Abstract
A new instrument, namely the 1 × 3 tandem differential mobility analyzer (1 × 3-TDMA), has been developed. Its primary measurement is the irreversibility of the hygroscopic growth factor of aerosol particles. The instrument uses the hysteresis of phase transitions to infer the solid or aqueous state of the particles. A first DMA passes particles of a selected electric mobility at relative humidity RH0. Exiting this DMA, the particles are split into three separate flows. The first flow is exposed to RH 0 → (RH 0 + δ ) → RH 0 in a deliquescence test before passing through a second DMA that is set to the same electric mobility as the first DMA. The second flow passes directly to a third DMA without change in RH, thereby serving as a reference arm. This DMA is also set to the same electric mobility as the first DMA. The transmission ratio of the 1 × 3-TDMA is defined as the particle concentration passing the deliquescence test divided by that passing through the reference arm. The transmission ratio is unity in the absence of deliquescence and zero when a phase transition occurs, at least for ideal instrument performance in application to a test aerosol of fully deliquesceable particles. For the third flow passing out of the first DMA, an efflorescence test is run by using the RH profile of RH 0 → (RH 0 − δ ) → RH 0 before passing through a fourth DMA. A full data set for the 1 × 3-TDMA is obtained by scanning RH0, typically from 20 to 85%. In the present paper, the 1 × 3-TDMA instrument is described, and laboratory data are presented for the phase transitions of externally mixed aerosols of aqueous and solid sodium chloride particles, aqueous and solid ammonium sulfate particles, and their mixtures, as well as a mixture of aqueous and solid sea salt particles. The observed transmission ratio is compared to a model analysis. The intent behind the development of this instrument is to deploy it for field measurements and use observations of the irreversibility of the growth factors of atmospheric particles as markers of their physical state.
1. INTRODUCTION
The radiation balance of Earth's atmosphere is strongly influenced by aerosol particles (CitationSeinfeld and Pandis 2006). The magnitude of this radiative forcing depends on the light scattering and absorption properties of the particles, which vary with factors such as size, composition, and physical state (CitationKinne et al. 2006). On a global basis sulfate is the largest anthropogenic contribution to the mass budget of atmospheric particles and consequently constitutes a significant cooling term to Earth's radiative energy balance (CitationSolomon et al. 2007). The fluctuation of atmospheric particles between aqueous and solid states, which is a very strong regulator of aerosol light scattering, is estimated to affect the annual-average global direct radiative forcing of sulfate particles by up to as much as 24% (CitationMartin et al. 2004; CitationWang et al. 2008 a, b).
The effect on light scattering mainly arises from the larger diameter of an aqueous particle compared to its solid counterpart (CitationHegg et al. 1993), as quantified by the hygroscopic growth factor (CitationGysel et al. 2002). Discontinuous, irreversible jumps in an individual particle's diameter occur at the transition from a solid to aqueous state (deliquescence) and vice versa (efflorescence), mostly from the incorporation and the rejection, respectively, of water molecules from the particle. The growth factor depends not only on the state variables of particle chemical composition and aerosol relative humidity (RH) but also, because of a hysteresis effect, on the recent RH history. For example, because solid sodium chloride particles deliquesce at 75% RH at 298 K and aqueous sodium chloride particles effloresce at 45% RH (CitationMartin 2000), sodium chloride particles may exist as either aqueous or solid particles over the domain 45 < RH < 75%, with the implication that the hygroscopic growth factor is not a single-valued function over this range. For ammonium sulfate, the similar range of hysteresis is 35 < RH < 80%. Hysteresis occurs because aqueous particles do not immediately crystallize when saturation is reached but rather remain metastable until the aqueous-phase supersaturation is sufficiently high such that homogeneous nucleation of the crystal is rapid (CitationBiskos et al. 2006a).
One approach for studying the phase transitions of atmospheric particles is the hygroscopic-growth tandem differential mobility analyzer (HTDMA). As three examples, CitationPitchford and McMurry (1994) studied deliquescence transitions of ambient particles in the Grand Canyon, CitationSantarpia et al. (2004) studied both deliquescence and efflorescence transitions in southeast Texas, and CitationKhlystov et al. (2005) studied the particle water content (thus inferring aqueous or solid state) in Pittsburgh. The design of the HTDMA, however, is optimized for primary measurements of hygroscopic growth factors (CitationSwietlicki et al. 2008), from which information about particle phase and phase transitions can be inferred as a derived quantity (CitationPitchford and McMurry 1994; CitationSantarpia et al. 2004; CitationKhlystov et al. 2005). A purpose-designed instrument to study the phase transitions of ambient particles has not been deployed previously, and such an instrument could be expected to provide more sensitivity, new statistics, and further insights into the phase transitions of ambient particles. A novel instrument is therefore introduced in the present study, namely the 1 × 3 tandem differential mobility analyzer (1 × 3-TDMA). The 1 × 3-TDMA exploits the hysteresis effect to infer the physical state of aerosol particles through a test of the reversibility of the hygroscopic growth factor.
A diagram of the approach employed by the 1 × 3-TDMA is shown in . At the inlet, aerosol particles are first charged and subsequently equilibrated to a set relative humidity RH0. A first differential mobility analyzer (DMA mono ) selects aerosol particles having a narrow band of electric mobility. The aerosol flow is next split into three parallel arms, namely a “reference” (denoted 0), a “deliquescence test” (denoted +δ), and an “efflorescence test” (denoted −δ). In the two test arms, the aerosol particles are subjected to an RH history that perturbs them by a small RH change (RH0 ± |δ|) before returning them to the previous RH0. The specific RH histories imposed in each arm are RH0 → (RH0+ |δ|) → RH0 for δ> 0 as a deliquescence test (e.g., 72% → 77% → 72% RH), RH0 → (RH0−|δ|) → RH0 for δ< 0 as an efflorescence test (e.g., 72% → 67% → 72% RH), and RH0→ RH0 → RH0 as a reference condition (e.g., 72% → 72% → 72% RH). The flows in the three arms next pass through separate DMAs tuned to the same electric mobility as DMA mono . Three condensation particle counters (CPCs) quantify the particle number concentration passing each of these DMAs. Irreversible changes in particle diameter and hence electric mobility in the two test arms are detected as reductions in the number concentrations in these arms compared to the reference arm. Specifically, the transmission ratio of the deliquescence test is calculated as the number concentration of CPC+δ to that of CPC0. The ratio of the number concentration of CPC−δ to that of CPC0 provides a similar definition of the transmission ratio for the efflorescence test.
FIG. 1 Diagram of the 1-by-3 tandem differential mobility analyzer (1 × 3-TDMA) for measurement of the irreversibility of the hygroscopic growth factor. The principles of the 1 × 3-TDMA operation are described in the text. Legend: RH0, adjustment of aerosol relative humidity to a central value; RH+δ, adjustment of aerosol relative humidity to RH0+ |δ|; RH−δ, adjustment of aerosol relative humidity to RH0−|δ|; DMA, differential mobility analyzer (all tuned to the same electric mobility); CPC, condensation particle counter.
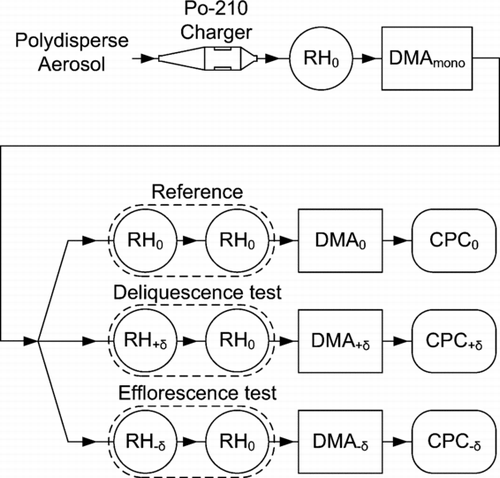
The expected response of the 1 × 3-TDMA can be compared to that of an HTDMA. The considered test aerosol has three externally mixed particle types, including ammonium nitrate of DRH = 60%, sodium chloride of DRH = 75%, and ammonium sulfate of DRH = 80%, where DRH is the deliquescence relative humidity. Under ideal conditions of constant temperature and relative humidity, a perfectly functioning HTDMA for increasing RH would observe step increases in diameter at 60, 75, and 80% RH. For more typical conditions, the HTDMA would observe an apparent smooth hygroscopic growth curve for underlying stepwise deliquescence. This test aerosol represents some of the complications of external mixing of real atmospheric particles, and this complexity can confound efforts to detect phase transitions by an HTDMA in field measurements. In comparison, for the same test aerosol the 1 × 3-TDMA would have improved performance for detection of the irreversible changes in particle diameter.
The purpose of the present report is to provide a description of the design and operation of the 1 × 3-TDMA under laboratory conditions. The intent behind the development of this instrument is deployment in the field for measurements of the irreversibility of the growth factor of atmospheric particles and to use these measurements as markers of their physical state. A companion report describes observations and findings during instrument deployment in the Southern Great Plains, Oklahoma, USA, in June 2007 (CitationMartin et al. 2008).
2. INSTRUMENT DESCRIPTION
A diagram highlighting the essential features of the 1 × 3-TDMA is shown in . Other technical diagrams are provided in Figures S1 and S2 (see Appendix). The differential mobility analyzers are described in Section 2.1. Humidity generation and control are presented in Section 2.2. Methods for aerosol generation for the laboratory studies are communicated in Section 2.3. These subsystems are integrated using Labview to allow computer-controlled instrument operation and data acquisition, including the flow rates, the relative humidities, the DMA voltages, and the CPC counts.
The entire assembly is constructed of two racks, which are moveable on wheels. One rack holds the electronics and another contains the humidification systems, the DMAs, and the CPCs. This second rack is enclosed in an acrylic box. Fans drawing air from the laboratory circulate air continuously within the box to minimize temperature differences (<0.5 K) among the various components while maintaining the apparatus at ambient temperature.
2.1. Differential Mobility Analyzers
Each of the four DMAs () is built from a TSI column (model 3081). The rod voltage of each is driven by a Glassman High Voltage power supply (model PS/EH10R10.0YH3) and is regulated by an interface to LabView. An open-loop system is used for the sheath air. For this purpose, each DMA is outfitted with a blower, a particle filter, a laminar flow element with a pressure-drop sensor, a heat exchanger, and an RH monitor. The in-flow of RH-controlled sheath air for each DMA is adjusted by the use of blowers to match the “excess out” flow. The out-flow of the sheath air is fixed by a critical orifice (10 or 20 L min–1) attached to the “excess out” port of each DMA. Figures S1 and S2 show details of this setup. Flows are calibrated and periodically checked with a primary flow standard (Gilibrator-2, Sensidyne, Inc.). DMA mono is operated at an aerosol flow of 3 L min–1 and a sheath flow of 20 L min–1. The downstream DMAs operate at aerosol and sheath flows of 1 and 10 L min–1, respectively. Particle concentrations passing DMA0, DMA+δ, and DMA−δ are measured using condensation particle counters (CPCs; model 3010, TSI Inc). Inside the CPCs are critical orifices that regulate the “aerosol out” flow to approximately 1 L min–1. The “aerosol in” flow is therefore indirectly but nevertheless precisely regulated as the balance flow to “sheath in,” “aerosol out,” and “excess out.” Prior to entering DMA mono , particles are charged using polonium-210 (Aerosol Dynamics Inc., USA). For the experiments described in this paper, the setpoint electric mobility corresponds to a mobility diameter of 100 nm (one-charge equivalent).
For the sheath-air system of each DMA, a sealed compressive blower (model SE12RE21S, part no. 081340, Ametek; model XP32-12/24 DC, Minarik, USA) produces pulsation-free air flow and greater than 3 kPa at 10 L min–1. A high-efficiency particle filter (HEPA; part no. 6702–7500, Whatman, USA) is also employed. This filter has a hydrophobic glass microfiber media, which minimizes water storage and release and thus has little effect on the time constant for equilibration to changes in RH0. A pressure drop across a laminar flow element (LFE, models FCO96E-30L and FCO96E-20L, Furness Controls, UK) responds linearly to the volumetric flow rate (0–30 L min–1 for DMA mono and 0–20 L min–1 for downstream DMAs). The measured pressure drop provides a proportional voltage signal fed to a proportional-integral-differential (PID) process controller (model CNi16D52-C24-DC, Omega, USA), thereby providing a control voltage to the DC speed drive of the compressor. A stainless-steel, finned-tube heat exchanger (model 4105G1SB, Lytron, USA) is used to remove the excess heat generated by the compressive blower. Relative humidity and temperature are monitored (Hygroclip S, Rotronic, Switzerland) prior to the sheath flow entry into the classifier column.
2.2. Humidity Generation and Control
Three humid flows (>95% RH) are generated by bubbling particle-free air through stainless-steel sintered diffusers (0.5 μm, St. Patrick's of Texas) submersed in deionized water (18 MΩ cm) in temperature-controlled vessels (Figure S2). Silicon heaters (7.62 cm × 45.72 cm, 0.388 W cm–2, McMaster-Carr) adhered to the outside of the vessels, in conjunction with submersed thermocouples (type K) to measure the water temperature and a process controller (model CNi3243, Omega, USA) to provide feedback, maintain a steady water temperature of 300 ± 0.1 K. The humid flows are proportionately mixed with dry flows (<5% RH) using mass flow controllers (MFCs) and a Labview control program to generate flows RH0, RH+δ, and RH-δ. Two 100 L min–1 MFCs (MKS model 1559A-100L-SV using a MKS Type 647C controller) regulate the flows for adjusting RH0, and two pairs of 10 L min–1 MFCs (MKS model M100B01314CS1BV) are employed for the RH+δ and RH−δ flows. For the usual mode of instrument operation, the requirement is 82 L min–1 of RH0 flow, 4.5 L min–1 of RH+δ flow, and 4.5 L min–1 of RH-δ flow. The RH of each flow is measured and used for feedback control to within an accuracy of ±1% RH using Hygroclip S sensors (Rotronic, Switzerland). The RH sensors are calibrated using saturated salt solutions of LiCl, MgCl2, Mg(NO3)2, NaCl, and KCl, and they are routinely tested against a dew point mirror hygrometer.
These flows are used to drive the seven conditioners shown in and S1. The humidity conditioner prior to DMA mono consists of two Nafion tube bundles (PD50T-12SS, Perma Pure). By passing an aerosol flow on one side of the Nafion membrane and a drive flow on the other side of the membrane, the RH of the aerosol can be fully adjusted to the RH of the drive flow provided that the residence time is sufficient for mass transfer. The estimated residence time of the aerosol flow in the humidity conditioners is less than 1 s for the flow rates employed. The drive flow is 7 L min–1 in each of the two bundles, and the aerosol flow is passed through them in series at 3 L min–1. The drive flows run countercurrent inside the Nafion tubes, and the aerosol flow runs outside of the bundle. The drive flow is regulated by a rotameter (model RMA-22-TMV, Dwyer Inst.) connected to a vacuum pump.
Each of the six humidity conditioners downstream of DMA mono consists of a single Nafion tube enclosed in a 1/4″ (outside diameter) stainless steel tube (Perma Pure MD-110C5-96S-4). The drive and aerosol flows are 4.5 L min–1 and 1 L min–1, respectively. The drive flow, regulated by a rotameter (RMA-26-TMV, Dwyer Inst.), runs outside the Nafion tube and countercurrent to the aerosol flow.
2.3. Aerosol Generation
Externally mixed test aerosols having variable fractions of aqueous and solid particles are generated using two atomizers (TSI 3076) (Figure S2). Restricting orifices after the atomizers are adjusted to 1 L min–1, and excess flow ports (equipped with HEPA cartridge filters for clean-air venting into the laboratory) ensure nearly atmospheric pressure exiting the atomizer. Downstream of the restricting orifices, ejector pumps (model AV147L, Air-Vac Engineering Co.) mix, dilute, and increase the aerosol flow. They also enhance the initial evaporation of water molecules from the particles and provide the pressure drop for the functioning of the restricting orifices. Solid or aqueous particles are obtained by passing the flows through a Nafion humidity conditioner set to RH > DRH for aqueous particles or through a silica gel diffusion dryer (2 m long, 1/2″ flow diameter, RH < 10% at exit) for dry particles. Pinch valves, in conjunction with additional excess flow ports, are used to control the relative flow and thereby adjust the ratio of solid to aqueous particles. The two flows are brought together in a final mixing chamber, which is a glass jar in which the particles have a typical residence time of 1 min. The jar contains a saturated salt solution of Mg(NO3)2 (53% RH) to equilibrate the aerosol to an RH between the efflorescence and deliquescence relative humidities and thus stabilize the population for analysis.
For testing of the 1×3-TDMA, we prepared several different test aerosols. Solid and aqueous particles of sodium chloride (NaCl) (Mallinckrodt Chemicals product number 7581, ACS grade, min 99.0% pure), ammonium sulfate (AS) (Sigma Aldrich product number A4915, ACS grade min 99.0% pure), and sea salt (Sigma Aldrich product number S9883) were examined. Although elemental analysis of the particular sea salt employed in these studies is not available, sea salt in general consists of 48.7% Cl−, 41.9% Na+, 4.7% Mg2+, 2.5% SO2− 4, 0.9% Ca2+, 0.9% K+ on a mole basis, accounting for 99.7% of the constituents (CitationStumm and Morgan 1996). NaCl and AS particles are widely used for calibration of growth factors measured by HTDMAs (CitationChan and Chan 2005; CitationMcFiggans et al. 2006). The DRH of NaCl is 75% and of AS is 79.5% at 298 K for bulk materials (CitationMartin 2000). The respective efflorescence relative humidities (ERHs) are 45 ± 2% and 35 ± 2%. Test aerosols included: (Equation1) a 80:20 mix of aqueous and solid NaCl particles for the results shown in , (Equation2) a 50:50 mix of solid and aqueous AS particles for , (Equation3) a 15:85 mix of solid and aqueous NaCl particles for , (4) a 20:10:40:20 mix of AS(aq):AS(s):NaCl(aq):NaCl(s) particles for (by further extension of the apparatus for aerosol generation shown in Figure S2), and () a 50:50 mix of low-RH (exposed to RH < 5%) and high-RH (exposed to RH > 95%) sea salt particles for . Particle concentrations in the test arms varied between 500 and 2500 particles cm–3 for the different test aerosols.
FIG. 2 (top) Theoretical hygroscopic growth curve of 100 nm sodium chloride particles modeled for a conventional HTDMA (CitationBiskos et al. 2006a). Left and right panels represent deliquescence and efflorescence scans for particles initialized in solid and aqueous particles, respectively. Arrows indicate the direction of the RH scan. (bottom) Theoretical 1 × 3-TDMA response (|δ|= 5), which is shown as the particle transmission ratio for the similar RH scans as the top row. The transmission ratio is defined as (left) the concentration count from CPC+δ divided by that of CPC0 for the deliquescence test and (right) the concentration count from CPC−δ divided by that of CPC0 for the efflorescence test. For the deliquescence test, the transmission ratio drops below unity at RH0 values for which a perturbation of +|δ| yields an irreversible change in the growth factor of solid particles (i.e., DRH – |δ|< RH0< DRH). Efflorescence likewise induces an irreversible response in the growth factor for aqueous particles for a perturbation of -|δ| over the range ERH < RH0< ERH + |δ|.
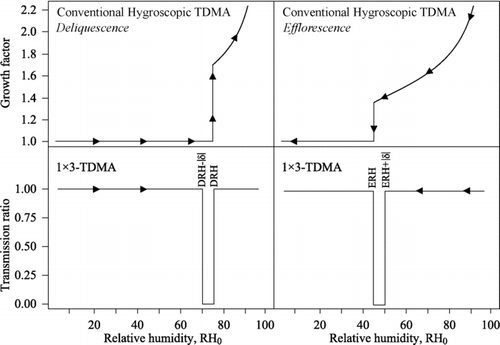
FIG. 3 Simulation showing the evolution of the number size distribution of sodium chloride particles inside the 1 × 3-TDMA for a deliquescence test. The three columns respectively show the distribution at RH0 prior to entering DMA mono (cf. ), after exiting DMA mono , and after the deliquescence test of RH0+ δ back to RH0 (δ=+5). The three rows successively show steps of RH0 from 67, to 72, to 77%. The black vertical bars show 0% transmission edges for 100-nm mobility diameter (one-charge equivalent). Distributions corresponding to aqueous particles are shown in red and those corresponding to solid particles are shown in blue.
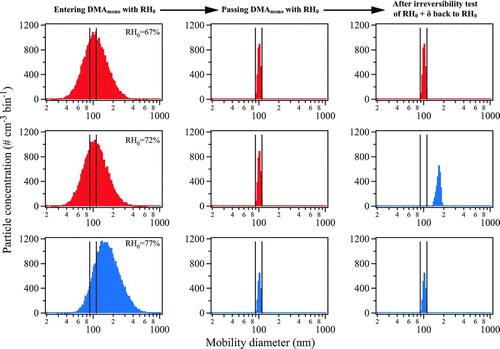
FIG. 4 Simulation of the 1 × 3-TDMA response showing (A1) the number concentration of particles reaching CPC0 in red and CPC+δ in blue when RH0 is scanned from low to high (i.e., “a deliquescence scan”), (B1) the concentration of particles reaching CPC0 in red and CPC−δ in blue when RH0 is scanned from high to low (i.e., “an efflorescence scan”), (A2) the ratio CPC+δ:CPC0 for the deliquescence scan, and (B2) the ratio CPC−δ:CPC0 for the efflorescence scan. Conditions: a test aerosol of 50,000 aqueous NaCl particles and 50,000 solid NaCl particles for the 1 × 3-TDMA set at a 100-nm mobility diameter (one-charge equivalent) and δ= ±5%. The size distribution of the particles prior to DMA0 is the same as in . The difference in the minimum transmission ratios (i.e., different from 0.5) in panels A2 and B2 as well as the noise in the simulation are attributable to insufficient particle counting statistics (i.e., more than 100,000 particles are necessary in the simulation to get an ideal response).
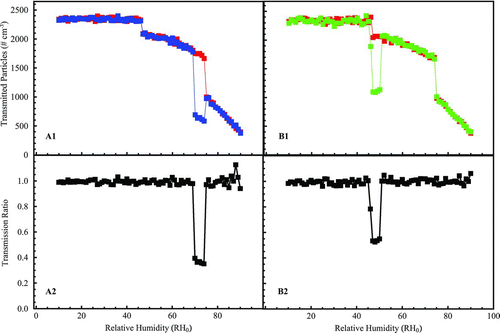
FIG. 5 Diagnostics and data recorded for a test aerosol of externally mixed aqueous and solid particles of aqueous and solid NaCl particles (80:20 mixture) with the 1 × 3-TMDA set to 100-nm mobility diameter (one-charge equivalent) and δ= ±5%. The figure shows the reproducibility and the stability of measurements. (a) Diagnostics of the relative humidities RH+δ, RH−δ, and RH0 in the test arms and the reference arm, the differences in RH between the test arms and the reference arm (i.e., δ), and the difference in the reference arm between the measured RH and its setpoint. (b) Data of the CPC measurements CPC0, CPC+δ, and CPC−δ for the three test arms and the corresponding transmission ratios CPC+δ:CPC0 and CPC−δ:CPC0. The labeled spikes i to iv are discussed in the text. For clarity, except for the final full upscan-downscan represented by the gray boxes, the upscan CPC−δ:CPC0 and the downscan CPC+δ:CPC0 are omitted from the figure because these data are not included in the later analysis.
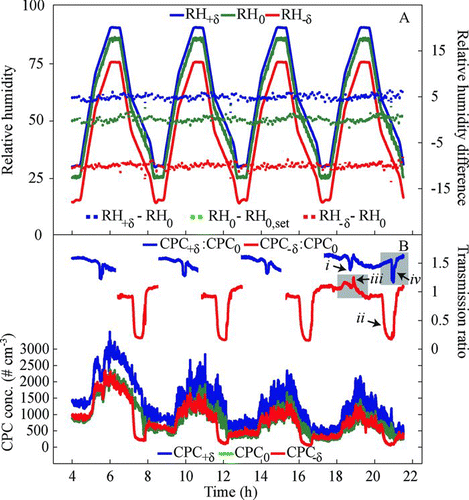
FIG. 6 1 × 3-TDMA response of CPC+δ:CPC0 and CPC−δ:CPC0 to test aerosols of externally mixed aqueous and solid particles of (a) ammonium sulfate (50:50 mixture; δ= ±5%) and (b) sodium chloride (85:15 mixture; δ=+5% for the deliquescence test and δ= −10% for the efflorescence test). Only upscans are shown for deliquescence (blue) and only downscans for efflorescence (red). The selected mobility diameter is 100 nm (one-charge equivalent).
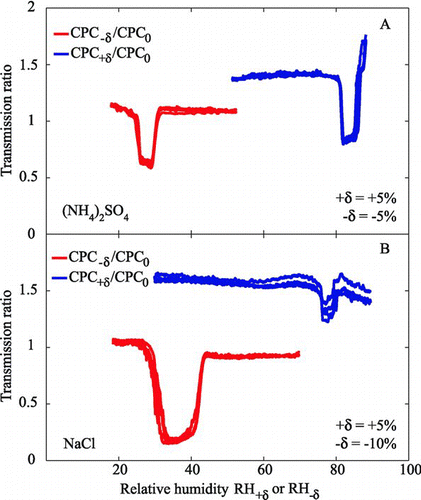
FIG. 7 1 × 3-TDMA response of CPC+δ:CPC0 and CPC−δ:CPC0 to test aerosols of (a) a 20:10:40:20 mix of AS(aq):AS(s):NaCl(aq):NaCl(s) externally mixed particles and with 1 × 3-TDMA set to δ= ±3% and (b) a 50:50 mix of low-RH (exposed to RH < 5%) and high-RH (exposed to RH > 95%) sea salt particles with 1 × 3-TDMA set to δ= ±5%. Other conditions and labeling are as for .
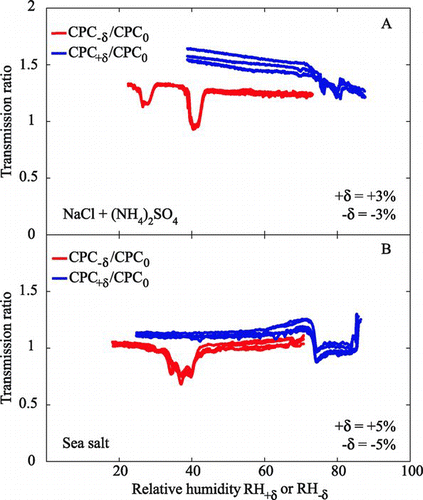
3. THEORY OF OPERATION AND SIMULATIONS
The top panels of show idealized growth-factor responses of a conventional HTDMA for deliquescence (left) and efflorescence (right) scans (CitationRader and Mcmurry 1986; CitationBiskos et al. 2006b), and these responses can be compared to idealized transmission-ratio responses of the 1 × 3-TDMA shown in the bottom panels. In these examples, the modeled aerosol consists of NaCl particles, and the 1 × 3-TDMA is operated with δ=±5%. For the deliquescence scans, the NaCl particles are initially solid, while for the efflorescence scans they are initially aqueous. The figure shows that the response of 1 × 3-TDMA is a boxcar for which the transmission ratio drops from 1.0 to 0.0 for DRH – |δ|< RH0< DRH for the deliquescence test of δ=+5% for solid particles and to 0.0 for ERH < RH0< ERH +|δ| for the efflorescence test of δ= −5% for aqueous particles. In comparison, the figure visually confirms that the response of the conventional HTDMA to these phase transitions is less detectable. The 1 × 3-TDMA has the potential to have a greater sensitivity to the irreversible nature of phase transitions than has a conventional hygroscopic HTDMA, with the price that the hygroscopic growth factor is not measured by the 1 × 3-TDMA. From another viewpoint, when the test aerosol of is regarded as an unknown aerosol, setting δ=+5% and scanning the central RH value from 20 to 85% RH reveals deliquescence at 75% RH. Using δ= –5% and scanning the central RH value from 85 to 20% RH reveals efflorescence at 45% RH.
The RH0 domain of the 1 × 3-TDMA can be subdivided into five useful subdomains: (Equation1) RH0< ERH, (Equation2) ERH < RH0< ERH +|δ|, (Equation3) ERH +|δ|< RH0< DRH – |δ|, (4) DRH – |δ|< RH0< DRH, and (5) DRH < RH0 (cf. ). For the first subdomain of RH0< ERH, a proposed test aerosol of 85% solid and 15% aqueous particles is reconditioned to 100% solid particles in the inlet RH0, and neither the deliquescence test (e.g., 20% → 25% → 20% for RH0= 20% and δ=+5%) nor the efflorescence test (e.g., 20% → 15% → 20% for RH0= 20% and δ= −5%) changes the particle diameter. Therefore, the theoretical transmission ratio is 1.0 for both the deliquescence and efflorescence tests.
TABLE 1 Transmission ratios for five subdomains of RH0 during efflorescence and deliquescence scans. The test aerosol before entering the 1 × 3-TDMA consists of a fraction faq of aqueous particles and a fraction fsol of solid particles
In the next RH subdomain of ERH < RH0< ERH + |δ|, the aerosol enters the first DMA as 15% metastable aqueous particles and 85% stable solid particles. The deliquescence test (e.g., 47% → 52% → 47%) changes the physical state of neither the aqueous nor the solid particles, and so their electric mobilities are also unchanged. Particle transmission remains at 1.0. In contrast, the efflorescence test (e.g., 47% → 42% → 47%) changes the physical state of aqueous particles to solid, thereby decreasing the diameter of 15% of the aerosol particles. These particles do not pass the second DMA, so the transmission ratio drops to 0.85. Analogous lines of reasoning hold for the other subdomains of the RH0 scan, including the dip in the transmission ratio to 0.15 for the DRH test for the fourth subdomain DRH – |δ|< RH0< DRH. This reasoning also leads to the conclusion that the boxcar RH widths of the second and fourth subdomains are equal to |δ|, corresponding to the RH span over which one of the test arms induces a phase transition while the reference arm does not.
3.1. Simulation
The particle response inside the 1 × 3-TDMA during a deliquescence test is illustrated by the simulation shown in . The Monte Carlo simulation incorporates particle charging of +1, +2, and +3, phase changes with RH cycling in the test arms (e.g., RH0 → RH+δ → RH0), and DMA transfer functions. The geometric mean diameter of the particle population in the aerosol is 100 nm, with a geometric standard deviation of 1.6. The simulation includes 106 particles. Sodium chloride particles are simulated, incorporating both hygroscopic growth of the aqueous particles and phase changes between the solid and aqueous phases based on DRH = 75% and ERH = 45%. The DMAs are tuned to a mobility diameter of 100 nm (one-charge equivalent). The simulation omits diffusional and electrostatic loss in the transport lines and the Nafion conditioners.
The top left panel of shows the initial particle size distribution for RH0= 67% prior to DMA mono . The particles are solid (coded red). The next panel to the right shows the electric-mobility-selected distribution after passing DMA mono while remaining at 67% RH. The third panel shows the distribution after an RH cycle from 72% (RH+δ) back to 67% (RH0). The distribution is unchanged by this cycle, and the deliquescence test is therefore negative. The transmission ratio, as defined by the number count of CPC+δ to that of CPC0, is 1.0.
The second row of shows the results for a simulation having RH0= 72%. The particle number size distributions prior to DMA mono and exiting it are identical for RH0= 67% and 72%. The last panel, however, differs. The changed size distribution shows that the deliquescence test from 77% (RH+δ) back to 72% (RH0) is positive. The aqueous particles (coded blue) increase in diameter and hence decrease in electric mobility. The particle size distribution grows out of the electric-mobility selection window of the second DMA. As a result, the transmission ratio is 0.0.
The third row shows the simulation of a deliquescence test using RH0= 77%. The particle size distribution prior to DMA mono shifts to larger diameters compared to RH0 of 67% and 72% because the aqueous particles (coded blue) grow hygroscopically compared to the solid particles. The middle panel shows that fewer particles exit DMA mono at 77% RH compared to at 67% and 72% RH because of this shifted distribution. The deliquescence test of an RH cycle from 77% to 82% (RH+δ) back to 77% (RH0) results in a transmission ratio of 1.0 because the initial condition of the particles is in the aqueous state. (See Figure S3 for a simulation similar to that of but for an efflorescence test.)
The simulation results in show the CPC responses (top row) and the resulting transmission ratios (bottom row) for deliquescence and efflorescence tests performed on an aerosol consisting of 40% solid and 60% aqueous sodium chloride particles. The simulation is run for δ= ±5%. Panel A1 (δ=+5%) shows the response of CPC+δ (blue) and CPC0 (red). These quantities co-vary until deliquescence at RH0= 70%, at which point the signal from CPC+δ drops abruptly because the deliquesced particles do not penetrate DMA+δ. This effect is clearly seen in panel A2, in which the transmission ratio CPC+δ:CPC0drops from 1.0 to 0.4. The signal recovers when RH0 reaches 75% (i.e., shifting from the subdomain of DRH – |δ|< RH0< DRH to that of DRH < RH0). The figure also shows that the number concentration measured by CPC+δ decreases after deliquescence (i.e., RH0> 77%), which can be explained by the shift in the particle population sampled by DMA mono as a result of the phase change (cf. ). The drop in CPC counts, however, is nulled by the reference arm so that CPC+δ:CPC0 is unaffected. Related results are shown in panels B1 and B2 for efflorescence scans (δ= −5%). These examples illustrate that the approach of using parallel arms for simultaneous measurements increases instrument sensitivity because some aspects of variability, such as of the test aerosol or of instrumental factors, do not influence the transmission ratio.
3.2. Resolution and Detection
For a decrease in the transmission ratio during a 1 × 3-TDMA scan to correspond directly to the fraction of particles that have undergone a phase change, the particle size distribution passing DMA mono must be shifted sufficiently that there is no overlap with the size distribution transmitted by DMA±δ. The requirement can be analyzed quantitatively by analyzing the required change in particle electric mobility. The maximum and minimum particle electric mobility (denoted Zmax and Zmin , respectively) passing a DMA at a setpoint mobility of Zcentral depends on the aerosol flow qa and the sheath flow qsh (CitationKnutson and Whitby 1975):
In a deliquescence test, complete separation between particles in DMA mono and DMA+δ requires that Zmax +δ<Zmin mono . Based on Equation (Equation1) and using n= 1, this inequality is satisfied by the following relationship:
In analogy, for an efflorescence test to give complete separation between particles in DMA mono and DMA−δ, the condition Zmin −δ>Zmax mono must be fulfilled. Based on Equation (Equation1) and using n= 1, this inequality is satisfied by the following relationship:
Compared to an aerosol of pure salt particles, the response of the 1 × 3-TDMA to an aerosol of internally mixed hygroscopic components is more complicated. The transmission ratio drops below unity for any points of irreversibility in the hygroscopic growth. For example, the hygroscopic growth factor of a two-component salt system has an irreversible response during a deliquescence scan for RH cycles that straddle either the eutonic RH or the final DRH. Whether the transmission ratio drops to zero for the eutonic RH or the final DRH depends on the magnitude of g with the irreversible event (i.e., g sol→aq > 1.17). A further caveat is that for some two-component salt systems an RH cycle straddling the eutonic RH during a deliquescence scan may represent a reversible cycle in the growth factor, i.e., for the case that the undissolved salt at the elevated RH is a good heterogeneous nucleus for the dissolved salt at the reduced RH in the cycle RH0 → (RH0+ |δ|) → RH0 during a deliquescence scan.
Equations (Equation2) and (Equation3) define the condition on g for complete particle filtering by DMA±δ compared to DMA0 for an irreversible change in the growth factor. This condition ensures that the decrease in the transmission ratio corresponds directly to the fraction of particles that have undergone a phase change. A complementary perspective on the detection limit of the 1 × 3-TDMA is the condition on g, such that a detectable change in the transmission ratio can be observed. The data in Figures and suggest that the baseline noise in the transmission ratio for laboratory conditions is 1%. The noise depends among other factors on the particle number concentration, and the noise therefore increases for field conditions because of lower concentrations of ambient particles. The baseline noise of CitationMartin et al. (2008) under field conditions was 5%. Simulations of the type represented in suggest that g sol→aq > 1.01 and g sol→aq > 1.03 should be just sufficient for baseline noise of 1% and 5%, respectively, to detect decreases in the transmission ratio across an RH window of RH0 to (RH0+ |δ|) during a deliquescence scan. This analysis is a lower limit for the condition are g sol→aq (especially for field conditions) because it assumes that the aerosol consists of a homogeneous particle population. For efflorescence scans, the conditions are g aq→sol < 0.99 and g aq→sol < 0.97 for laboratory and field conditions, respectively.
4. EXPERIMENTS AND OBSERVATIONS
Diagnostics during a typical run of the 1× 3-TDMA for an aerosol composed of an 80:20 mix of aqueous and solid sodium chloride particles are shown in . Shown in are the relative humidities RH0, RH+δ, and RH−δ for the three arms. The RH profile alternates between a downramp to 25% and an upramp to 85% RH. A variable scan rate is used for a total ramp time of 60 min. Also shown are (Equation1) the difference between the two test loops and the reference loop (i.e., +δ= RH+δ − RH0 and −δ= RH−δ – RH0) and (Equation2) the difference between the reference loop and the set RH0 profile (i.e., RH0 - RH0, set ). The figure shows that |+δ| and |−δ| track within 1% the setpoint values of 5% and 10%, respectively.
The primary measurements of the 1 × 3-TDMA are the particle number concentrations penetrating the reference, deliquescence, and efflorescence test arms. shows the primary responses CPC0, CPC+δ, and CPC−δ as well as the derived ratios CPC+δ:CPC0 and CPC−δ:CPC0 to the challenge aerosol. The base CPC count of the arms varies during an RH ramp because different slices of the particle number size distribution are selected by DMA mono when RH changes (cf. Figures and ). As expected, however, the ratios CPC+δ:CPC0 and CPC−δ:CPC0 have low variability over the same range of RH. For ideal operating conditions, CPC+δ:CPC0 and CPC−δ:CPC0 should be unity outside of RH subdomains having phase transitions ().
Although the baseline CPC−δ:CPC0 is unity as expected (), CPC+δ:CPC0 is approximately 1.5. The explanation is that the DMA transmission functions and line losses are not perfectly balanced (i.e., different flow rates) between the deliquescence test and the reference arm for the experiments of . The boxcars of the phase transitions, however, are clearly differentiated as relative changes from the local baseline of 1.5. The flow imbalance is attributable mostly to the critical orifices used on the “excess out”; these orifices were built by the in-house workshop and had greater variability than commercially available products. also shows that the local baseline drifts somewhat from 1.5 during a scan. Intra-instrument variability in the time series of temperature, relative humidity, and flow can explain the drift.
In , the blue downspikes (labeled i) are deliquescence boxcars during an RH upscan, and the red downspikes (labeled ii) are efflorescence boxcars during an RH downscan. Boxcars i and ii therefore correspond to the responses modeled in Figures -a2 and -b2, respectively. The data for the four consecutive measurements shown in show that the measured DRH of sodium chloride varies between 75 and 76% RH. Although the precision is therefore 1%, the accuracy is estimated as ±2% taking into account the uncertainty of the RH measurements and the temperature fluctuations during a scan. The data for the four consecutive measurements of efflorescence show that the measured ERH of sodium chloride varies between 43 and 44% RH.
In addition to the down boxcars at the expected RH values of deliquescence and efflorescence, also shows up boxcars iii and down boxcars iv. These artifact boxcars are attributable to instrumental gradients of 1 to 2% RH. For example, an efflorescence test is susceptible near DRH to artifact boxcars such as iii because there are small differences among (RH0) i in (RH0)1 → (RH0−|δ|) → (RH0)2 of the efflorescence test arm in comparison to (RH0)1 → (RH0)3 → (RH0)4 of the reference arm. As a result, for (RH0) i in the vicinity of DRH, solid particles can deliquesce in the efflorescence arm at a slightly different time point compared to those in the reference arm, inducing artificial differences in CPC−δ and CPC0 and hence spikes in the their ratio. A case of (RH0)1 ⩽ (RH0)3= (RH0)4< (RH0)2 represents one possible permutation of the relative values of (RH0) i . For such a case, as (RH0)1 increases, the deliquescence of the solid particles occurs in the reference arm (implying that CPC0 decreases) slightly in advance of the deliquescence of the solid particles in the efflorescence arm because (RH0)4< (RH0)2. CPC−δ:CPC0 therefore spikes upward for a gap ΔRH = (RH0)4 - (RH0)2, which is typically 1 to 2% RH. In the data of , these upward spikes appear as boxcars iii. (A case of (RH0)1 ⩽ (RH0)2< (RH0)3= (RH0)4 would lead to downward spikes. A case of (RH0)2 ≈ (RH0)3 ≈ (RH0)4< (RH0)1 would nearly eliminate the spikes because the artifacts in CPC−δ:CPC0 would depend not on a phase transition but instead on a difference in growth factors at (RH0)2 compared to (RH0)4.) Related shifts in the relative time points of efflorescence for aqueous particles in the deliquescence test arm compared to those in the reference arm can explain spike iv for RH0 in the vicinity of ERH. Because the artifact boxcars are present for the upscan in the deliquescence test and for the downscan in the efflorescence test, further data presented in Figures and are shown for CPC+δ:CPC0 of the deliquescence test for upscans only and for CPC−δ:CPC0 of the efflorescence test for downscans only.
An implication of this analysis is that, for the practical operation of the 1 × 3-TDMA, |δ| must be significantly greater than the small differences among (RH0) i (e.g., we employ |δ|= 5% in this study), and measures should be taken to minimize the differences among (RH0) i . The small differences among (RH0) i originate both from temperature differences of 0.1 to 0.2 K in the humidity conditioners (affecting all i) and DMAs (affecting i∈2,4) and from variability in the time constant for RH accommodation for the Nafion material (affecting all i), even for Nafion bundles that are nominally identical. The artifact boxcars decrease when the RH ramp is slowed (results not shown), confirming the importance of RH accommodation in the Nafion material.
shows the transmission ratios using |δ|= 5 for upscans of the deliquescence test and for downscans of the efflorescence test of a test aerosol containing an external 50:50 mixture of aqueous and solid ammonium sulfate particles. The abscissa is RH+δ for the upscans and RH−δ for the downscans. Several different runs are overlaid to show reproducibility. shows a similar experiment performed with an 85:15 mixture of aqueous and solid sodium chloride particles. In this experiment the Δ values were set to +5% RH and −10% RH for the deliquescence and efflorescence test arms, respectively. The measured DRH values of 80 ± 2% and 75 ± 2% for ammonium sulfate and sodium chloride, respectively, and the measured ERH values of 33 ± 2% and 43 ± 2%, respectively, agree with values measured in our laboratory using an HTDMA (CitationBiskos et al. 2006a, Citationc). The values also agree with literature reports of 79–81% and 75–76% for DRH and of 33–37% and 40–46% for ERH for the respective materials (CitationTang and Munkelwitz 1993, Citation1994; CitationMartin 2000). The results in the figure also demonstrate that for these experiments the relative depths of the DRH and ERH boxcars corresponds to the fractions in the test aerosol of the particles in the solid and aqueous states, respectively. using the deliquescence test shows a response of approximately 15% and the efflorescence test shows a response of approximately 85%, indicating an external mixture of 15% solid and 85% aqueous particles, in agreement with the prepared aerosol (Section 2.3).
shows two examples for more complicated test aerosols, one externally and one internally mixed (Figures and b, respectively). The test aerosol corresponding to contains an external mixture of aqueous and solid ammonium sulfate and sodium chloride particles in the ratio 20:10:40:20. Efflorescence events are therefore expected at 33% (medium strength) and 45% RH (strong), and deliquescence events are expected at 75% (weak) and 80% RH (very weak). shows that the 1 × 3 TDMA setup picks up all four phase change events, showing one of the strengths of this approach. For this experiment, to enhance the resolution |δ|= 3% was used. For |δ|= 5% (results not shown), the instrument does not separate the two DRH events at 75% RH (NaCl) and 80% RH (AS).
The test aerosol corresponding to contains internally mixed sea salt ions in a 50:50 external mixture of aqueous particles (exposed to RH > 95%) and particles dried to RH < 5%. The boxcar for the deliquescence test (blue line) occurs at 73% RH, corresponding to the irreversible deliquescence of sodium chloride mixed with other salts. The width of 10% RH for the boxcar exceeds Δ (5%), indicating sustained irreversibility of the hygroscopic growth factor from 73% to 78%. This response can be explained by an irreversible event at 73% RH, such as full dissolution of one crystal for a mixed salt, whose boxcar is incompletely resolved (i.e., because δ= 5%) from another irreversible event of final deliquescence at 78% RH.
The efflorescence test in shows an initial drop in the transmission ratio at 42% RH. Although poorly formed, the boxcar has a width of 10% RH and therefore exceeds Δ. Moreover, some structure is discernable in the bottom of the boxcar. Combined, these facts indicate stepwise crystallization from 42 to 37% RH or alternatively irreversible changes in particle shape with RH cycling across this RH range.
For comparison to these results, CitationCziczo et al. (1997) studied the drying and hydration of artificial sea salt particles using an aerosol flow tube with infrared spectroscopy. Water remained in the particles to low RH (i.e., <2% RH). The authors hypothesized that either the particles did not crystallize or solid hydrates of Mg2+ such as MgCl2·6H2O formed. The authors also showed significant water uptake for RH > 50% upon hydration. Similar behavior was reported for sea salt particles by CitationTang et al. (1997) and CitationLiu et al. (2008). Under the assumption that similar behavior occurs for the experiments of , the implication is that the water uptake from 50% to 73% is reversible, and that irreversible changes such as full dissolution of critical crystals do not initiate until 73% RH (CitationHarvie et al. 1980).
5. APPLICATIONS AND OUTLOOK
The 1 × 3-TDMA was introduced in this article with a focus on using the efflorescence and deliquescence arms in the condition of equal but opposite Δ values and with application to a single particle electric mobility. Minor alterations to this protocol can broaden the potential scope and application of the 1 × 3-TDMA. In addition to phase transitions, other processes can contribute to irreversible changes in the particle size distribution and are therefore can potentially be studied by the 1 × 3-TDMA. These processes include irreversible evaporation of organic molecules with a change in water content, irreversible changes in particle morphology with RH cycling, and irreversible particle-phase chemical reactions accompanying a change in water content. The 1 × 3-TDMA also has the potential to select particles between 10 and 190 nm mobility diameter (one-charge equivalent). The analysis of multiple size fractions can be important for aerosol particles having several modes of different chemical composition or alternatively having a nanosize effect on deliquescence or efflorescence (CitationBiskos et al. 2006b, Citationc). Another option is to employ independent but negative Δ values for both test loops (e.g., −5% and −10%), thus gaining redundancy in the efflorescence test. In this mode of operation, phase transitions will give identical results for both −5% and −10% whereas some other mechanisms, such as RH-dependent irreversible evaporation of organic molecules, will give different results in each arm. A final possibility, especially relevant to ambient studies, is to fix RH0 and |δ| at values that lie within a deliquescence boxcar and then track CPC+δ:CPC0 with time to observe the diel evolution of the phase-transition fraction. Tracking in the efflorescence boxcar is equally possible.
The 1 × 3-TDMA can be expanded in simple ways to provide additional capability. A particle dryer of 5% RH before the inlet to initiate all particles in a common state can help to determine the fraction of the particle population that is able to deliquesce. This fraction, in conjunction with a measurement of the fraction of particles that deliquesce when the inlet is set to ambient RH, can be used as a measure of the fraction of particles in a metastable state in the atmosphere. Similarly, placement of a humidifier of 90% RH before the inlet can provide complementary information about the efflorescence fraction.
Field campaigns and laboratory results obtained using the 1 × 3-TDMA will help to explain and understand in greater quantitative detail the life cycle processes of atmospheric particles and thus to improve the performance of predictive models of Earth's climate. An understanding of the growth factor as a path function is a requirement in the development of mechanistic, predictive models of the effects of aerosol particles on the direct radiative forcing of climate.
UAST_A_382371_SUP_0001.zip
Download Zip (731.1 KB)Acknowledgments
[Supplementary materials are available for this article. Go to the publisher's online edition of Aerosol Science and Technology to view the free supplementary files.]
This material is based upon work supported by the National Science Foundation under Grant No. ATM-0317583 and ATM-0633840 and by the Danish Natural Science Research Council Grant No. 272-05-0328 and 272-06-0318. Any opinions, findings, and conclusions or recommendations expressed in this material are those of the authors and do not necessarily reflect the views of the National Science Foundation. Helpful discussions with D.R. Collins in the development of the instrument are gratefully acknowledged.
References
- Biskos , G. , Paulsen , D. , Russell , L. M. , Buseck , P. R. and Martin , S. T. 2006a . Prompt Deliquescence and Efflorescence of Aerosol Nanoparticles . Atmos. Chem. Phys. , 6 : 4633 – 4642 .
- Biskos , G. , Russell , L. M. , Buseck , P. R. and Martin , S. T. 2006b . Nanosize Effect on the Hygroscopic Growth Factor of Aerosol Particles . Geophys. Res. Lett. , 33 : L07801
- Biskos , G. , Malinowski , A. , Russell , L. M. , Buseck , P. R. and Martin , S. T. 2006c . Nanosize Effect on the Deliquescence and the Efflorescence of Sodium Chloride Particles . Aerosol Sci. Technol. , 40 : 97 – 106 .
- Chan , M. N. and Chan , C. K. 2005 . Mass Transfer Effects in Hygroscopic Measurements of Aerosol Particles . Atmos. Chem. Phys , 5 : 2703 – 2712 .
- Cziczo , D. J. , Nowak , J. B. , Hu , J. H. and Abbatt , J. P. D . 1997 . Infrared Spectroscopy of Model Tropospheric Aerosols as a Function of Relative Humidity: Observation of Deliquescence and Crystallization . J. Geophys. Res. , 102 : 18843 – 18850 .
- Gysel , M. , Weingartner , E. and Baltensperger , U. 2002 . Hygroscopicity of Aerosol Particles at Low Temperatures. 2. Theoretical and Experimental Hygroscopic Properties of Laboratory Generated Aerosols . Environ. Sci. Technol. , 36 : 63 – 68 .
- Harvie , C. E. , Weare , J. H. , Hardie , L. A. and Eugster , H. P. 1980 . Evaporation of Seawater: Calculated Mineral Sequences . Science , 208 : 498 – 500 .
- Hegg , D. , Larson , T. and Yuen , P. F. 1993 . A Theoretical Study of the Effect of Relative Humidity on Light Scattering by Tropospheric Aerosols . J. Geophys. Res , 98 : 18435 – 18439 .
- Kinne , S. , Schulz , M. , Textor , C. , Guibert , S. , Balkanski , Y. , Bauer , S. E. , Berntsen , T. , Berglen , T. F. , Boucher , O. , Chin , M. , Collins , W. , Dentener , F. , Diehl , T. , Easter , R. , Feichter , J. , Fillmore , D. , Ghan , S. , Ginoux , P. , Gong , S. , Grini , A. , Hendricks , J. E. , Herzog , M. , Horowitz , L. , Isaken , L. , Iversen , T. , Kirkavag , A. , Kloster , S. , Koch , D. , Kristjansson , J. E. , Krol , M. , Lauer , A. , Lamarque , J. F. , Lesins , G. , Liu , X. , Lohmann , U. , Montanaro , V. , Myhre , G. , Penner , J. E. , Pitari , G. , Reddy , S. , Seland , O. , Stier , P. , Takemura , T. and Tie , X. 2006 . An AeroCom Initial Assessment—Optical Properties in Aerosol Component Modules of Global Models . Atmos. Chem. Phys. , 6 : 1815 – 1834 .
- Khlystov , A. , Stanier , C. O. , Takahama , S. and Pandis , S. N. 2005 . Water Content of Ambient Aerosol during the Pittsburgh Air Quality Study . J. Geophys. Res. , 110 : D07S10
- Knutson , E. O. and Whitby , K. T. 1975 . Aerosol Classification by Electric Mobility: Apparatus, Theory, and Applications . J. Aerosol Sci. , 6 : 443 – 451 .
- Liu , Y. , Yang , Z. , Desyaterik , Y. , Gassman , P. L. , Wang , H. and Laskin , A. 2008 . Hygroscopic Behavior of Substrate-Deposited Particles Studied by Micro-FT IR Spectroscopy and Complementary Methods of Particle Analysis . Anal. Chem , 80 : 633 – 642 .
- Martin , S. T. 2000 . Phase Transitions of Aqueous Atmospheric Particles . Chem. Rev. , 100 : 3403 – 3453 .
- Martin , S. T. , Hung , H. M. , Park , R. J. , Jacob , D. J. , Spurr , R. J. D. , Chance , K. V. and Chin , M. 2004 . Effects of the Physical State of Tropospheric Ammonium-Sulfate Nitrate Particles on Global Aerosol Direct Radiative Forcing . Atmos. Chem. Phys. , 4 : 183 – 214 .
- Martin , S. T. , Rosenoern , T. , Chen , Q. and Collins , D. R. 2008 . Phase Changes of Ambient Particles in the Southern Great Plains of Oklahoma, USA . Geophys. Res. Lett. , 35 : L22801
- McFiggans , G. , Artaxo , P. , Baltensperger , U. , Coe , H. , Facchini , M. C. , Feingold , G. , Fuzzi , S. , Gysel , M. , Laaksonen , A. , Lohmann , U. , Mentel , T. F. , Murphy , D. M. , O'Dowd , C. D. , Snider , J. R. and Weingartner , E. 2006 . The Effect of Physical and Chemical Aerosol Properties on Warm Cloud Droplet Activation . Atmos. Chem. Phys. , 6 : 2593 – 2649 .
- Pitchford , M. L. and McMurry , P. H. 1994 . Relationship Between Measured Water Vapor Growth and Chemistry of Atmospheric Aerosol for Grand Canyon, Arizona, in Winter 1990 . Atmos. Environ. , 28 : 827 – 839 .
- Rader , D. J. and McMurry , P. H. 1986 . Application of the Tandem Differential Mobility Analyzer to Studies of Droplet Growth or Evaporation . J. Aer. Sci. , 17 : 771 – 787 .
- Santarpia , J. L. , Li , R. J. and Collins , D. R. 2004 . Direct Measurement of the Hydration State of Ambient Aerosol Populations . J. Geophys. Res. , 109 : D18209
- Seinfeld , J. H. and Pandis , S. N. 2006 . Atmospheric Chemistry and Physics , 2nd edition , New York : Wiley .
- Solomon , S. , Qin , D. , Manning , M. , Chen , Z. , Marquis , M. , Averyt , K. B. , Tignor , M. and Miller , H. L. 2007 . “ IPCC, Climate Change 2007: The Physical Science Basis ” . New York : Cambridge University Press .
- Stumm , W. and Morgan , J. J. 1996 . “ ı ” . In Aquatic Chemistry , 3rd , New York : Wiley .
- Swietlicki , E. , Hansson , H. C. , Hameri , K. , Svonningsson , B. , Massling , A. , McFiggans , G. , McMurry , P. H. , Petaja , T. , Tunved , P. , Gysel , M. , Topping , D. , Weingartner , E. , Baltensperger , U. , Rissler , J. , Wiedensohler , A. and Kulmala , M. 2008 . Hygroscopic Properties of Submicrometer Atmospheric Aerosol Particles Measured with H-TDMA Instruments in Various Environments—A Review . Tellus , 60B : 432 – 469 .
- Tang , I. N. and Munkelwitz , H. R. 1993 . Composition and Temperature Dependence of the Deliquescence Properties of Hygroscopic Aerosols . Atmos. Environ. , 27 : 467 – 473 .
- Tang , I. N. and Munkelwitz , H. R. 1994 . Aerosol Phase Transformation and Growth in the Atmosphere . J. Appl. Meteor. , 33 : 791 – 796 .
- Tang , I. N. , Tridico , A. C. and Fung , K. H. 1997 . Thermodynamic and Optical Properties of Sea Salt Aerosols . J. Geophys. Res. , 102 : 23269 – 23275 .
- Wang , J. , Hoffmann , A. A. , Park , R. , Jacob , D. J. and Martin , S. T. 2008 . Global Distribution of Solid and Aqueous Sulfate Aerosols: Effect of the Hysteresis of Particle Phase Transitions . J. Geophys. Res. , 113 : D11206
- Wang , J. , Jacob , D. J. and Martin , S. T. 2008 . Sensitivity of Sulfate Direct Climate Forcing to the Hysteresis of Particle Phase Transitions . J. Geophys. Res. , 113 : D11207