. K. , Canagaratna , M. and Onasch , T. B. 2007 . Laboratory and Ambient Particle Density Determinations Using Light Scattering in Conjunction with Aerosol Mass Spectrometry . Aerosol Sci. Technol. , 41 : 343 – 359 .
- DeCarlo , P. F. , Slowik , J. G. , Worsnop , D. R. , Davidovits , P. and Jimenez , J. L. 2004 . Particle Morphology and Density Characterization by Combined Mobility and Aerodynamic Diameter Measurements. Part 1: Theory . Aerosol Sci. Technol. , 38 : 1185 – 1205 .
- Erdmann , N. , Dell'Acqua , A. , Cavalli , P. , Gruning , C. , Omenetto , N. , Putaud , J. P. , Raes , F. and Van Dingenen , R. 2005 . Instrument Characterization and First Application of the Single Particle Analysis and Sizing System (SPASS) for Atmospheric Aerosols . Aerosol Sci. Technol. , 39 : 377 – 393 .
- Gard , E. , Mayer , J. E. , Morrical , B. D. , Dienes , T. , Fergenson , D. P. and Prather , K. A. 1997 . Real-Time Analysis of Individual Atmospheric Aerosol Particles: Design and Performance of a Portable ATOFMS . Anal. Chem. , 69 : 4083 – 4091 .
- Ge , Z. Z. , Wexler , A. S. and Johnston , M. V. 1998 . Laser Desorption/Ionization of Single Ultrafine Multicomponent Aerosols . Environ. Sci. Technol. , 32 : 3218 – 3223 .
- Gross , D. S. , Galli , M. E. , Silva , P. J. and Prather , K. A. 2000 . Relative Sensitivity Factors for Alkali Metal and Ammonium Cations in Single Particle Aerosol Time-of-Flight Mass Spectra . Anal. Chem. , 72 : 416 – 422 .
- Hinz , K. P. , Greweling , M. , Drews , F. and Spengler , B. 1999 . Data Processing in On-Line Laser Mass Spectrometry of Inorganic, Organic, or Biological Airborne Particles . J. Am. Soc. Mass Spectrom. , 10 : 648 – 660 .
- Hinz , K. P. and Spengler , B. 2007 . Instrumentation, Data Evaluation and Quantification in On-Line Aerosol Mass Spectrometry . J. Mass Spectrom. , 42 : 843 – 860 .
- Huffman , J. A. , Jayne , J. T. , Drewnick , F. , Aiken , A. C. , Onasch , T. , Worsnop , D. R. and Jimenez , J. L. 2005 . Design, Modeling, Optimization, and Experimental Tests of a Particle Beam Width Probe for the Aerodyne Aerosol Mass Spectrometer . Aerosol Sci. Technol. , 39 : 1143 – 1163 .
- Jayne , J. T. , Leard , D. C. , Zhang , X. F. , Davidovits , P. , Smith , K. A. , Kolb , C. E. and Worsnop , D. R. 2000 . Development of an Aerosol Mass Spectrometer for Size and Composition Analysis of Submicron Particles . Aerosol Sci. Technol. , 33 : 49 – 70 .
- Johnston , M. V. 2000 . Sampling and Analysis of Individual Particles by Aerosol Mass Spectrometry . J. Mass Spectrom. , 35 : 585 – 595 .
- Kleinman , L. I. , Springston , S. R. , Daum , P. H. , Lee , Y. N. , Nunnermacker , L. J. , Senum , G. I. , Wang , J. , Weinstein-Lloyd , J. , Alexander , M. L. , Hubbe , J. , Ortega , J. , Canagaratna , M. R. and Jayne , J. 2008 . The Time Evolution of Aerosol Composition Over the Mexico City Plateau . Atmos. Chem. Phys. , 8 : 1559 – 1575 .
- Liu , P. , Ziemann , P. J. , Kittelson , D. B. and Mcmurry , P. H. 1995a . Generating Particle Beams of Controlled Dimensions and Divergence. 1. Theory of Particle Motion in Aerodynamic Lenses and Nozzle Expansions . Aerosol Sci. Technol. , 22 : 293 – 313 .
- Liu , P. , Ziemann , P. J. , Kittelson , D. B. and Mcmurry , P. H. 1995b . Generating Particle Beams of Controlled Dimensions and Divergence. 2. Experimental Evaluation of Particle Motion in Aerodynamic Lenses and Nozzle Expansions . Aerosol Sci. Technol. , 22 : 314 – 324 .
- Liu , P. S. K. , Deng , R. , Smith , K. A. , Williams , L. R. , Jayne , J. T. , Canagaratna , M. R. , Moore , K. , Onasch , T. B. , Worsnop , D. R. and Deshler , T. 2007 . Transmission Efficiency of an Aerodynamic Focusing Lens System: Comparison of Model Calculations and Laboratory Measurements for the Aerodyne Aerosol Mass Spectrometer . Aerosol Sci. Technol. , 41 : 721 – 733 .
- McMurry , P. H. 2000 . A Review of Atmospheric Aerosol Measurements . Atmos. Environ. , 34 : 1959 – 1999 .
- Morrical , B. D. , Fergenson , D. P. and Prather , K. A. 1998 . Coupling Two-Step Laser Desorption/Ionization with Aerosol Time-of-Flight Mass Spectrometry for the Analysis of Individual Organic Particles . J. Am. Soc. Mass Spectrom. , 9 : 1068 – 1073 .
- Murphy , D. M. 2007 . The Design of Single Particle Laser Mass Spectrometers . Mass Spectrom. Rev. , 26 : 150 – 165 .
- Murphy , D. M. , Middlebrook , A. M. and Warshawsky , M. 2003 . Cluster Analysis of Data from the Particle Analysis by Laser Mass Spectrometry (PALMS) Instrument . Aerosol Sci. Technol. , 37 : 382 – 391 .
- Nam , E. J. , Han , Y. , Mueller , K. , Zelenyuk , A. and Imre , D. 2007 . “ ClusterSculptor: A Visual Analytics Tool for High-Dimensional Data ” . In EEE Symposium on Visual Analytics Science and Technology, VAST 2007. 75 – 82 .
- Nash , D. G. , Baer , T. and Johnston , M. V. 2006 . Aerosol Mass Spectrometry: An Introductory Review . Int. J. Mass Spectrom. , 258 : 2 – 12 .
- Noble , C. A. and Prather , K. A. 2000 . Real-Time Single Particle Mass Spectrometry: A Historical Review of a Quarter Century of the Chemical Analysis of Aerosols . Mass Spectrom. Rev. , 19 : 248 – 274 .
- Phares , D. J. , Rhoads , K. P. , Wexler , A. S. , Kane , D. B. and Johnston , M. V. 2001 . Application of the ART-2a Algorithm to Laser Ablation Aerosol Mass Spectrometry of Particle Standards . Anal. Chem. , 73 : 2338 – 2344 .
- Rebotier , T. P. and Prather , K. A. 2007 . Aerosol Time-of-Flight Mass Spectrometry Data Analysis: A Benchmark of Clustering Algorithms . Anal. Chim. Acta , 585 : 38 – 54 .
- Reents , W. D. , Downey , S. W. , Emerson , A. B. , Mujsce , A. M. , Muller , A. J. , Siconolfi , D. J. , Sinclair , J. D. and Swanson , A. G. 1995 . Single-Particle Characterization by Time-of-Flight Mass–Spectrometry . Aerosol Sci. Technol. , 23 : 263 – 270 .
- Reilly , P. T. A. , Lazar , A. C. , Gieray , R. A. , Whitten , W. B. and Ramsey , J. M. 2000 . The Elucidation of Charge-Transfer-Induced Matrix Effects in Environmental Aerosols via Real-Time Aerosol Mass Spectral Analysis of Individual Airborne Particles . Aerosol Sci. Technol. , 33 : 135 – 152 .
- Rhoads , K. P. , Phares , D. J. , Wexler , A. S. and Johnston , M. V. 2003 . Size–Resolved Ultrafine Particle Composition Analysis, 1. Atlanta . J. Geophys Res. [Atmos.] , 108
- Salcedo , D. , Onasch , T. B. , Canagaratna , M. R. , Dzepina , K. , Huffman , J. A. , Jayne , J. T. , Worsnop , D. R. , Kolb , C. E. , Weimer , S. , Drewnick , F. , Allan , J. D. , Delia , A. E. and Jimenez , J. L. 2007 . Technical Note: Use of a Beam Width Probe in an Aerosol Mass Spectrometer to Monitor Particle Collection Efficiency in the Field . Atmos. Chem. Phys. , 7 : 549 – 556 .
- Song , X. H. , Hopke , P. K. , Fergenson , D. P. and Prather , K. A. 1999 . Classification of Single Particles Analyzed by ATOFMS Using an Artificial Neural Network, ART-2A . Anal. Chem. , 71 : 860 – 865 .
- Su , Y. X. , Sipin , M. F. , Furutani , H. and Prather , K. A. 2004 . Development and Characterization of an Aerosol Time-of-Flight Mass Spectrometer with Increased Detection Efficiency . Anal. Chem. , 76 : 712 – 719 .
- Suess , D. T. and Prather , K. A. 1999 . Mass Spectrometry of Aerosols . Chem. Rev. , 99 : 3007 – + .
- Sullivan , R. C. and Prather , K. A. 2005 . Recent Advances in Our Understanding of Atmospheric Chemistry and Climate Made Possible by On-Line Aerosol Analysis Instrumentation . Anal. Chem. , 77 : 3861 – 3885 .
- Sykes , D. C. , Woods , E. , Smith , G. D. , Baer , T. and Miller , R. E. 2002 . Thermal Vaporization–Vacuum Ultraviolet Laser Ionization Time-of-Flight Mass Spectrometry of Single Aerosol Particles . Anal. Chem. , 74 : 2048 – 2052 .
- Thomson , D. S. , Schein , M. E. and Murphy , D. M. 2000 . Particle Analysis by Laser Mass Spectrometry WB-57F Instrument Overview . Aerosol Sci. Technol. , 33 : 153 – 169 .
- Trimborn , A. , Hinz , K. P. and Spengler , B. 2000 . Online Analysis of Atmospheric Particles With a Transportable Laser Mass Spectrometer . Aerosol Sci. Technol. , 33 : 191 – 201 .
- Wenzel , R. J. and Prather , K. A. 2004 . Improvements in Ion Signal Reproducibility Obtained Using a Homogeneous Laser Beam for On-Line Laser Desorption/Ionization of Single Particles . Rapid Commun. Mass Spectrom. , 18 : 1525 – 1533 .
- Woods , E. , Smith , G. D. , Dessiaterik , Y. , Baer , T. and Miller , R. E. 2001 . Quantitative Detection of Aromatic Compounds in Single Aerosol Particle Mass Spectrometry . Anal. Chem. , 73 : 2317 – 2322 .
- Yu , Y. , Ezell , M. , Zelenyuk , A. , Imre , D. , Alexander , L. , Ortega , J. , D'Anna , B. , Harmon , C. W. , Johnson , S. N. and Finlayson-Pitts , B. J. 2008a . Photooxidation of Alpha-Pinene at High Relative Humidities in the Presence of Increasing Concentrations of NOx . Atmos. Environ. , 42 : 5044 – 5060 .
- Yu , Y. , Ezell , M. J. , Zelenyuk , A. , Imre , D. , Alexander , L. , Ortega , J. , Thomas , J. L. , Gogna , K. , Tobias , D. J. , D'Anna , B. , Harmon , C. W. , Johnson , S. N. and Finlayson-Pitts , B. J. 2008b . Nitrate Ion Photochemistry at Interfaces: A New Mechanism for Oxidation of -Pinene . Phys. Chem. Chem. Phys. , 10 : 3063 – 3071 .
- Zelenyuk , A. , Cai , Y. , Chieffo , L. and Imre , D. 2005 . High Precision Density Measurements of Single Particles: The Density of Metastable Phases . Aerosol Sci. Technol. , 39 : 972 – 986 .
- Zelenyuk , A. , Cai , Y. and Imre , D. 2006a . From Agglomerates of Spheres to Irregularly Shaped Particles: Determination of Dynamic Shape Factors from Measurements of Mobility and Vacuum Aerodynamic Diameters . Aerosol Sci. Technol. , 40 : 197 – 217 .
- Zelenyuk , A. , Cuadra-Rodriguez , L. A. , Imre , D. , Shimpi , S. and Warey , A. 2006b . Comprehensive Characterization of Ultrafine Particulate Emission from 2007 Diesel Engines: PM Size Distribution, Loading and Individual Particle Size and Composition . Eos Trans. AGU , Abstract A43A–0121
- Zelenyuk , A. and Imre , D. 2007 . On the Effect of Particle Alignment in the DMA . Aerosol Sci. Technol. , 41 : 112 – 124 .
- Zelenyuk , A. and Imre , D. 2005 . Single Particle Laser Ablation Time-of-Flight Mass Spectrometer: An Introduction to SPLAT . Aerosol Sci. Technol. , 39 : 554 – 568 .
- Zelenyuk , A. , Imre , D. , Cai , Y. , Mueller , K. , Han , Y. P. and Imrich , P. 2006c . SpectraMiner, an Interactive Data Mining and Visualization Software for Single Particle Mass Spectroscopy: A Laboratory Test Case . Int. J. Mass Spectrom. , 258 : 58 – 73 .
- Zelenyuk , A. , Imre , D. , Cuadra-Rodriguez , L. A. and Ellison , B. 2007 . Measurements and Interpretation of the Effect of a Soluble Organic Surfactant on the Density, Shape and Water Uptake of Hygroscopic Particles . J. Aerosol Sci. , 38 : 903 – 923 .
- Zelenyuk , A. , Imre , D. , Han , J. H. and Oatis , S. 2008a . Simultaneous Measurements of Individual Ambient Particle Size, Composition, Effective Density, and Hygroscopicity . Anal. Chem. , 80 : 1401 – 1407 .
- Zelenyuk , A. , Imre , D. , Nam , E. J. , Han , Y. and Mueller , K. 2008b . ClusterSculptor: Software for Expert–Steered Classification of Single Particle Mass Spectra . Int. J. Mass Spectrom. , 275 : 1 – 10 .
- Zelenyuk , A. , Yang , J. , Song , C. , Zaveri , R. and Imre , D. 2008c . A New Real-Time Method for Determining Particles' Density and Sphericity: Application to Secondary Organic Aerosol from a–Pinene . Environ. Sci. Technol. , 42 : 8033 – 8038 .
- Zelenyuk , A. , Yang , J. , Song , C. , Zaveri , R. A. and Imre , D. 2008d . “Depth-Profiling” and Quantitative Characterization of the Size, Composition, Shape, Density, and Morphology of Fine Particles with SPLAT, a Single-Particle Mass Spectrometer . J. Phys. Chem. A , 112 : 669 – 677 .
- Zelenyuk , A. , Yang , J. and Imre , D. 2009a . Comparison between Mass Spectra of Individual Organic Particles Generated by UV Laser Ablation and in the IR/UV Two-Step Mode . Int. J. Mass Spectrom. , in press
- Zelenyuk , A. , Yang , J. , Imre , D. and Choi , E. 2009b . Achieving Size Independent Hit-Rate in Single Particle Mass Spectrometry . Aerosol Sci. Technol. Aerosol. Sci. Techno. , 43 : 305 – 310 .