Abstract
An aerosol mass spectrometer for measurements of the alkali metal content in individual submicron aerosol particles is presented. The instrument combines surface ionization of individual particles on a hot platinum surface with orthogonal acceleration time-of-flight mass spectrometry. The instrument simultaneously provides the content of different alkali metal elements in single particles with high sensitivity. The instrument is characterized in laboratory experiments, and determination of the alkali metal content is demonstrated for particle diameters of 50–500 nm. The technique is demonstrated in ambient air measurements at an urban background site, and sea spray particles and particles originating from biomass burning are identified based on their content of sodium and potassium. Possible further improvements and applications of the technique are discussed.
1. INTRODUCTION
Aerosol mass spectrometers (AMSs) have become important tools in aerosol research during recent years (CitationSuess and Prather 1999; CitationJohnston 2000; CitationNoble and Prather 2000; CitationSullivan and Prather 2005; CitationCoe and Allan 2006, CitationCanagaratna et al. 2007). They provide the aerosol composition in ambient air with high spatial and temporal resolution, and allow for detailed studies on the time-scales of relevance for aerosol processes in the atmosphere. Common to most existing AMS techniques is that aerosol particles are sampled into a vacuum system where they are decomposed, and the produced fragments are characterized by mass spectrometry. One approach combines laser ablation and ionization of single particles with time-of-flight mass spectrometry (CitationGard et al. 1997; CitationTrimborn et al. 2000; CitationZelenyuk and Imre 2005). In other types of instruments the particles are instead vaporized on a heated metal surface and the emitted gases are ionized by electron-impact ionization (CitationJayne et al. 2000; CitationJimenez et al. 2003). Volatile and semi-volatile components are monitored, while compounds that are not vaporized at the temperature of the heated surface are not detected. In one version of the technique, aerosol particles are collected on the metal surface during some time and they are thereafter thermally evaporated to receive integrated information about their composition (CitationTobias et al. 2000). In another method, aerosol components are ionized in direct contact with a hot surface by surface ionization (SI), and highly efficient detection can be achieved for elements with sufficiently low ionization potentials (Davies 1973, 1977; CitationMyers et al. 1975; CitationSinha et al. 1985; CitationStoffels et al. 1981; CitationSvane et al. 2004). A major strength of the different types of AMSs lies in their ability to identify particles from various sources in the atmosphere based on their chemical composition. The AMS methods are in rapid development and current challenges include improved quantification, organic speciation, lower size analysis limits, sampling efficiency, instrument miniaturization, and data analysis approaches.
We have recently described a new AMS instrument based on the SI technique (CitationSvane et al. 2004). The instrument employs an aerodynamic inlet system for efficient sampling of particles into vacuum, and the detection of individual particles is based on decomposition and SI on a hot platinum surface. The Pt surface has a box-like design that limits problems associated with particle bounce effects and incomplete ionization. A quadrupole mass spectrometer has so far been used for analysis of alkali ions. High transmission efficiencies and quantitative determination of the alkali metal content in individual particles with diameters down to 14 nm have been demonstrated. High size resolution can be achieved with the instrument, as illustrated by the detection of multiply charged particles passing through a DMA. The AMS has recently been applied in field studies in urban air (CitationSvane et al. 2005a) and in large-scale biomass combustion (CitationSvane et al. 2005b, Citation2006).
The recently described AMS based on the SI technique (CitationSvane et al. 2004) is further developed in the present study by the implementation of an orthogonal acceleration time-of-flight mass spectrometer (oa-TOFMS). The oa-TOFMS technique is a well suited method to interface a continuous or semi-continuous ion source to a TOFMS (CitationGuilhaus et al. 2000). The technique is based on TOFMS in a separate direction orthogonal to the ion-beam axis of the ion source, and it provides a higher ion sampling efficiency compared to other methods. The new instrument is capable of recording complete mass spectra for individual aerosol particles in the size range 50–500 nm. The multi-element detection capability significantly improves the chances of on-line identification of major aerosol components in ambient air, including sea salt particles, mineral dust, and particles from biomass burning and coal combustion. Laboratory studies with the aim to characterize the new instrument are described here, and the technique is demonstrated in ambient air measurements. The oa-TOFMS technique has previously been combined with several ionization methods including electrospray, matrix assisted laser desorption/ionization, electron impact ionization, and plasma ionization (CitationGuilhaus et al. 2000). In related work, CitationDrewnick et al. (2005) described an AMS based on electron impact ionization combined with an oa-TOFMS unit.
2. EXPERIMENTAL
The AMS described in the present study is a further development of a recently presented instrument (CitationSvane et al. 2004). A schematic picture of the vacuum system of the apparatus is shown in . The system consists of three differentially pumped vacuum chambers containing the aerosol inlet, the surface ionization unit, and the mass spectrometer unit. The vacuum system, the aerosol inlet system, and the surface ionization unit are identical to the ones described earlier (CitationSvane et al. 2004), and are only briefly presented here. The vacuum system is pumped by one mechanical and two turbomolecular pumps, and a pressure of 1·10–5 mbar is maintained in the main chamber during operation. Aerosol particles are drawn into the AMS through an aerodynamic lens system (CitationLiu et al. 1995a, Citation1995b), which produces a sharply focused particle beam with a high transmission of particles into the detection unit (CitationJayne et al. 2000; CitationSvane et al. 2004). The particle beam is directed onto a resistively heated platinum surface in the detection chamber. The Pt surface has been designed as a box (3 mm × 3 mm × 5 mm) made from thin Pt foil (thickness 0.025 mm). The particle inlet housing is tilted by 35° from the normal direction of the Pt surface, and the construction avoids problems due to particle bouncing effects on the hot surface (CitationSvane et al. 2004). Particles that stick inside the Pt box decompose in contact with the hot surface. The alkali metal content of the particles desorbs in ionic form from the metal surface, a phenomenon know as SI (CitationIonov 1972; CitationZandberg 1995). The ionization probability is determined by the difference between the work function of the metal and the ionization potential of the desorbing compound. The alkali metals have unusually low ionization potentials and the ionization probability may approach 100%. Most other compounds have higher ionization potentials than the alkali metals, thus desorption in neutral form dominates completely and ionic desorption is negligible. During the experiments the Pt surface was heated to a temperature of 1500 K, and the decomposition of an individual alkali salt particle on the hot surface typically resulted in emission of alkali ions over about 1 ms (CitationSvane et al. 2004).
FIG. 1 Cross-section view of the vacuum system of the AMS featuring (a) the particle inlet containing the aerodynamic lens system, (b) the ionizing platinum surface and a focusing electrode, (c) oa-TOFMS unit.
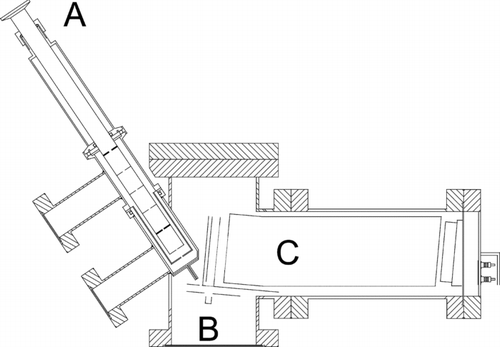
The main difference between the present instrument and the earlier version is that the previously used quadrupole mass spectrometer has been replaced with an orthogonal acceleration time-of-flight mass spectrometer (oa-TOFMS). This change makes it possible to obtain a complete mass spectrum for each detected particle instead of just one pre-selected ion mass. The oa-TOFMS unit has been integrated with the existing SI unit of the AMS. The oa-TOFMS consists of a linear time-of-flight unit, which limits the achievable mass resolution compared to instruments using an ion mirror for energy spread compensation (CitationGuilhaus et al. 2000). The small and simplified setup used here was, however, considered acceptable for the purpose of mass separation of alkali ions. The new setup is illustrated in . shows how ions emitted from the Pt surface are focused by a lens in front of the Pt surface, and a relatively narrow ion beam enters the “deflection volume” of the oa-TOFMS; note that grids with 90% transmission are indicated by thin solid lines. The “deflection volume” is confined between a deflector electrode and an opposite grid. Most of the time (12.51 μs) the deflector is kept at low voltage and ions fill up and pass through the deflection volume (). At a certain time, the deflector voltage is rapidly increased to 70 V over 2 μs (rise time 10 ns), and ions present in the volume are accelerated into the time-of-flight unit (). Ions originating from different positions within the deflection volume are focused in time during their time-of-flight and reach the ion detector situated directly after the flight tube at approximately the same time, as illustrated in . The setup is optimized so that new ions fill up the deflection volume during the time it takes for deflected ions to reach the ion detector. The ions initially have a kinetic energy of 11 eV in the forward direction and obtain an energy of 430 eV in the perpendicular direction after deflection into the time-of-flight tube. Due to the two ion velocity components parallel and perpendicular to the initial ion beam direction, the TOF unit is tilted by an angle as illustrated in .
FIG. 2 SIMION (SIMION 2000) calculations of ion trajectories in the instrument: (a) overview of the electrode setup, (b) focusing of ions emitted from the hot Pt surface into the MS, and (c) acceleration and subsequent field-free flight in the TOF section of the MS. The plotted trajectories are for K+ ions. Every 0.5 μs flight-time is marked on the trajectories in (a). The initial kinetic energies were 10 eV in (a) and (c), and 0.1 eV in (b). The following parts are indicated in panel (a): A = platinum surface, B = deflector electrode, and C = time-of-flight tube. Grids with 90% transmission are indicated by thin solid lines.
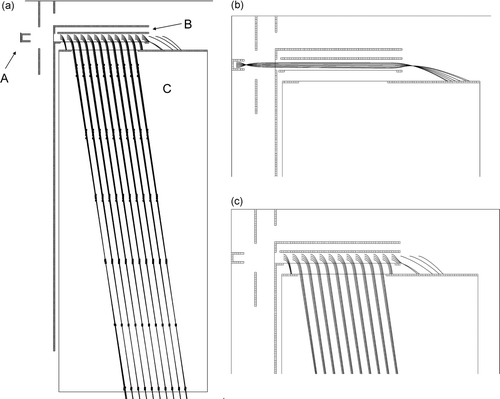
Ions are detected with a chevron type micro-channel plate MCP detector (model MCP-MA56/2, Del Mar Ventures). The output pulses from the MCP detector were amplified with a preamplifier (Ortec 9326-P) and counted by a computer-controlled Fastflight-2 Digital Signal Averager (DSA) (Advanced Measurement Technology Inc.). The time resolution of the DSA was 1 ns and pulses were sampled with a 4.51 μs time delay in 10000 channels during each 14.51 μs scan. 1000 consecutive scans were added to a mass spectrum with a 14.51 ms long data collection period, and mass spectra were continuously stored to a computer for further analysis. The signal from the DSA was recalculated into a number of detected ions based on a separate calibration experiment where the MCP detector was used as an ion collector. Signals are reported as the number of detected (or counted) ions, but it should be kept in mind that the DSA is an analog-to-digital converter and not a pulse counting unit.
During the laboratory evaluation of the AMS, the performance of the apparatus was tested using pure alkali salt particles. These were prepared from aqueous salt solutions with an atomizer (TSI model 3076) connected to a diffusion dryer (TSI model 3062). An electrostatic classifier (TSI model 3080), including a differential mobility analyzer (TSI model DMA 3081) was used to provide a monodisperse aerosol. The particle number concentration was measured with a condensation particle counter (TSI model 3010).
3. RESULTS
Laboratory experiments have been carried out with pure monodisperse alkali salt particles in the size range 50–500 nm to characterize the operation of the AMS. shows examples of recorded mass spectra for single particles with diameters of 70 and 300 nm. The particles were in this case generated from a solution with equal concentrations of sodium, potassium, rubidium, and cesium chloride salts and the particle size was pre-selected with a DMA before the particles entered the AMS. The signals from the different types of alkali ions are clearly resolved. The mass resolution in is relatively low and the instrument has been optimized for a high sensitivity. A mass resolution of about 350 has been achieved with the instrument for a case with relatively low sensitivity. The intensity is lower for Na than for K, Rb, and Cs. This is due to the design and operation of the oa-TOFMS that is a trade-off between acceptable detection probabilities for light and heavy ions. Light ions (Na+) have sufficient time to pass through the deflection volume during the 12.51 μs between consecutive deflections and are partially lost, which reduces the detection probability. The heavy ions (Rb+, Cs+) on the other hand travel a limited distance into the deflection region and are instead partially lost in the entrance region of the deflection volume (see ). The entrance loss effect applies to all ions, but the relative effect is smaller for light ions. The described loss effects are a common feature to all orthogonal TOF-MS designs and the importance of the different effects depends on the detailed design of each setup (CitationGuilhaus et al. 2000). SIMION (SIMION 2000) calculations of ion trajectories in the instrument were used to estimate the ion transmission of the TOF unit. Theoretical transmissions of 0.40, 0.53, 0.47, 0, 43 were calculated for Na+, K+, Rb+ and Cs+, respectively, and the estimates include the effects of three grids with a transmission of 0.9 that the ions have to pass. The relative intensities of different ions in qualitatively agree with the calculations, and a more thorough SIMION analysis including the combined hot Pt surface and the TOF-unit would probably improve the agreement further. A few tests with LiCl particles were also performed. Lithium binds more strongly to the Pt surface compared to the other alkali elements, and the surface temperature of 1500 K used here was too low to allow ion bursts from individual lithium salt particles to be detected. Instead, the lithium signal varies slowly over time reflecting integrated changes in lithium salt concentrations.
FIG. 3 Example of recorded mass spectra for single laboratory generated aerosol particles with diameters of 70 and 300 nm. Particles were generated from a solution with equal concentrations of sodium, potassium, rubidium, and cesium chloride salts, and particles with a given size were selected with a DMA before entering the AMS.
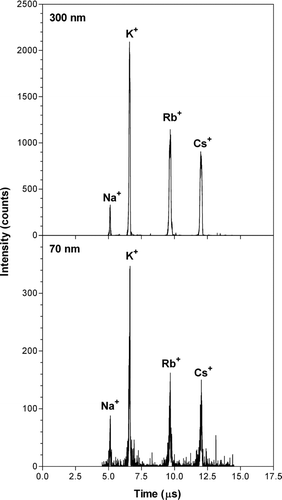
shows the number of ions detected for individual NaCl particles of different pre-selected sizes. The results are based on ca. 500 detected particles in each case and the integrated number of detected Na+ ions was determined for each particle. Similar results were obtained for other types of alkali salts. The individual distributions seen in are relatively narrow. This indicates that particle decomposition at the Pt surface proceeds in a similar way for all detected particles and that particle bounce is a limited problem, thus confirming the results from previous work (CitationSvane et al. 2004). The increased width of the distributions with increased particle size seen in was also observed in the earlier study, and it was concluded to be due to the transfer function of the DMA.
FIG. 4 Distributions of the number of counts observed for individual NaCl particles. The pre-selected diameters used in the experiments are indicated in the panels. The y-axis units are on the same scale.
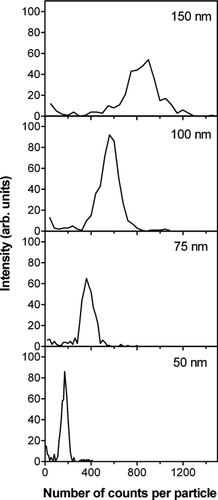
The relation between the detected number of ions and size of the alkali salt particles is illustrated in . The displayed data are average number of ion counts for a given particle diameter and the data are based on the type of distributions shown in . The sensitivity for different salts varies due to different detection probabilities in the oa-TOFMS unit, as described earlier in connection with . The ion signals increase rapidly with increasing particle size up to 300 nm, while a slower increase is observed for larger sizes. A limited set of experiments with mixed alkali salt particles also showed results that were consistent with the data in . CitationSvane et al. (2004) previously showed that close to complete ionization can be obtained with the Pt surface setup used in the present study. Differences between the two studies are the low potential (11 V) applied to the hot surface and the low field strength between the Pt surface and the first electrode in the present work compared to the earlier study. The low voltage results from the need to feed the oa-TOFMS with low energy ions, but this operation mode may result in high transient ion densities in the volume outside the ionizing surface that diverge the ion flux. We conclude that the observed signals are related to the alkali content of individual particles and that semi-quantitative measurements can be achieved after corrections for the non-linearity shown for large particle sizes in .
FIG. 5 Average number of ion counts per particle as a function of particle diameter. The particles were generated from solutions of sodium, potassium, rubidium, and cesium chloride salts. The statistical errors were smaller than the size of the symbols used.
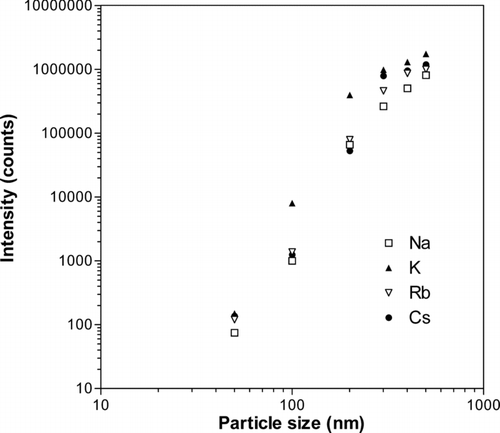
The detected number of ions is a small fraction of the original number of alkali atoms in each particle. We note that the intensity is sensitive to the detailed electrode setup and applied voltages, and this is in particular true for the geometry near the hot Pt surface. We have at the present stage not systematically varied the electrode setup around the Pt cup, and conclude that there is considerable potential for further improvement in sensitivity with a more optimized setup. The instrument already in its present state of development provides mass spectra for individual particles with sizes down to a few tens of nanometers, which should make it suitable for a number of applications.
As a first demonstration of the new AMS it was applied in ambient air measurements at the University of Gothenburg Campus. Gothenburg is located at 57.7°N 11.9°E on the west coast of Sweden and has a population of close to 600,000 people. The measurements were conducted from the Atmospheric Science laboratory at the Department of Chemistry. The laboratory is situated on the 4th floor and has access to a roof immediately outside the laboratory windows. The site is in close proximity to the downtown Gothenburg area and may be characterized as an urban background site. The sampled aerosol was directed to the inlet of the instrument through a 1 m long copper tube (i.d. 0.9 cm) on the outside of the wall, connected to 0.75 m of conducting tubing (i.d. 0.6 cm) inside the laboratory.
shows two examples of mass spectra recorded for individual ambient air particles on August 24, 2005. The mean temperature was about 15°C and wind speeds were below 7 ms–1 during the measurements. A particle size of 100 nm was selected with a DMA prior to the AMS. The spectrum displayed in the upper panel is a common type with a high K and low Na content. The K:Na ratio and the high alkali concentration indicate that the particle originates from biomass combustion. The second and less common type of spectrum (lower panel) is rich in Na and has a small K content, and has a K:Na ratio that indicates that it is a sea salt particle.
FIG. 6 Examples of mass spectra recorded for particles from ambient air with the AMS. Particles with a diameter of 100 nm were selected with a DMA prior to the AMS. The measurements were performed at the University of Gothenburg Campus on August 24, 2005.
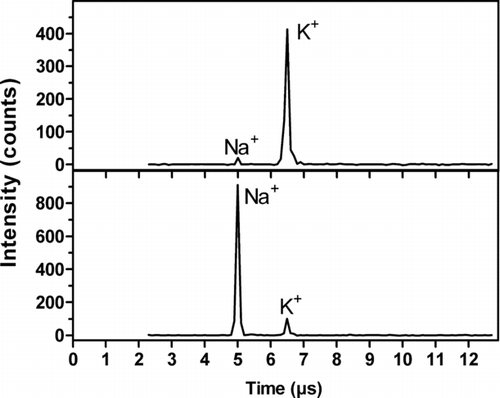
illustrates typical Na, K, Rb, and Cs signals observed when all particle sizes were allowed to enter the AMS during a short time period. Signals from individual particles are seen as sharp peaks in the time series and coincident signals for different alkali metals indicate that they originate from the same particle. Low Rb and Cs signals are associated with high Na and/or K signals. The sodium and potassium signals often, but not always, coincide indicating contributions from several sources to the particle-bound alkali concentrations.
FIG. 7 Na, K, Rb, and Cs concentrations as a function of time during 40 seconds of operation in ambient air for all particle sizes. The measurements were performed at the University of Gothenburg Campus on August 25, 2005.
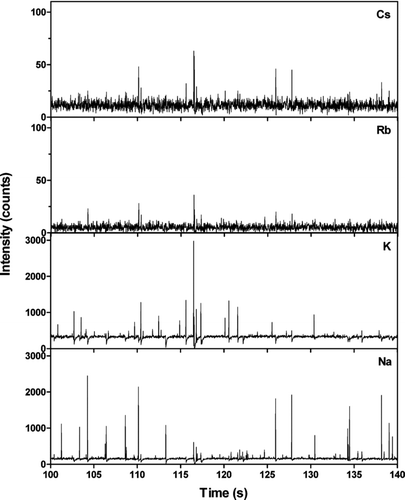
shows Na and K concentrations in individual particles during two episodes with distinctly different ambient conditions. All particle sizes where allowed to enter the instrument during the experiments. The data are displayed on both linear and logarithmic (inset) scales. Gothenburg experiences several ground based temperature inversion episodes every winter, which result in inefficient mixing and trapping of pollutants near ground level. During the night from February 20 to 21, low temperatures at ground level (from –5 to –8°C) and low wind speeds (below 2 ms–1) resulted in the development of a strong ground inversion. shows data from a four hour period starting at 04:00 in the morning of February 21. High number concentrations of alkali-containing particles were observed during this period and a majority of the particles have a high K:Na ratio. In addition to the K:Na ratio, one important characteristic of all particles displayed in is their relatively high alkali content, which limits the number of known sources considerably. The types of alkali-rich particles expected to be found include sea salt particles, particles produced by combustion of solid fuels such as biomass, coal and waste, mineral dust, and mixed particles of different types including one or more of the components mentioned before. A high K:Na ratio is typical for particles originating from biomass burning (CitationSvane et al. 2005b), and the data in indicate that such sources made a significant contribution to the polluted air in central Gothenburg. Biomass burning is used for domestic heating within the Gothenburg region, and a few large-scale district heating plants within the city are also using biomass fuel. The total number concentration of submicron particles is typically 104–105 cm–3 during inversion events and the observed alkali-containing particles are estimated to contribute with less than 5% of the total number concentration.
FIG. 8 The number of Na+ and K+ ion counts in individual particles. (a) Sampled from ambient air in Gothenburg from 04:00 to 08:00 on February 20, 2007. (b) Sampled from ambient air in Gothenburg from 15:52 to 19:52 on April 11, 2007. (c) Sampled from a laboratory setup used to mimic the formation of sea spray particles. All particle sizes where let into the AMS during the experiments. The insets show the same data on a logarithmic scale.
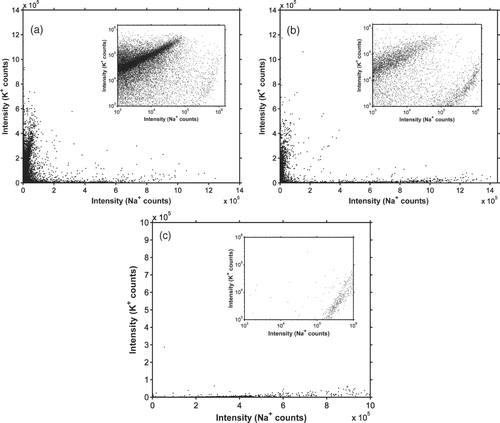
shows data taken during a four hour period starting at 15:52 on April 11 2007. The time period was characterized by westerly winds (1–11 ms–1) and temperatures of 8–12°C. A similar mode as the one attributed to biomass burning in is clearly observed also on this day, but the concentration is substantially lower than on February 21. A second group of particles with high Na and low K content is also seen in the data. The K:Na ratio in sea water is 0.036 (Kennish 2001), and the observed particles may correspond to the sea salt in sea spray particles. This is consistent with the relatively high wind speeds during the day and the wind direction from the nearby sea towards the city. The concentration of these particles was relatively low and may be estimated to correspond to less than 1% of the total number of submicron particles. This is consistent with what is known about the sea spray aerosol source: although it is the largest or second largest aerosol source globally, only challenged by dust, it is only expected to contribute with up to a few hundred particles per cubic centimeter (CitationNilsson et al. 2001), which would of course be overwhelmed by the much larger aerosol number sources in the city. Additional laboratory experiments were carried out with artificially generated sea salt particles similar to Mårtensson et al. (2003). Sea spray aerosol was generated at a water temperature of 12°C in a 200 l stainless steel tank filled with 100 l of surface sea water collected at the coastline outside Gothenburg on November 7, 2008 (57.3618° N; 11.5318° E). Air was entrained in the water by the impact of a recirculating vertical water jet with a flow rate of 10 l min–1, and the entrained air formed bubbles that burst to form sea spray aerosol. The method has been shown to form bubble and aerosol number spectra (Hultin et al., paper in preparation) and aerosol mass spectra (CitationFacchini et al. 2008), respectively, similar to bubble spectra found in the ocean and the part of ambient aerosol spectra that has been directly attributed to sea spray. It can therefore be considered a realistic artificial production of a full sea spray aerosol spectra, with the added advantages that the sea spray source is isolated, and that higher concentrations can be produced in the steel tank than what is found in the ambient air, corresponding to the production directly over a white cap at sea before the aerosol is diluted vertically and horizontally. illustrates the results obtained when particles produced in the tank were let into the AMS. The observed results are very similar to the data attributed to sea salt particles in , which supports the identification of this mode with sea salt particles.
To summarize, the short series of measurements in the laboratory and in ambient air show that the instrument is capable of on-line quantitative chemical analysis of individual particles. The high sensitivity combined with multi-element detection on the single particle level may make it possible to identify the source of alkali-containing submicron particles in the ambient atmosphere.
4. CONCLUDING REMARKS
A new type of AMS based on combined surface ionization and oa-TOFMS techniques has been described. The instrument is capable of detection and recording of complete alkali mass spectra for individual nanometer-size aerosol particles. Quantitative on-line measurements of the alkali content of single particles can be carried out for particles sizes in the range 50–500 nm, which should make the instrument suitable for a range of applications. The high sensitivity of the instrument indicates that it should be a useful tool in the development and characterization of other instruments. It should also be of use in other types of laboratory studies, e.g., studies of agglomeration processes involving alkali salt particles. Some of the major types of particles in the atmosphere have a substantial content of alkali compounds, including sea salt particles, mineral dust, and particles emitted during biomass burning and combustion of solid fuels like coal. The AMS should be ideal for on-line detection of these different types of particles, in particular since the new multi-element detection capability increases the possibility to attribute each detected particle to a given source. Field campaigns using this capability are currently under way.
One important aspect of the AMS design is the method chosen to determine the size of the detected particles. Particle sizing with light scattering techniques may be implemented in a similar way as used in laser desorption/ionization of particles (CitationGard et al. 1997). In the present setup implementation of light scattering for particle sizing would be an efficient way to size relatively large particles, while it would not be efficient for particle below 100 nm due to their limited reflectivity. Another opportunity is given by the fact that the velocity of particles leaving the aerodynamic lens system depends on particle size. A beam chopper may be used to measure the time-of-flight distribution of particles, and the particle size distribution is determined from the known relation between velocity and particle size. However, the use of a chopper introduces limitations to the experimental setup. The flight distance must be relatively long and the detector must have a response time of tens of microseconds or better to resolve the time-of-flight distribution. In addition, the chopper typically has a transmittance of a few percent. In the present study we instead use a DMA to determine the particle size prior to the AMS inlet. The transmission of this setup is comparable to the transmission expected for an instrument using a chopper for size selection, and the total complexity of the two instrument designs would be similar. The vacuum system of the AMS can be made considerably smaller when a DMA is used instead of a chopper, and one effect of a shorter flight path is an increased detection probability for particles smaller than 50 nm since the beam divergence is considerable for these particles. In addition, we do not have to design a hot surface where alkali-containing particles would have to completely disintegrate on the time scale of tens of microseconds.
There is considerable potential for further improvements of the instrument. The aim to develop a simple and easy-to-operate instrument for field measurements has been fulfilled, but the size of the present prototype can be significantly reduced by using components available on the market. The sensitivity may possibly be improved by up to a factor of 102–103 by optimization of the electrode setup near the ionizing filament. An ultimate version of the instrument should then be able to detect a few tens of alkali atoms in a single particle, and the lower detection limit would be set by the generation of background ions at the hot filament. As shown in earlier studies (Davies 1973; Davies 1977; CitationMyers et al. 1975; CitationStoffels et al. 1981), the use of other filament materials instead of platinum may increase the range of elements that can be detected by positive SI. In addition, negative SI could possibly be used to measure certain aerosol compounds, e.g., the nitrate content of individual particles.
Acknowledgments
We gratefully acknowledge discussions with Leif Holmlid and technical support by Benny Lönn and Agneta Fransson. This work was supported by the Swedish Research Council for Environment, Agricultural Sciences and Spatial Planning, the Swedish Research Council and the Swedish Radiation Protection Authority.
References
- Canagaratna , M. R. , Jayne , J. T. , Jimenez , J. L. , Allan , J. D. , Alfarra , M. R. , Zhang , Q. , Onasch , T. B. , Drewnick , F. , Coe , H. , Middlebrook , A. , Delia , A. , Williams , L. R. , Trimborn , A. M. , Northway , M. J. , DeCarlo , P. F. , Kolb , C. E. , Davidovits , P. and Worsnop , D. R. 2007 . Chemical and Microphysical Characterization of Ambient Aerosols with the Aerodyne Aerosol Mass Spectrometer . Mass Spectrom. Rev. , 26 : 185 – 222 .
- Coe , H. and Allan , J. D. 2006 . Mass Spectrometric Methods for Aerosol Composition Measurements, in Analytical Techniques for Atmospheric Measurement , 265 – 310 . Oxford : Blackwell Publishing .
- Davis , W. D. 1973 . Surface Ionization Mass Spectroscopy of Airborne Particulates . J. Vac. Sci. Technol. , 10 : 273
- Davis , W. D. 1977 . Continuous Mass Spectrometric Analysis of Particulates by Use of Surface Ionization . Environ. Sci. Technol. , 11 : 587 – 592 .
- Drewnick , F. , Hings , S. S. , DeCarlo , P. F. , Jayne , J. T. , Gonin , M. , Fuhrer , K. , Weimer , S. , Jimenez , J. L. , Demerjian , K. L. , Borrmann , S. and Worsnop , D. R. 2005 . A New Time-of-Flight Aerosol Mass Spectrometer (ToF-AMS)—Instrument Description and First Field Deployment . Aerosol Sci. Technol. , 39 : 637 – 658 .
- Facchini , M. C. , Rinaldi , M. , Decesari , S. , Carbone , C. , Finessi , E. , Mircea , M. , Fuzzi , S. , Ceburnis , D. , Flanagan , R. , Nilsson , E. D. , de Leeuw , G. , Martino , M. J. Woeltjen and O'Dowd , C. D. 2008 . Primary Submicron Marine Aerosol Dominated by Insoluble Organic Colloids and Aggregates . Geophys. Res. Lett. , 35 : L17814
- Gard , E. E. , Mayer , J. E. , Morrical , B. D. , Dienes , T. , Fergenson , D. P. and Prather , K. A. 1997 . Real-Time Analysis of Individual Atmospheric Aerosol Particles: Design and Performance of a Portable ATOFMS . Anal. Chem. , 69 : 4083 – 4091 .
- Guilhaus , M. , Selby , D. and Mlynski , V . 2000 . Orthogonal Acceleration Time-of-Flight Mass Spectrometry . Mass Spectrom. Rev. , 19 : 65 – 107 .
- Hultin , K. , Nilsson , E. D. , Krejci , R. , Mårtensson , E. M. , de Leeuw , G. and Ehn , M. 2008 . In situ laboratory sea spray microphysics during the MAP 2006 cruise on the North East Atlantic, manuscript in preparation .
- Ionov , N. I. 1972 . Progress in Surface Science , Edited by: Davison , S. G. Vol. 1 , Oxford : Pergamon Press .
- Jayne , J. T. , Leard , D. C. , Zhang , X. , Davidovits , P. , Smith , K. A. , Kolb , C. A. and Worsnop , D. R. 2000 . Development of an Aerosol Mass Spectrometer for Size and Composition Analysis of Submicron Particles . Aerosol Sci. Technol. , 33 : 49 – 70 .
- Jimenez , J. L. , Jayne , J. T. , Shi , Q. , Kolb , C. A. , Worsnop , D. R. , Yourshaw , I. , Seinfeld , J. H. , Flagan , R. C. , Zhang , X. , Smith , K. A. , Morris , J. W. and Davidovits , P. 2003 . Ambient Aerosol Sampling Using the Aerodyne Aerosol Mass Spectrometer . J. Geophys. Res. , 108 ( D7 ) : 8425 doi:10.1029/2001JD001213
- Johnston , M. V. 2000 . Sampling and Analysis of Individual Particles by Aerosol Mass Spectrometry . J. Mass. Spectrom. , 35 : 585 – 595 .
- Kennish , J. M. , ed. 2001 . Practical Handbook of Marine Science , 80 Boca Raton : CRC Press .
- Liu , P. , Ziemann , P. J. , Kittelson , D. B. and McMurry , P. H. 1995a . Generating Particle Beams of Controlled Dimensions and Divergence: I. Theory of Particle Motion in Aerodynamic Lenses and Nozzle Expansions . Aerosol Sci. Technol. , 22 : 292 – 313 .
- Liu , P. , Ziemann , P. J. , Kittelson , D. B. and McMurry , P. H. 1995b . Generating Particle Beams of Controlled Dimensions and Divergence: II. Experimental Evaluation of Particle Motion in Aerodynamic Lenses and Nozzle Expansions . Aerosol Sci. Technol. , 22 : 314 – 324 .
- Myers , R. L. and Fite , W. L. 1975 . Electrical Detection of Airborne Particulates Using Surface Ionization Techniques . Environ. Sci. Technol. , 9 : 334 – 336 .
- Mårtensson , E. M. , Nilsson , E. D. , de Leeuw , G. , Cohen , L. H. and Hansson , H.-C . 2003 . Laboratory Simulations and Parameterization of the Primary Marine Aerosol Production . J. Geophys. Res. , 108 : 4297 doi:10.1029/2002JD002263
- Nilsson , E. D. , Rannik , Ü , Swietlicki , E. , Leck , C. , Aalto , P. P. , Zhou , J. and Norman , M. 2001 . Turbulent Aerosol Fluxes Over the Arctic Ocean, Part 2, Wind Driven Sources from the Sea . J. Geophys. Res. , 106 : 32139 – 32154 .
- Noble , C. A. and Prather , K. A. 2000 . Real-Time Single Particle Mass Spectrometry: A Historical Review of a Quarter Century of the Chemical Analysis of Aerosols . Mass. Spectrom. Rev. , 19 : 248 – 274 .
- 2000 . SIMION (ION and electron optics SIMulation package), 3D version Idaho National Engineering and Environmental Laboratory .
- Sinha , M. P. and Friedlander , S. K. 1985 . Real-Time Measurement of Sodium Chloride in Individual Aerosol Particles by Mass Spectrometry . Anal. Chem. , 57 : 1880 – 1883 .
- Stoffels , J. J. and Lagergren , C. R. 1981 . On the Real-Time Measurement of Particles in Air by Direct Inlet Surface Ionization Mass Spectrometry . Int. J. Mass Spectrom. Ion Phys. , 40 : 243 – 254 .
- Sullivan , R. C. and Prather , K. A. 2005 . Recent Advances in Our Understanding of Atmospheric Chemistry and Climate made Possible by On-Line Aerosol Analysis Instrumentation . Anal. Chem. , 77 : 3861 – 3886 .
- Suess , D. T. and Prather , K. A. 1999 . Mass Spectrometry of Aerosols . Chem. Rev. , 99 : 3007 – 3035 .
- Svane , M. , Hagström , M. and Pettersson , J. B. C . 2004 . Chemical Analysis of Individual Alkali-Containing Aerosol Particles: Design and Performance of a Surface Ionization Particle Beam Mass Spectrometer . Aerosol Sci. Technol. , 38 : 655 – 663 .
- Svane , M. , Janhäll , S. , Hagström , M. , Hallquist , M. and Pettersson , J. B. C . 2005a . On-line Alkali Analysis of Individual Aerosol Particles in Urban Air . Atmos. Environ. , 39 : 6919 – 6930 .
- Svane , M. , Hagström , M. and Pettersson , J. B. C . 2005b . Online Measurements of Individual Alkali-Containing Particles Formed in Biomass and Coal Combustion: Demonstration of an Instrument Based on Surface Ionization Technique . Energy & Fuels. , 19 : 411 – 417 .
- Svane , M. , Hagström , M. , Davidsson , K. O. , Boman , J. and Pettersson , J. B. C. 2006 . Cesium as a Tracer For Alkali Processes in a Large-Scale Combustion Facility . Energy & Fuels. , 20 : 979 – 985 .
- Tobias , H. J. , Kooiman , P. M. , Docherty , K. S. and Ziemann , P. J. 2000 . Real-Time Chemical Analysis of Organic Aerosols Using a Thermal Desorption Particle Beam Mass Spectrometer . Aerosol Sci. Technol. , 33 : 170 – 190 .
- Trimborn , A. , Hinz , K.-P. and Spengler , B. 2000 . Online Analysis of Atmospheric Particles with a Transportable Laser Mass Spectrometer . Aerosol Sci. Technol. , 33 : 191 – 201 .
- Zandberg , E. Ya . 1995 . Surface Ionization Detection of Particles . Tech. Phys. , 40 : 865 – 884 .
- Zelenyuk , A. and Imre , D. 2005 . Single Particle Laser Ablation Time-of-Flight Mass Spectrometer: An Introduction to SPLAT . Aerosol Sci. Technol. , 39 : 554 – 568 .