Abstract
Although commuting time typically accounts for only 6% of the day for Americans, it has become a significant source of exposure to ultrafine particles (d p < 0.1 μ m) from vehicular emissions. Particle deposition onto surfaces, as an important particle loss mechanism, has been studied extensively in the indoor environments. However since air velocities, surface area to volume ratios and other contributing factors differ greatly between indoor and in-cabin environments, conclusions from indoor studies may not be directly applied to in-cabin microenvironments. In this study, ultrafine particle deposition rates were characterized under a range of air velocities and surface areas conditions inside different types of passenger vehicles. A diesel generator was used as a particle source and a Scanning Mobility Particle Sizer (SMPS) was used to measure ultrafine particle size distribution inside the test vehicles. As in-cabin air velocities increased from natural convection (< 0.02 m s–1 ) to 0.65 m s–1 , ultrafine particle deposition rates increased with the greatest increases occurred for smaller particles. Other influencing factors, such as the number of passengers inside the vehicle, were also considered and investigated. It was found that ultrafine particle deposition rates are proportional to the surface areas inside vehicles consistent with previous indoor studies. Compared with available ultrafine particle deposition rates reported in the indoor environments, the in-cabin ultrafine particle deposition rates found in this study are about 3 to 20 times greater. This is likely due to higher air velocities and larger surface area to volume ratios in the in-cabin microenvironment.
INTRODUCTION
Epidemiological studies worldwide have demonstrated a strong correlation between exposures to particulate matter (PM) and increasing rates of respiratory and cardiovascular illness and other adverse health effects (CitationDockery 2001; CitationBeckett 2002; CitationDonaldson et al. 2002; CitationPope et al. 2002; CitationMacNee and Donaldson 2003; CitationPope and Dockery 2006). Ultrafine particles, due to their distinctive composition and size, pose a greater threat to human health, and have greater toxicity in comparison with larger particles (CitationXia et al. 2004; CitationNel et al. 2006; CitationKleinman et al. 2008; CitationNemmar and Inuwa 2008). In modern times, people spend more time indoors than outdoors and experience personal exposure indoors, where particle concentrations often exceed outdoor concentrations (CitationWallace 1996; CitationMonn 2001). In-cabin microenvironments similar to indoor environments have become a significant source of exposure to ultrafine particles from vehicular emissions due to the high in-vehicle concentrations typically in the range of 105 particles cm–3 (CitationZhu et al. 2007). These exposures are significant, although the commuting time accounts for only 6% of the day for Americans (CitationKlepeis et al. 2001). Further evidence by an on-roadway study in Los Angeles indicated that 33–45% of total daily ultrafine particle exposure was associated with time spent traveling in vehicles (Fruin et al. 2007). CitationZhu et al. (2007) also reported that, for one hour daily commute exposure, the in-vehicle microenvironment contributed 10–50% of people's daily exposure to ultrafine particles from traffic.
Unlike gaseous molecules, aerosol particles can adhere to the surface of the wall when they hit a wall and then can not be inhaled unless resuspended. As a result, particle deposition onto indoor surfaces is an important particle removal process, which substantially reduces indoor airborne particle concentrations and plays a critical role in estimating indoor particle exposure and the associated health effects.
Most previous indoor studies on particle deposition were conducted in experimental chambers (CitationByrne et al. 1995; CitationThatcher et al. 1995; CitationMorawska and Jamriska 1996; CitationCheng 1997; CitationNomura et al. 1997; CitationAbadie et al. 2001; CitationMosley et al. 2001; CitationLai et al. 2002; CitationLai and Nazaroff 2005; CitationLai 2006; CitationAfshari and Reinhold 2008), and in residential or experimentally controlled houses (CitationFogh et al. 1997; CitationAbt et al. 2000; CitationLong et al. 2001; CitationVette et al. 2001; CitationRiley et al. 2002; CitationThatcher et al. 2002; CitationHoward-Reed et al. 2003; CitationWallace et al. 2004a; CitationHe et al. 2005). Besides particle deposition, these studies also laid emphasis upon the effects of other influencing factors on indoor particle concentrations, such as the indoor-outdoor particle concentration ratio, the penetration rate of outdoor particles, and indoor particle concentrations due to daily human activities. Although a large variability in particle deposition rates existed among different studies, some consistencies have been reached. For example, indoor particle deposition onto surfaces is due primarily to turbulent diffusion to the boundary layer (CitationCheng 1997; CitationNomura et al. 1997). Particle deposition rates as a function of particle size were found to follow a U-shaped distribution. For smaller particles (< 0.1 μ m), diffusion is dominant, while gravitation is dominant for larger particles (> 1 μ m). For particle sizes between 0.1 μ m and 1 μ m, where the minimum of particle deposition rates occurs, a mixture of both mechanisms may be assumed. Although indoor particle deposition has been extensively studied over the past several decades, those conclusions may not be directly applied to the in-cabin microenvironments where no deposition experiments were intentionally carried out before. Due to the uniqueness of in-cabin microenvironments, air velocities and surface area to volume ratios differ greatly between the indoor and the in-cabin environments. Only CitationOtt et al. (2007) reported in-vehicle pollutant concentrations from secondhand smoke and particle deposition rates under high air exchange rates on a preliminary scale. The specific objectives of this study are to: (1) quantify ultrafine particle deposition rates inside passenger vehicles; (2) investigate the effects of in-cabin air velocities and surface areas on ultrafine particle deposition rates; and (3) compare ultrafine particle deposition rates between the in-cabin and indoor environments.
METHODS
Test Vehicles
Three different types of vehicles (1997 Isuzu SUV; 1996 Saturn SL2 car; and 2005 Ford F-250 Super Duty truck) were employed as test vehicles to represent a range of passenger vehicles in our daily life. Particle deposition measurements were completed inside these vehicles. summarizes the in-cabin volume and the interior surface areas of each test vehicle. For interior surface area, additional areas due to distinctive and complex in-cabin geometric structure such as seats, dashboards, middle separators and other inner furnishings, were included. The Ford pick-up truck has the smallest in-cabin space because the majority of its body is occupied by the back hopper, which leads to the largest surface area to volume ratio (S/V), compared with the SUV and the passenger car. The SUV, due to its special sports utility characteristic, has a larger car size and its trunk is not separated with the in-cabin microenvironment. Generally speaking, cotton, artificial leather, carpet, plastic, rubber, flax, and other materials were widely applied as surface textures and frequently observed in in-cabin microenvironments. Different materials lead to disparate surface roughness that has been found to have a great impact on indoor particle deposition (CitationAbadie et al. 2001; CitationLai et al. 2002; CitationLai and Nazaroff 2005). The interior surface materials inside three test vehicles are also given in . Other important parameters inside test vehicles during the experiments, such as in-cabin temperature, and relative humidity, were also recorded by Q-Trak (TSI Model 7565). The in-cabin temperature (30.12 ± 0.76°C) observed during the experiments was relatively stable from test to test. Higher relative humidity was observed during the experiments conducted inside the Saturn SL car (74.42 ± 4.68%) than other vehicles (43.91 ± 3.67%). The details of the equipment setup and experimental protocol are described in the following sections.
TABLE 1 Information of test vehicles in this study
In-Cabin Air Velocity Measurements
In order to simulate in-cabin air flow conditions under realistic driving conditions, the average air velocity inside the test vehicles at different driving speeds (20 to 60 mph) and various ventilation settings (fan on/off, recirculation (RC) on/off) were measured. All the windows and doors of the test vehicle were kept closed during the measurements. A hot-wire anemometer (TSI Model 9290) was used to measure the in-cabin air velocity every 30 seconds. presents the average air velocity and standard deviation as a function of vehicle speeds under different ventilation conditions. The air velocities observed in this study are greater than those reported in chambers or houses which are usually less than 0.2 m s–1 (CitationMatthews et al. 1989; CitationCheng 1997; CitationThatcher et al. 2002). When the car speed was 60 mph and the ventilation setting was fan on/RC off, the highest in-cabin air velocity occurred. When the car speed was 20 mph and fan off/RC off, the lowest air velocity occurred. This suggests that vehicle speeds and ventilation settings are primary contributors to in-cabin air velocity when windows are closed. Based on the in-cabin air velocity data presented in , four air flow conditions were employed to perform the in-cabin ultrafine particle deposition study in parked vehicles. These four conditions include natural convection with minimum in-cabin air motion (V0 < 0.02 m s–1), and three increasing air velocities (V1 = 0.25 m s–1, V2 = 0.45 m s–1, and V3 = 0.65 m s–1).
FIG. 1 The average air velocity inside test vehicles under various vehicle speeds and ventilation settings.
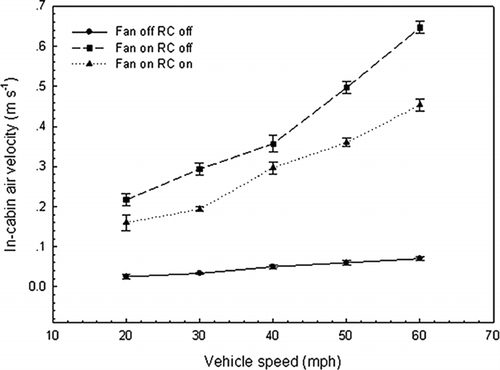
Prior to the deposition experiment, an additional 12 V DC cooling fan was mounted above the dashboard to change the air flow condition inside the vehicles. The test vehicles were always stationary during the experiments. The additional fan, which has been modified to be able to run at various speeds through altering the rheostat to change the applied voltage of the fan, was the only adjustment for the in-cabin air flow pattern. The vehicle fan and the recirculation system were not used during the experiments in order to exclude the effects on particle deposition from other competing factors such as particles removal by vehicle's own ventilation system. In addition, operating vehicle's own fan needs an energy supply, which means the vehicle engine need to be turned on. This will result in sampling the test vehicle's own exhaust plume which will affect the test results.
Surface Areas inside Test Vehicles
The number of passengers inside vehicles will change the in-cabin surface area. After measuring the interior surface areas inside three test vehicles, mannequins wearing T-shirts and blue jeans were used to increase in-cabin surface areas and to evaluate the effect of the surface area on particle deposition rates. Each mannequin has the surface area of about 1.8 m2 just like the average surface area of human skin (CitationDavis 1989). Additional surface areas from experimental instruments were also considered. In this study, putting mannequins into vehicles to simulate the conditions from no-passenger to three passengers (S0 to S3) sitting inside the vehicle was performed under natural convection (< 0.02 m s–1) without using the mixing fan to increase the in-cabin air velocity. Only the mannequin's surface areas were considered, but in reality fibers and textures also can contribute to additional surface areas, which may lead to the underestimation of the actual in-cabin surface area and the S/V ratio.
Particle Generation and Measurements
presents the schematic diagram of the experimental setup. A diesel generator (Red Hawk Equipment Model DG4LE) was used as the particle source for the deposition experiments. Before the exhaust injection, the mixing fan was switched on to produce specific in-cabin air flow conditions. Then the exhaust from the generator was injected into the vehicle after appropriate dilution with a system comprised of a mechanical blower, and aluminum and PVC ducts. Approximately 3 min later, the particle concentrations stabilized for all particle sizes, indicating that the particles were well mixed inside the test vehicle. At that time, the diesel generator was switched off and all the windows and doors of the test vehicles were closed. The mixing fan, the only adjustment for the in-cabin air flow pattern, was always switched on during the experiments, while the vehicle fan and recirculation system were never switched on during the experiments. Ultrafine particle deposition rates were determined based on size-resolved particle concentration decay sampled and recorded by a Scanning Mobility Particle Sizer (SMPS, TSI Model 3080) consisting of a differential mobility analyzer (DMA, TSI Model 3081) and a condensation particle counter (CPC, TSI Model 3785). Every single deposition experiment lasted for about 3 hours until the in-cabin ultrafine particle concentrations approached ambient levels. The in-cabin temperature, relative humidity (RH) and CO2 concentration were measured by Q-Trak (TSI Model 7565) with a 30-sec interval during the experiments. These instruments were set up and located inside the vehicles during the experiments.
Data analysis of the SMPS and Q-Trak output were done by the Aerosol Instrument Manager (TSI Version 7.3) and TrakPro software (TSI Version 3.62), respectively. In the present study, we selected the diesel generator as particle source because in-cabin particles from vehicular emissions are mainly in the ultrafine size range, and ultrafine particles are formed by nucleation during dilution and cooling of the exhaust from a diesel engine (Kittelson 1997). The diluted exhaust from the diesel generator provided enough initial ultrafine particle concentrations of approximately 105–106 particles cm–3 to estimate particle deposition rates while keeping the coagulation effect insignificant. Two lead acid batteries (East Penn Manufacturing Model 8G31DT) linked with a sine wave inverter (Xantrex Model Prisine 1000) were used as power supplies and were also placed inside the vehicle. Overall, eight sets of deposition experimental data, under four in-cabin air velocities and four in-cabin surface areas, were collected inside each test vehicle in the present study. The deposition rates reported in the following sections are for particles between 30 and 101.8 nm.
Air Exchange Rates
The in-cabin air exchange rate (AER) was estimated by the decay rate of CO2 concentrations measured with the Q-Trak (TSI Model 7565) during every deposition experiment. In general, CO2 concentration was rapidly elevated to 1200 ppm after injecting the diluted diesel generator exhaust and the background CO2 levels were usually around 350 ppm. The air exchange rate (h–1), α, is related to the change in CO2 concentration with time by
Calculation of Particle Deposition Rates
CitationCrump and Seinfeld (1981) derived a general formula for the aerosol deposition rate β in a turbulently mixed, enclosed vessel of arbitrary shape, taking into account deposition due to turbulent diffusion, Brownian diffusion and gravitational sedimentation. CitationNazaroff et al. (1993) described the decay of an initial aerosol concentration C0 with zero air exchange rate as
RESULTS AND DISCUSSION
The Effect of the In-Cabin Air Velocity on Particle Deposition Rates
The particle deposition is exponential (e.g., first-order) in the enclosure. An example of the relationship between ln(C/C0) and time for three selected particle sizes during the experiments conducted inside the Isuzu SUV is given in . Ultrafine particles quickly deposited onto internal surfaces inside the vehicle. Smaller particles need less time to finish the deposition process compared with larger particles. The linear relationship curves under four in-cabin air velocities conditions track more closely for larger particles indicating that the air velocity exhibits weaker effects on particle deposition rates for larger particles. The value of the slope is equal to particle deposition rate and increased with increasing in-cabin air velocity. Together with data presented in , ultrafine particles are found to be removed more efficiently and their concentrations are reduced more rapidly inside vehicles driving at a greater speed. Similar phenomena were also observed during the experiments conducted inside the Ford F-250 Super Duty truck and the Saturn SL2 car.
FIG. 3 The relationship between ln(C/C0) and time for (a) 30 nm, (b) 66.1 nm, and (c) 101.8 nm particles inside the Isuzu Rodeo SUV.
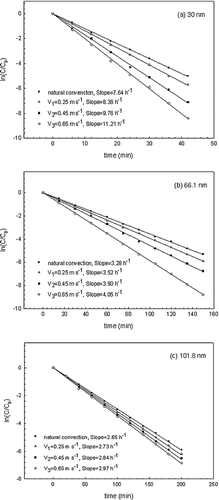
In this study, three replicate runs for each condition showed the experimental results were consistent from test to test and the mean values were reported. illustrates the effect of in-cabin air velocities on the deposition rate inside three test vehicles. For all the vehicles, when the in-cabin air velocities increased from the natural convection (< 0.02 m s–1) to 0.65 m s–1, the particle deposition rates increased for all studied particles sizes with the greatest enhancement observed for smaller particles. Diffusion is dominant for particles smaller than 100 nm, especially for smaller ultrafine particles. After the in-cabin air velocity is increased, it is likely to produced more turbulent air flow intensifying the turbulent diffusion, which leads to a greater probability for the particles to hit and deposit onto surfaces. Similarly, CitationLai et al. (2002) found that particle deposition rates increased with increased indoor air speeds in a test chamber. illustrates the relative ratio of particle deposition rates for 30, 66.1, and 101.8 nm particles under two air flow conditions (V3 = 0.65 m s–1 and V0 = natural convection) inside three test vehicles, which explicitly indicate that increasing the in-cabin air velocity has a greater effect on the particle deposition rate for smaller particles compared with larger ones. Quantitatively, for example, the ratio is 1.58, 1.27, and 1.16 for three chosen particle sizes inside the Ford F-250 Super Duty truck, respectively. It is noted that although the high vehicle speed leads to high in-cabin air velocity, which increases the particle deposition rates inside the vehicle, the high vehicle speed also increases air exchange rates which contribute to high in-cabin ultrafine particle levels by bringing more particles from outside into in-cabin microenvironments. Hence, high particle deposition rates do not necessary lead to low in-cabin exposure levels, because particle concentrations inside the vehicle are affected by many other influencing factors besides deposition rate, such as penetration factor, in-cabin filter filtration efficiency, and vehicle speed (CitationXu and Zhu 2009). When the ventilation setting is fan off/RC off, in-cabin particle concentrations are mainly affected by penetration factor and deposition rate. When the ventilation setting is fan on/RC off, the airflow rate of mechanical ventilation is the predominant influencing factor. When the ventilation setting is fan on/RC on, the effects of penetration factor, deposition rate, and vehicle speed are comparable (CitationXu and Zhu 2009).
The Effect of the In-Cabin Surface Area on Particle Deposition Rates
The effect of vehicle interior surface areas on particle deposition rates is presented in . During the experiments, internal surface areas were increased by using mannequins wearing T-shirts and blue jeans to simulate different number of passengers inside the vehicle. Four in-cabin conditions (none, one, two, and three passengers inside test vehicles) were investigated. The experiments were conducted under natural convection (< 0.02 m s–1). From , increases can be seen in particle deposition rates with increased surface areas or more passengers for all the vehicles, which is consistent with previous indoor studies. For example, CitationThatcher et al. (2002) have reported that increases in the amount of furnishing resulted in greater deposition rates. Although the surface area for the three-passenger condition was only about 30% larger than for the no-passenger condition, additional surface areas attributable to fibers and textures and changed air flow patterns by people inside the vehicle were not included, all of which may have contributed to the increased deposition rates.
The Effect of the Surface Area to Volume Ratio and Other Influencing Factors on Particle Deposition Rates
The Isuzu Rodeo SUV and the Saturn SL2 car possess similar interior surface materials. Particle deposition rates as a function of surface area to volume ratio for 30, 66.1, and 101.8 nm particles inside these two test vehicles are shown in . Symbols and solid lines represent collected data. Results from the figure suggest that the surface area to volume ratio may be an important factor influencing ultrafine particle deposition rates. There is a positive correlation between surface area to volume ratios and ultrafine particle deposition rates. Greater surface area to volume ratio results in higher deposition rate for ultrafine particles. The particles deposition rates inside the SUV, which has the smallest S/V ratio among three test vehicles, are lower than those inside the Saturn SL2 car.
FIG. 7 The particle deposition rates as a function of surface area to volume ratios for 30, 66.1, and 101.8 nm particles inside two test vehicles.
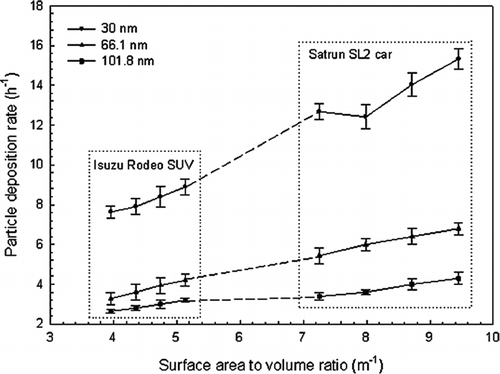
From where the mean particle deposition rates under four in-cabin air conditions were plotted as a function of particle size inside three test vehicles, it can be seen that the highest deposition rates occurred inside the Saturn SL2 car, although the Ford F-250 Super Duty truck has the greatest surface area to volume ratio among three test vehicles. Surface roughness may be the primary contributor to this observation. The surface materials of seats inside the Saturn SL2 car are cotton and sponge, much rougher than artificial leather-made seat covers inside the Ford F-250 Super Duty truck. The car's age may also play a role. The Saturn SL2 car is the oldest vehicle among three test vehicles, hence prossessing most contaminated interior surfaces, which can lead to greater surface roughness (CitationHinds 1999). Many previous indoor studies have reported increases in deposition rates with increased surface roughness (CitationAbadie et al. 2001; CitationLai et al. 2002; CitationAfshari and Reinhold 2008). Increased protrusion elements due to greater surface roughness can reduce the thickness of the particle concentration boundary layer, which enhances the particle concentration gradient near the wall according to the Fick's first law of diffusion. Equivalently, it reduces the particle transfer resistance to the wall surfaces, which serves as the dominant influencing factor on particle deposition (CitationZhao and Wu 2007). As a result, the turbulent diffusion is intensified due to the increased particle concentration gradient, and then particles are more efficiently removed due to the greater turbulent diffusion (CitationNomura et al. 1997).
Total Particle Deposition Rates Inside Test Vehicles
The in-cabin total particle number concentrations during the experiments also were measured by only using a condensation particle counter (CPC, TSI Model 3785) inside three test vehicles following the same experimental protocol. The results were relatively consistent from test to test. In general, under the typical in-cabin microenvironment with ultrafine particle number concentrations of approximately 105 cm–3 on or near roadways (CitationZhu et al. 2002; CitationZhu and Hinds 2005; CitationZhu et al. 2007), if there is no obvious in-cabin particle source, after about 160 minutes, 90% of in-cabin ultrafine particles are estimated to deposit onto the interior surfaces. This suggests that the particle deposition is a significant factor for in-cabin ultrafine particle concentration decay and needs to be considered when assessing their exposure and associated health effects.
Comparison with Indoor Studies
A number of previous studies have reported the particle deposition rates indoors, most of which focused on fine and coarse particle size range. CitationLai (2002) has compiled data from 15 major studies completed in experimental chambers and test houses. It is found that there is a large variability in particle deposition rates attributable to different experimental designs, particle types, measuring instruments, calculation methods, and other properties of the deposition environment, such as surface roughness, all of which can tremendously affect the particle deposition rates indoors. The large amount of variability observed verifies considerable difficulties in separating deposition from many other competing factors. Here, some pertinent data in the ultrafine particle size range were selected to compare with in-cabin results from this study. summarizes experimental conditions of selected indoor studies. compares ultrafine particle deposition rates from the current work with indoor studies summarized in and an indoor model. The mean particle deposition rates as a function of particle size inside three test vehicles under all experimental conditions investigated in this study were included in the figure for comparison. In general, all experimental data and the model follow a similar trend. However, there is a considerably large degree of variability in particle deposition rates for all the particle sizes from study to study. For example, for 35 nm particles, experimental data vary by a factor of 33.7, from 0.32 to 10.79 h–1. Different test locations, particle sources or types, and interior volume may contribute to the differences in particle deposition rates among selected studies and the present study. The in-cabin ultrafine particle deposition rates measured in the present work are consistently higher than those measured and modeled in the indoor environments. This is likely due to the higher in-cabin air velocity inside vehicles and the larger surface area to volume ratio existing in the in-cabin microenvironment. Compared with houses and chambers in previous indoor studies, the in-cabin microenvironment has much smaller space, shown in the last column in . Besides, the discrepancy between experimental data and modeling work is outstanding. In general, the model estimates, especially for ultrafine particles, are much smaller than those of the experimental results mainly owing to the assumption of smooth surfaces in the model which maybe cause limitations (CitationLai and Nazaroff 2000).
TABLE 2 A summary of experimental conditions of indoor particle deposition studies compared in
CONCLUSION
The present study has advanced our understanding of ultrafine particles deposition in in-cabin microenvironments where no deposition experiments were intentionally conducted before. Average ultrafine particle deposition rates, in the range of 3.2–11.8 h–1 for different particle sizes, were observed in the present in-cabin study. Deposition rates as a function of particle size found in this study are about 3 to 20 times greater than those reported in previous indoor studies. This is probably due to the larger surface area to volume ratio and higher in-cabin air velocities inside vehicles. This study also demonstrated the strong relationship between the deposition rates and the in-cabin air velocity and surface area. As the in-cabin air velocity increased from natural convection (< 0.02 m s–1) to 0.65 m s–1, ultrafine particle deposition rates increased with the largest increases occurred for smaller particles due to greater diffusional deposition. Increasing number of passengers inside the vehicle increased the in-cabin surface areas as well as the deposition rates. The interior surface roughness and car's age also should be considered as influencing factors. The particle deposition process can significantly affect in-cabin particle concentrations. Therefore, it needs to be taken into account when assessing their exposures and associated human health effects.
Acknowledgments
This material is based upon work partially supported by the Center of Research Excellence in Science and Technology—Research on Environmental Sustainability of Semi-Arid Coastal Areas (CREST-RESSACA) at Texas A&M University—Kingsville through a Cooperative Agreement (No. HRD-0734850) from the National Science Foundation. Any opinions, findings, and conclusions or recommendations expressed in this material are those of the authors and do not necessarily reflect the views of the National Science Foundation. Bin Xu would like to thank China Scholarship Council for his scholarship under the State Scholarship Fund. The authors also gratefully appreciated the assistance from Neil Friedberg, Qunfang Zhang, and Robert Salinas.
REFERENCES
- Abadie , M. , Limam , K. and Allard , F. 2001 . Indoor Particle Pollution: Effect of Wall Textures on Particle Deposition . Building and Environ. , 36 : 821 – 827 .
- Abt , E. , Suh , H. , Catalano , P. and Koutrakis , P. 2000 . Relative Contribution of Outdoor and Indoor Particle Sources to Indoor Concentrations . Environ. Sci. Technol. , 34 : 3579 – 3587 .
- Afshari , A. and Reinhold , C. 2008 . Deposition of Fine and Ultrafine Particles on Indoor Surface Materials . Indoor Built Environ. , 17 : 247 – 251 .
- Beckett , W. S. 2002 . Inhaled Particle Characteristics and Early Lung Effects , Crisp Data Base National Institutes of Health .
- Byrne , M. A. , Goddard , A. J. H. , Lange , C. and Roed , J. 1995 . Stable Tracer Aerosol Deposition Measurements in a Test Chamber . J. Aerosol Sci. , 26 : 645 – 653 .
- Cheng , Y. S. 1997 . Wall Deposition of Radon Progeny and Particles in a Spherical Chamber . Aerosol Sci. Technol. , 27 : 131 – 146 .
- Crump , J. G. and Seinfeld , J. H. 1981 . Turbulent Deposition and Gravitational Sedimentation of an Aerosol in a Vessel of Arbitrary Shape . J. Aerosol Sci. , 12 : 405 – 415 .
- Davis , F. A. 1989 . Tabler's Cyclopedic Medical Dictionary , Philadelphia, PA : FA Davis Company .
- Dockery , D. W. 2001 . Epidemiologic Evidence of Cardiovascular Effects of Particulate Air Pollution . Environmental Health Perspectives , 109 : 483 – 486 .
- Donaldson , K. , Brown , D. , Clouter , A. , Duffin , R. , MacNee , W. , Renwick , L. , Tran , L. and Stone , V. 2002 . The Pulmonary Toxicology of Ultrafine Particles . J. Aerosol Med. , 15 : 213 – 220 .
- Fogh , C. L. , Byrne , M. A. , Roed , J. and Goddard , A. J. H. 1997 . Size Specific Indoor Aerosol Deposition Measurements and Derived I/O Concentrations Ratios . Atmos. Environ. , 31 : 2193 – 2203 .
- Fruin , S. , Westerdahl , D. , Sax , T. , Sioutas , C. and Fine , P. M. 2008 . Measurements and Predictors of On-road Ultrafine Particle Concentrations and Associated Pollutants in Los Angeles . Atmos. Environ. , 42 : 207 – 219 .
- He , C. , Morawska , L. and Gilbert , D. 2005 . Particle Deposition Rates in Residential Houses . Atmos. Environ. , 39 : 3891 – 3899 .
- Hinds , W. C. 1999 . Aerosol Technology: Properties, Behavior, and Measurement of Airborne Particles , New York : John Wiley & Sons .
- Howard-Reed , C. , Wallace , L. A. and Emmerich , S. J. 2003 . Effect of Ventilation Systems and Air Filters on Decay Rates of Particles Produced by Indoor Sources in an Occupied Townhouse . Atmos. Environ. , 37 : 5295 – 5306 .
- Kittelson , D. B. 1998 . Engines and Nanoparticles: A Review . J. Aerosol Sci. , 29 : 575 – 588 .
- Kleinman , M. T. , Araujo , J. A. , Nel , A. , Sioutas , C. , Campbell , A. , Cong , P. Q. , Li , H. and Bondy , S. C. 2008 . Inhaled Ultrafine Particulate Matter Affects CNS Inflammatory Processes and May Act via MAP Kinase Signaling Pathways . Toxicology Letters , 178 : 127 – 130 .
- Klepeis , N. E. , Nelson , W. C. , Ott , W. R. , Robinson , J. P. , Tsang , A. M. , Switzer , P. , Behar , J. V. , Hern , S. C. and Engelmann , W. H. 2001 . The National Human Activity Pattern Survey (NHAPS): A Resource for Assessing Exposure to Environmental Pollutants . J. Expos. Anal. Environ. Epidemiol. , 11 : 231 – 252 .
- Lai , A. C. K. and Nazaroff , W. W. 2000 . Modeling Indoor Particle Deposition from Turbulent Flow onto Smooth Surfaces . J. Aerosol Sci. , 31 : 463 – 476 .
- Lai , A. C. K. , Byrne , M. A. and Goddard , A. J. H. 2002 . Experimental Studies of the Effect of Rough Surfaces and Air Speed on Aerosol Deposition in a Test Chamber . Aerosol Sci. Technol. , 36 : 973 – 982 .
- Lai , A. C. K. and Nazaroff , W. W. 2005 . Supermicron Particle Deposition from Turbulent Chamber Flow onto Smooth and Rough Vertical Surfaces . Atmos. Environ. , 39 : 4893 – 4900 .
- Lai , A. C. K. 2006 . Particle Deposition and Decay in a Chamber and the Implications to Exposure Assessment . Water, Air, and Soil Pollution , 175 : 323 – 334 .
- Long , C. M. , Suh , H. H. , Catalano , P. J. and Koutrakis , P. 2001 . Using Time- and Size-Resolved Particulate Data to Quantify Indoor Penetration and Deposition Behavior . Environ. Sci. Technol. , 35 : 2089 – 2099 .
- MacNee , W. and Donaldson , K. 2003 . Mechanism of Lung Injury Caused by PM10 and Ultrafine Particles with Special Reference to COPD . European Respiratory Journal , : 21
- Matthews , T. G. , Thompson , C. V. , Wilson , D. L. and Hawthorne , A. R. 1989 . Air Velocities inside Domestic Environments: An Important Parameter in the Study of Indoor Air Quality and Climate . Environ. Inter. , 15 : 545 – 550 .
- Monn , C. 2001 . Exposure Assessment of Air Pollutants: A Review on Spatial Heterogeneity and Indoor/Outdoor/Personal Exposure to Suspended Particulate Matter, Nitrogen Dioxide and Ozone . Atmos. Environ. , 35 : 1 – 32 .
- Morawska , L. and Jamriska , M. 1996 . Deposition of Radon Progeny on Indoor Surfaces . J. Aerosol Sci. , 27 : 305 – 312 .
- Mosley , R. B. , Greenwell , D. J. , Sparks , L. E. , Guo , Z. , Tucker , W. G. , Fortmann , R. and Whitfield , C. 2001 . Penetration of Ambient Fine Particles into the Indoor Environment . Aerosol Sci. Technol. , 34 : 127 – 136 .
- Nazaroff , W. W. , Gadgil , A. J. and Weschler , C. J. 1993 . “ Critique of the Use of Deposition Velocity in Modeling Indoor Air Quality ” . In In Modeling Indoor Air Quality and Exposure , ASTM STP 1205 Edited by: Nagda , N. L. 81 – 104 . Philadelphia, PA : American Society for Testing and Materials .
- Nel , A. , Xia , T. , Mädler , L. and Li , N. 2006 . Toxic Potential of Materials at the Nanolevel . Science , 311 : 622 – 627 .
- Nemmar , A. and Inuwa , I. M. 2008 . Diesel Exhaust Particles in Blood Trigger Systemic and Pulmonary Morphological Alterations . Toxicology Letters , 176 : 20 – 30 .
- Nomura , Y. , Hopke , P. K. , Fitzgerald , B. and Mesbah , B. 1997 . Deposition of Particles in a Chamber as a Function of Ventilation Rate . Aerosol Sci. Technol. , 7 : 62 – 72 .
- Ott , W. , Klepeis , N. and Switzer , P. 2007 . Air Change Rates of Motor Vehicles and In-Vehicle Pollutant Concentrations from Secondhand Smoke . J. Expos. Sci. Environ. Epidemiol. , 4 : 1 – 14 .
- Pope , C. A. , Burnett , R. T. , Thun , M. J. , Calle , E. E. , Krewski , D. , Ito , K. and Thurston , G. D. 2002 . Lung Cancer, Cardiopulmonary Mortality, and Long-Term Exposure to Fine Particulate Air Pollution . J. American Med. Assoc. , 287 : 1132 – 1141 .
- Pope , C. A. and Dockery , D. W. 2006 . Health Effects of the Fine Particulate Air Pollution: Lines that Connect . J. Air & Waste Manage. Assoc. , 56 : 709 – 774 .
- Riley , W. J. , McKone , T. E. , Lai , A. C. K. and Nazaroff , W. W. 2002 . Indoor Particulate Matter of Outdoor Origin: Importance of Size-Dependent Removal Mechanisms . Environ. Sci. Technol. , 36 : 200 – 207 .
- Thatcher , T. L. and Layton , D. W. 1995 . Deposition, Rresuspension, and Penetration of Particles within a Residence . Atmos. Environ. , 29 : 1487 – 1497 .
- Thatcher , T. L. , Lai , A. C. K. , Moreno-Jackson , R. , Sextro , R. G. and Nazaroff , W. W. 2002 . Effects of Room Furnishings and Air Speed on Particle Deposition Rates Indoors . Atmos. Environ. , 36 : 1811 – 1819 .
- Vette , A. F. , Rea , A. W. , Lawless , P. A. , Rodes , C. E. , Evans , G. , Highsmith , V. R. and Sheldon , L. 2001 . Characterization of Indoor-Outdoor Aerosol Concentration Relationships during the Fresno PM Exposure Studies . Aerosol Sci. Technol. , 34 : 118 – 126 .
- Wallace , L. 1996 . Indoor Particles: A Review . J. Air & Waste Manage. Assoc. , 46 : 98 – 126 .
- Wallace , L. A. , Emmerich , S. J. and Howard-Reed , C. 2004a . Source Strengths of Ultrafine and Fine Particles due to Cooking with a Gas Stove . Environ. Sci. Technol. , 38 : 2304 – 2311 .
- Wallace , L. A. , Emmerich , S. J. and Howard-Reed , C. 2004b . Effect of Central Fans and In-Duct Filters on Deposition Rates of Ultrafine and Fine Particles in an Occupied Townhouse . Atmos. Environ. , 38 : 405 – 413 .
- Xia , T. , Korge , P. , Weiss , J. N. , Li , N. , Venkatesen , I. , Sioutas , C. and Nel , A. 2004 . Quinones and Sromatic Chemical Compounds in Particulate Matter (PM) Induce Mitochondrial Dysfunction: Implications for PM-Induced Oxidative Stress and Toxicity . Environ. Health Perspect. , 112 : 13471358
- Xu , B. and Zhu , Y. 2009 . Quantitative Analysis of the Parameters Affecting In-Cabin to On-Roadway (I/O) Ultrafine Particle Concentration Ratios . Aerosol Sci. Technol. , 43 ( 5 ) In press
- Xu , M. D. , Nematollahi , M. , Sextro , R. G. , Gadgil , A. J. and Nazaroff , W. W. 1994 . Deposition of Tobacco Smoke Particles in Low Ventilation Room . Aerosol Sci. Technol. , 20 : 194 – 206 .
- Zhao , B. and Wu , J. 2007 . Particle Deposition in Indoor Environments: Analysis of Influencing Factors . J. Hazardous Materials , 147 : 439 – 448 .
- Zhu , Y. , Hinds , W. C. , Kim , S. and Sioutas , C. 2002 . Concentration and Size Distribution of Ultrafine Particles Near a Major Highway . J. Air & Waste Manage. Assoc. , 52 : 1032 – 1042 .
- Zhu , Y. and Hinds , W. C. 2005 . Predicting Particle Number Concentrations near a Highway Based on Vertical Concentration Profile . Atmos. Environ. , 39 : 1557 – 1566 .
- Zhu , Y. , Hinds , W. C. , Krudysz , M. , Kuhn , T. , Froines , J. and Sioutas , C. 2005 . Penetration of Freeway Ultrafine Particles into Indoor Environments . J. Aerosol Sci. , 36 : 303 – 322 .
- Zhu , Y. , Eiguren-Fernandez , A. , Hinds , W. C. and Miguel , A. H. 2007 . In-Cabin Commuter Exposure to Ultrafine Particles on Los Angeles Freeways . Environ. Sci. Technol. , 47 : 2138 – 2145 .