Abstract
The charging efficiency function of the Electrical Low Pressure Impactor (ELPI) corona charger was estimated for spherical, cubical, and fractal particles having an electrical mobility diameter D b ranging from 0.01 to 0.5 μ m. Uncertainty analysis was performed on the experimental results for comparison with previous results for reference aerosols (spherical and cubical). Significant discrepancies were observed for the reference aerosols relative to Marjamäki et al. (Citation2000, Citation2002). For fractal particles, charging efficiency follows a power-law function; the coefficients show significant discrepancies with those estimated for the reference aerosols. Consequently, fractal particles present higher charging efficiency than reference particles with a similar electrical mobility diameter. Two approaches are proposed to estimate the equivalent charge diameter of particles denoting fractal morphology. The work of CitationRogak and Flagan (1992) provides the best agreement between the experimental results and the theoretical representation of the ELPI charging efficiency.
INTRODUCTION
Since its introduction, the Electrical Low Pressure Impactor (ELPI Dekati) has been widely used for measuring particulate emissions. CitationAhlvik et al. (1998), CitationMoisio (1999), CitationMaricq et al. (2000), and CitationVan Gulijk et al. (2004) recently used the ELPI for combustion aerosol characterization. In contrast with reference aerosols, combustion aerosols denote well-known fractal morphology (CitationJullien and Botet 1987). Few studies deal with the influence of this fractal shape on the ELPI response. Initial investigations were conducted by Matti CitationMaricq et al. (2000), CitationVirtanen et al. (2002), and CitationVan Gulijk et al. (2004), who compared combustion aerosol size distributions obtained using the ELPI and the Scanning Mobility Particle Sizer (SMPS). As a result of these studies, fractal theory is now used in aerosol metrology and the opportunities are numerous. Nevertheless, few studies deal with the influence of fractal morphology on the charging efficiency of the ELPIs. The available work has established the ELPI's charging efficiency for spherical and cubical particles (CitationKeskinen et al. 1992; CitationMarjamäki et al. 2000, Citation2002). Marjamäki et al. (Citation2000, Citation2002) reported no charging efficiency discrepancies between spherical and cubical particles, but more recently, CitationUnger et al. (2004) underlined major discrepancies between spherical and cubical particles for unipolar charging in a corona wire charger.
Regarding fractal particles, numerous theoretical studies are available on bipolar charging (CitationWen et al. 1984; CitationRogak and Flagan 1992; CitationLall et al. 2006) and unipolar charging (CitationChang 1981; CitationHan and Gentry 1993; CitationBiskos et al. 2004); nevertheless, to our knowledge, the only study dealing with experimental measurement of charging efficiency using a corona charger for fractal particles is the work of CitationNtziachristos et al. (2004). The authors underlined the significant discrepancy between the charging efficiency of reference aerosols and combustion aerosols for the “filter-stage” configuration of the ELPI corona charger: the results agree with the work of CitationOh et al. (2004). More recently, CitationBiskos et al. (2005) studied unipolar charging of combustion aerosols in a corona charger and, in contrast to Ntziatchristos et al. (2004), described good agreement between combustion and reference aerosol charging efficiency and with the theoretical approach of CitationFuchs (1963).
In the present work, two distinct aspects have been considered: uncertainty analysis of the charging efficiency function for reference aerosols and the influence of fractal morphology on the charging efficiency of the ELPI corona charger. The experimental procedure, similar to CitationMarjamäki et al. (2000), was first used for cubical, spheroid, and spherical aerosols, then for fractal particles generated by a GFG-1000 PALAS spark discharge generator (CitationEvans et al. 2003) and by acetylene gas and polymer diffusion flames (CitationOuf et al. 2008).
ELECTRICAL LOW PRESSURE IMPACTOR AND CORONA CHARGING
A. ELPI Principle
Since the ELPI granulometer has been widely described in previous publications (CitationKeskinen et al. 1992; CitationMoisio 1999; CitationMarjamäki et al. 2000, Citation2002, Citation2005; CitationLettemy 2005), we will provide only a brief description of how it works. The ELPI combines a low-pressure impactor with a corona charger. At the inlet of the ELPI, a corona charger imposes an electrical charging state on the particles composing the aerosol. At the charger's outlet, the remaining ions are trapped by an electrostatic precipitator and the particles are classified according to their aerodynamic diameter using a low pressure cascade impactor. Currents induced by particles collected on impaction stages are measured using electrometers and are converted, with the help of the charging efficiency function, into particle number concentration.
B. Principle, Description, and Characteristics of the Corona Charger
The principle of the ELPI corona charger is described in (CitationDekati Ltd., 2005). First, an ionization field is generated by applying 5000 V (1 μ A in current) to a wire 100 μ m in diameter. Particles flowing through this ionization field acquire electrical charges by diffusion and field charging. At the outlet of the corona charger, non-fixed ions are electrically precipitated using a trap voltage of 400 V. The averaged characteristics of the corona charger are derived from CitationMoisio (1999):
• | intensity of field charging E: 1.67.105 V/m, | ||||
• | ion density in field charging Ni: 1.9.1014 #/m3, | ||||
• | field charging volume Vcharging: 7.3.10–5 m3 = 73 cm3, | ||||
• | sampling flow rate Q: 1.65.10–4 m3/s = 165 cm3/s, | ||||
• | residence time in the charging zone t = Vcharging/Q: 0.44 s, | ||||
• | radius of the corona charger and outer radius of the ion trap r = router: 3 cm, | ||||
• | ion trap voltage U: 400 V, | ||||
• | length of ion trap L: 1.5 cm, | ||||
• | inner radius of the ion trap rinner: 2.65 cm |
For a given size range, the conversion between measured current I and particle number concentration C is defined as Ech:
THEORETICAL CONSIDERATIONS
We present the different relations used for the analysis and after-treatment (see Appendix II) of our experimental results. The specific design of the corona charger imposes two electrical charging particle phenomena, the first by unipolar ion diffusion and the second by field charging. The first phenomenon results from collisions between unipolar ions generated by the corona charger and particles flowing through this charger. The number of charges that a spherical particle acquires by unipolar diffusion as a function of residence time has been theoretically summarized by CitationHinds (1999), Baron and Willeke (2000), and more recently CitationKleefsman and Van Gulijk (2008):
-
ϵ0: vacuum permittivity: 8.85.10–12 C2/N.m2,
-
Dp: particle diameter if particles are assumed spherical (m),
-
k: Boltzmann constant: 1.380658.10–23 J/K,
-
T: temperature (K),
-
e: charge of an electron: 1.6.10–19 C,
-
: mean velocity of ions: 240 m/s.
The second charging phenomenon encountered in the ELPI is field charging. This phenomenon corresponds to the charging of particles by unipolar ions in a strong electrical field. The number of charges that a particle acquires by field charging was also reported by CitationHinds (1999):
-
ϵp: relative permittivity of the particle,
-
Zi: electrical mobility of ions: 1.5.10–4 m2/V.
The global charging of the corona charger can be approximated as the sum of the previously described charges induced by diffusion and field charging:
Charging efficiency, as described in Equation (Equation2), also integrates particle losses inside the corona charger. Two loss mechanisms were identified, one in the charging zone and the other in the ion trap. For losses in the charging zone, CitationHinds (1999) reported using a numerical approach based on the Deutsch-Anderson equation for penetration coefficient estimation Pcharging(Dp,ϵp t):
-
λ : mean free path (66.5 nm for air at 293.15 °K and 101.3 kPa),
-
μ : dynamic viscosity (1.833.10−5 Pa.s for air at 293.15 °K and 101.3 kPa).
One must notice that the Deustch-Anderson equation assumes that particles acquire their charge as soon as they enter the corona charger. For losses inside the ion trap, the penetration coefficient Ptrap(Dp,ϵp t) is estimated assuming a perpendicular field inside a symmetrical, cylindrical tube (CitationHinds 1999):
MEASUREMENTS
Aerosol Generation
This section describes the type and shape of the aerosol studied. First, for reference aerosols we used a TSI 3076 Constant Output Atomizer with four different solutions:
• | liquid spherical particles of diethyl sebacate (DES) and diethyl phtalate (DEP), | ||||
• | solid cubical particles of sodium chloride (NaCl) and spheroid particles of cesium chloride (CsCl). |
Solid particle shapes were investigated using transmission electron microscopy (TEM) and are detailed in . The cubical shape of NaCl and the intermediate spheroid appearance of CsCl are in good agreement with the previous results of CitationWise et al. (2005). gives the particle characteristics of aerosols produced with the TSI atomizer.
FIG. 1 TEM micrographs of solid particles generated by PALAS GFG 1000, acetylene, and PMMA fuels, and TSI 3076 Constant Output Atomizer for NaCl and CsCl solutions.

TABLE 1 Physical characteristics of reference aerosol particles
Carbon aggregates were generated using a PALAS GFG 1000 under the following experimental conditions:
• | 1.5 bar of argon, | ||||
• | spark frequency: 270 Hz. |
The aggregates produced were mainly composed of graphitic carbon with an assumed density of 2.2 g/cm3 and a dielectric constant ranging from 12 to 15. The recent work of CitationEvans et al. (2003) and CitationNaumann (2003) describes these particles as fractal nanoparticle aggregates with a fractal dimension near 2 and primary particle diameter of 7.4 nm. shows transmission electron microscopy (TEM) images of these particles.
For the combustion aerosol, two fuels were used in this study: a gas (acetylene, C2H2) and a solid polymer (polymethyl methacrylate, PMMA, C5H8O2). A detailed study of the combustion aerosol properties produced of these two fuels is available in the recent work of CitationOuf et al. (2008). provides the global characteristics of soot particles produced during acetylene and PMMA combustion in our experimental conditions.
TABLE 2 Physical characteristics of fractal aggregates studied
presents TEM micrograph examples of soot particles produced by acetylene and PMMA. Size distributions of the seven different aerosols were established using a scanning mobility particle sizer (DMA TSI model 3081, CPC TSI model 3022A). All the size distributions were fitted with a log-normal distribution:

TABLE 3 Characteristics of aerosol size distributions
Charging Efficiency Determination
presents the experimental device used to determine the charging efficiency of spherical, spheroid, cubical, and fractal particles respectively produced using the TSI atomizer, the GFG 1000, or the acetylene and PMMA flames (CitationOuf et al. 2008).
FIG. 2 Experimental device for determining the corona charging efficiency of spherical, spheroid, cubical, and fractal aggregates (ELPI corona charger principle taken from CitationDekati Ltd., 2005).
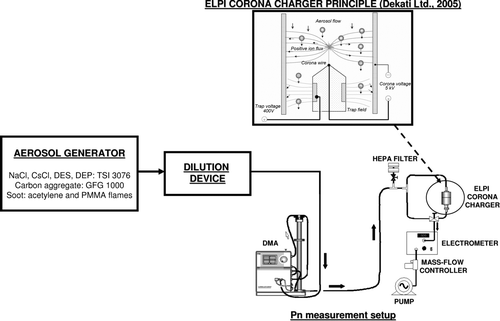
Both experimental set-ups used generation devices (atomizer, GFG 1000, or diffusion flame) and dilution devices (dilution tube or FPS 4000). Particles were then classified according to their electrical mobility using a Kr-85 radiation source (TSI 3077) and an electroclassifier (TSI EC 3080) with a long DMA column (TSI 3081) allowing mobility diameter selection from 10 to 1000 nm. At the DMA outlet, the aerosol was diluted with filtered air to reach the ELPI nominal flow rate of 10 LPM. The current induced by the charged particles was then measured simultaneously at the inlet Iinlet and outlet Ioutlet of the ELPI corona charger, using a TSI 3068 electrometer. The charging efficiency Pn is thus defined as:
It is experimentally impossible to generate monodisperse soot particles in a representative way. We have chose to use both synthetic (GFG 1000) and realistic carbon aggregates (acetylene and PMMA flames). These generation processes tend to produce aerosols denoting highly polydisperse size distributions. According to this dispersion in terms of size, the multiple charge effect on the selected electrical mobility diameter must be taken into account, and the results presented below are corrected using the method detailed in Appendix II.
RESULTS AND DISCUSSION
Comparison with Previous Results
The ELPI's charging efficiency was first experimentally determined by CitationKeskinen et al. (1992). More recently, it was determined by CitationMarjamäki et al. (2000), using a similar procedure. The generation procedure was similar in both studies and is based on a Condensation-Evaporation Monodisperse Aerosol Generator with an electrical mobility diameter lower than 1 μ m. For CitationKeskinen et al. (1992), the charging efficiency was determined by comparing the number concentration measurement at the corona charger's inlet using a condensation particle counter, with the current measured at the outlet using an electrometer. CitationMarjamäki et al. (2000) modified this procedure and directly compared currents measured at the outlet and the inlet of the corona charger. By using this second approach and only one measuring device (in this case an electrometer), experimental uncertainty is reduced. For particles with a mobility diameter higher than 1 μ m, a Vibrating Orifice Aerosol Generator was used by CitationMarjamäki et al. (2000) and charging efficiency was estimated by comparing number concentration at the corona charger's inlet using an Aerodynamic Particle Sizer (APS) with the current measured at the outlet by an electrometer. Using this experimental procedure, CitationMarjamäki et al. (2000) proposed a general expression of the charging efficiency Pn, for spherical dioctyl sebacate particles and cubical sodium chloride particles:
FIG. 3 Charging efficiency of reference aerosol measured for “trap on” (black dots and curve) and “filter stage” (grey dots and curve) configurations.
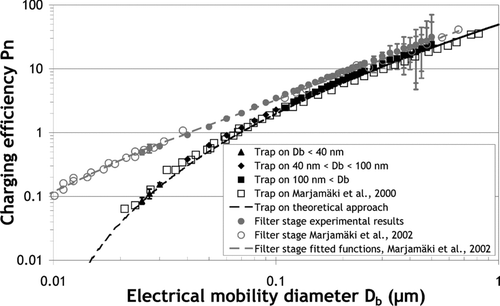
We compared our results with the theoretical approach (Equation [11]) introduced in the third part of this study and the previous experimental results of CitationMarjamäki et al. (2000). Overall, our results agree with the theoretical approach, but our results are significantly higher than the theoretical charging efficiency. The charging efficiency determined by CitationMarjamäki et al. (2000) is also in overall agreement with the theoretical model, but in this case experimental charging efficiency appears to be lower than the theoretical approach. An overall discrepancy of 10% for Db < 0.1 μ m and 25% for Db < 0.5 μ m can be observed between the present work and the previous results of CitationMarjamäki et al. (2000). Similar to CitationMarjamäki et al. (2000), presents the three size ranges considered for fitting the experimental results: below 40 nm, between 40 and 100 nm, and above 100 nm. One way of explaining the discrepancies observed between our results and those of CitationMarjamäki et al. (2000) is that there might be some discrepancies in the characteristics of ELPI corona charger used in the present study and in the work of CitationMarjamäki et al. (2000). To our knowledge there is no publication dealing with the comparison of several ELPI corona charger's charging efficiency.
A widely used ELPI configuration is the 0V ion-trap configuration. Given the ion trap's cut-off diameter of 25 nm (CitationMarjamäki et al. 2000), this device must be disabled for the “filter stage” configuration which allows decreasing the lowest diameter measured by the ELPI from 30 to 8 nm. Measurements were performed by CitationMarjamäki et al. (2002) and charging efficiency functions were introduced:
To present the discrepancies between our experimental results and CitationMarjamäki et al. (2000), we developed discrepancy functions comparing experimental and theoretical charging efficiencies:
Estimation of the Uncertainties Associated with the Charging Efficiency Function
Based on this set of experimental results, we propose new power-law charging efficiency functions. Two distinct approaches were considered; the first is similar to Marjamäki et al. (Citation2000, Citation2002) and does not integrate the uncertainties associated with each experimental result. The second approach involves a charging efficiency function weighted by the uncertainty associated with each experimental result. The main assumption of a fitting procedure is that the parameter associated with the X-axis is a well-known parameter with the smallest uncertainty. According to Mulholland et al. (1999, 2006) and CitationEhara et al. (2000), the uncertainty associated with measuring the electrical mobility diameter Db using a DMA is nearly 5%. This uncertainty is mainly due to uncertainties on DMA voltage, DMA flow-rates and air characteristics at the experimental conditions (CitationMulholland et al. 2006). Since this uncertainty is small compared to the experimental uncertainty for Pn, we have associated Db with the X-axis. The main advantage of this approach is that it integrates a complete computation of the uncertainty of the fitting function parameters. More information on determining the power-law fitting uncertainty can be found in Appendix I.C. Power-law functions were considered for the entire size range (Db in μ m):
presents the fitting parameters obtained without and with uncertainty weighting (α, β and α′, β′ respectively) for “trap on” and “filter stage” configurations. Uncertainties associated with these parameters (σα′ and σβ′) are also reported in this table. According to the fitting procedure, the overlap of the fitted functions must be taken into account. Fitted functions are obtained from the experimental results but do not always intercept exactly at the limits of the experimental size range. In our case, charging efficiency is defined as a power-law function of the electrical mobility diameter (see Equation [18]). Consequently, the overlapping diameter is defined by the following equation:
• | discrepancies between weighted and non-weighted parameters:
| ||||
• | discrepancies between the non-weighted parameters of Marjamäki et al. (Citation2000, Citation2002) and the weighted parameters of the present study:
|
TABLE 4 Fitting parameters for “trap on” and “filter stage” configurations for reference aerosols
TABLE 5 Discrepancies between weighted and non-weighted fitting parameters for “trap on” and “filter stage” configurations
Note that the discrepancies between weighted and non-weighted fitting parameters are lower than 4% for all size ranges. We can thus assume that the experimental uncertainties have little impact on the procedure for fitting the experimental results. However, there is a large discrepancy between the present fitted functions and those proposed by Marjamäki et al. (Citation2000, Citation2002). This discrepancy is particularly significant for the α parameter in the size range Db > 0.1 μ m. In both the “trap on” and “filter stage” configurations, significant discrepancies were observed between the present fitted functions and those of Marjamäki et al. (Citation2000, Citation2002). These discrepancies are less pronounced for the “filter stage” configuration. For the “trap on” configuration, a significant gap is observed for the particles denoting an electrical mobility diameter larger than 0.1 μ m.
Influence of Particle Shape on Charging Efficiency
The second part of this experimental study deals with the influence of particle shape on the corona charging efficiency. shows the charging efficiency of “trap on” and “filter stage” configuration measured for fractal aerosols generated by the GFG 1000 and during acetylene and PMMA combustion.
FIG. 5 Charging efficiency of fractal particles for “trap on” (black dots and curve) and “filter stage” (grey dots and curve) configurations.
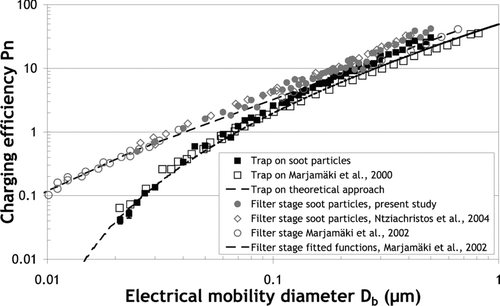
For the “trap on” configuration and for particles with electrical mobility diameter larger than 0.1 μ m, the charging efficiency obtained for fractal aggregates appears to be significantly higher than the charging efficiency of a sphere or cube with the same electrical mobility diameter. This higher charging efficiency (25% for the biggest particles) is in good agreement with the experimental results of CitationOh et al. (2004), which show that aggregates with low fractal dimension can carry 30% more charge than compact particles. It should be noted that charging efficiency is similar for all fuels which produce soot composed of primary particle with different diameters (7.4 nm for GFG 1000 and 64 nm for the acetylene diffusion flame). For the “filter stage” configuration, the charging efficiency of soot particles is also higher than for compact particles and the present results are in good agreement with the work of CitationNtziachristos et al. (2004) on diesel particles. Nevertheless, while discrepancies are observed between fractal and compact particles, the charging efficiency of fractal particles can be represented by a power-law function, as it can for spherical or cubical particles. In , we propose new fitting parameters, α and β, of a power-law function for fractal aggregates. There is a significant discrepancy between the power-law coefficients for fractal particles and the same coefficients for reference aerosols (cf. ).
TABLE 6 Fitting parameters for “trap on” and “filter stage” configurations for fractal aggregates
Influence of the Fractal Morphology of Soot Particles on Charging Efficiency
Experimental results obtained for fractal aggregates shown that the charging efficiency of these particles is higher than for spherical or cubical particles with the same electrical mobility diameter. Furthermore, in the case of bipolar diffusion charging, CitationRogak and Flagan (1992) have established a link between equivalent charge diameter Dqe and electrical mobility diameter for fractal aggregates of TiO2:
FIG. 6 Charging efficiency of fractal particles for “trap on” configuration as a function of equivalent charge diameter.
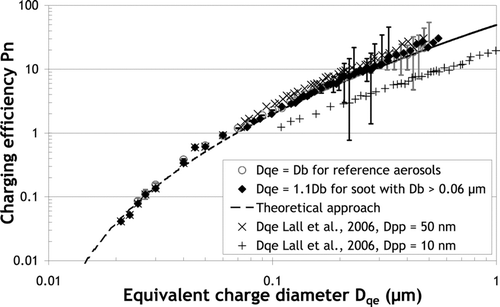
FIG. 7 Charging efficiency of fractal particles for “filter stage” configuration as a function of equivalent charge diameter.
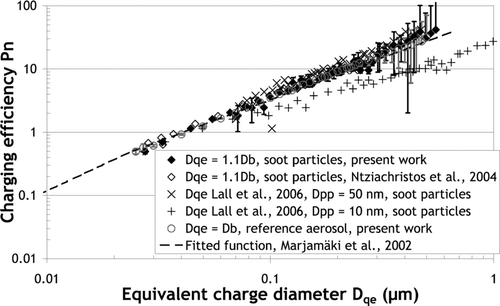
Regarding equivalent charge diameter, better agreement between reference aerosols and fractal aggregates was observed with the empirical approach developed by CitationRogak and Flagan (1992). Furthermore, the Lall and Friedlander approach (2006) does not reduce the discrepancy between the fractal and theoretical charging efficiencies.
CONCLUSIONS
To our knowledge, this study represents the first uncertainty analysis of the ELPI charging efficiency function. The experimental procedure for charging efficiency described by Marjamäki et al. (Citation2000, Citation2002) was applied to spherical, spheroid, and cubical reference aerosols. Our experimental results underline a significant discrepancy with Marjamäki et al. (Citation2000, Citation2002) that may be explained by some discrepancies between ELPI corona charger used in the present study and in the work of Marjamäki et al. (Citation2000, Citation2002). We developed new charging efficiency functions and performed a detailed uncertainty analysis. The general shape of the charging efficiency is similar to previous work and can be approximated with a power-law function:
The second part of this study demonstrated the influence of the fractal shape of nanoparticle agglomerates and combustion aerosols on the ELPI charging efficiency. The same experimental procedure to determine the charging law was applied to nanoparticle agglomerates of carbon produced by a PALAS GFG-1000 and to fractal aggregates generated during acetylene and PMMA combustion. A significant discrepancy in charging efficiency was observed for fractal aggregates and reference aerosols with the same electrical mobility diameter. Using the theoretical approach developed by CitationRogak and Flagan (1992), this discrepancy can be reduced if charging efficiency is considered as a function of the charge equivalent diameter:
APPENDIX I: UNCERTAINTY ANALYSIS
A. Charging Efficiency Uncertainty
The uncertainty for charging efficiency was determined using the following definition:
The uncertainties associated with Iinlet and Ioutlet can be determined from the definitions of these currents and based on Equation (Equation13). Since the definitions of Iinlet and Ioutlet are similar, we only described the uncertainty associated with Iinlet;
B. Weighted Mean Value and Associated Uncertainty
From the set of experimental results, one can compute mean values weighted with the uncertainty associated with each value. Considering xi values obtained in the same experimental conditions and σi experimental uncertainties associated with these values, the uncertainty σ and the weighted mean value
of this series of n measurements of x are defined as:
C. Weighted Fit Function of Charging Efficiency and Associated Uncertainty
Using the experimental results, fitting functions can be established that take into account the uncertainties associated with the experimental results. The present method is reliable for linear fitting; consequently, we considered the charging efficiency functions in a log-normal scale, then used Equation (Equation18) to introduce the following relationship:
Where is the mean value of x (or ln(Db)),
is the mean value of y (or ln(Pn)), and σ (y) is the experimental uncertainty associated with the considered value of y. For the slope σ (β) and the origin σ (ln(α)) of the fit applied to Nb experimental values of y, the associated uncertainties are defined as:
APPENDIX II: MULTIPLE CHARGES CORRECTION
Due to the aerosol generation procedure and the DMA classification, multiple charged particles may interfere with the measurement of charging efficiency. By definition, electrical mobility Zp(n,Dbn) depends both on particle diameter Dbn and the number of charges n carried by the particle. Thus, when an electrical mobility Zp(n,Dbn) is selected using a DMA, the aerosol at the outlet of the DMA is composed of particles with different diameters and charging states. We only included four charging states and the response of the electrometer at the DMA outlet can be defined as:
First, the current induced by particles of electrical mobility Zp(n,Dbn) at the ELPI corona charger's outlet must be defined. For this configuration, the current is a convolution of the aerosol size distribution N(Db), the probability that a particle can carry n charges Φ (n,Db), and the DMA transfer function Ω (Zp(n,Dbn)):
In the same way, the current measured at the outlet of the ELPI corona charger can be defined as a convolution of the aerosol size distribution N(Db), the probability that a particle can carry n charges Φ (n,Db), the DMA transfer function Ω(Z p(n,Dbn)), and the charging efficiency of the corona charger Pn(Db):
In order to correct the currents experimentally measured for each electrical mobility diameter at the inlet and outlet of the corona charger, correction factors can be introduced:
Notes
*From Ouf et al. 2008.
***Theoretical hypothesis from Nauman (2003).
**From Nauman 2003.
REFERENCES
- Ahlvik , P. , Ntziachristos , L. , Keskinen , J. and Virtanen , A. 1998 . Real Time Measurements of Diesel Particle Size Distributions with an Electrical Low Pressure Impactor SAE Paper. 980410
- Baron , P. A. and Willeke , K. 2001 . Aerosol Measurement—Principles, Techniques And Applications, , Second Edition , New York : John Wiley & Sons .
- Biskos , G. , Mastorakos , E. and Collings , N. 2004 . Monte-Carlo Simulation of Unipolar Diffusion Charging for Spherical and Non-Spherical Particles . J. Aerosol Sci. , 35 : 707 – 730 .
- Biskos , G. , Reavell , K. and Collings , N. 2005 . Unipolar Diffusion Charging of Aerosol Particles in the Transition Regime . J. Aerosol Sci. , 36 : 247 – 265 .
- Chan , P. and Dahneke , B. 1981 . Free-Molecule Drag on Straight Chains of Uniform Spheres . J. Applied Phys. , 52 : 3106 – 3110 .
- Chang , J. S. 1981 . Theory of Diffusion Charging of Arbitrarily Shaped Conductive Aerosol Particles by Unipolar Ions . J. Aerosol Sci. , 12 : 19 – 26 .
- Dahneke , B. 1982 . Viscous Resistance of Straight-Chain Aggregates of Uniform Spheres . Aerosol Sci. Technol. , 1 : 179 – 185 .
- Dekati Ltd. 2005 . ELPI User Manual v4.0
- Ehara , K. , Mulholland , G. W. and Hagwood , R. C. 2000 . Determination of Arbitrary Moments of Aerosol Size Distributions from Measurements with a Differential Mobility Analyzer . Aerosol Sci. Technol. , 32 : 434 – 452 .
- Evans , D. E. , Harrison , R. M. and Ayres , J. G. 2003 . The Generation and Characterisation of Elemental Carbon Aerosols for Human Challenge Studies . J. Aerosol Sci. , 34 : 1023 – 1041 .
- Fuchs , N. A. 1963 . On the Stationary Charge Distribution on Aerosol Particles in a Bipolar Ionic Atmosphere . Geofisica Pura e Applicata. , 56 : 185 – 193 .
- Hagwood , C. , Sivathanu , Y. and Mulholland , G. 1999 . The DMA Transfer Function with Brownian Motion: A Trajectory/Monte-Carlo Approach . Aerosol Sci. Technol. , 30 : 40 – 61 .
- Han , R. J. and Gentry , J. W. 1993 . Field and Combined Diffusional and Field Charging of Fibrous Aerosols . Aerosol Sci. Technol. , 18 : 165 – 179 .
- Hinds , W. C. 1999 . Aerosol Technology: Properties, Behaviour, and Measurement of Airborne Particles. , Wiley-Interscience .
- Ji , J. H. , Bae , G. N. and Hwang , J. H. 2004 . Characteristics of Aerosol Charge Neutralizers for Highly Charged Particles . Aerosol Sci. Technol. , 35 : 1347 – 1358 .
- Jullien , R. and Botet , R. 1987 . Aggregation and Fractal Aggregates. , World Scientific Pub Co Inc .
- Kasper , G. 1982 . Dynamics and Measurement of Smokes. II. The Aerodynamic Diameter of Chain Aggregates in the Transition Regime . Aerosol Sci. Technol. , 1 : 201 – 215 .
- Kleefsman , W. A. and Van Gulijk , C. 2008 . Robust Method to Compare Aerosol Chargers . J. Aerosol Sci. , 39 : 1 – 9 .
- Keskinen , J. , Pietarinen , K. and Lehtimäki , M. 1992 . Electrical Low Pressure Impactor . J. Aerosol Sci. , 23 : 353 – 360 .
- Lall , A. A. and Friedlander , S. K. 2006 . On–Line Measurement of UltraFine Aggregate Surface Area and Volume Distributions by Electrical Mobility Analysis: I. Theoretical Analysis . J. Aerosol Sci. , 37 : 260 – 271 .
- Lettemy , M. , Marjamäki , M. and Keskinen , J. 2005 . The ELPI Response and Data Reduction II: Properties of Kernels and Data Inversion . Aerosol Sci. Technol. , 39 : 583 – 595 .
- Maricq , M. , Podsiadlik , D. and Chase , R. 2000 . Size Distributions of Motor Vehicle Exhaust PM: A Comparison Between ELPI and SMPS Measurements . Aerosol Sci. Technol. , 33 : 239 – 260 .
- Marjamäki , M. , Keskinen , J. , Chen , D.-R. and Pui , D. Y. H. 2000 . Performance Evaluation of the Electrical Low Pressure Impactor (ELPI) . J. Aerosol Sci. , 31 : 249 – 261 .
- Marjamäki , M. , Ntziachristos , L. , Virtanen , A. , Ristimäki , J. , Keskinen , J. , Moisio , M. and Palonen , M. 2002 . Electrical filter stage for the ELPI SAE Paper. 2002–01–0055
- Marjamäki , M. , Lemmety , M. and Keskinen , J. 2005 . ELPI Response and Data Reduction I: Response Functions . Aerosol Sci. Technol. , 39 : 575 – 582 .
- Moisio , M. 1999 . Real time size distribution measurement of combustion aerosols. , Ph.D. Thesis Tampere University of Technology publications 279, Tampere, Finland
- Mulholland , G. W. , Bryner , N. P. and Croarkin , C. 1999 . Measurement of the 100 nm NIST SRM 1963 by Differential Mobility Analysis . Aerosol Sci. Technol. , 31 : 39 – 55 .
- Mulholland , G. W. , Donnelly , M. K. , Hagwood , C. R. , Kubuck , S. R. and Hackley , V. A. 2006 . Measurement of 100 nm and 60 nm Particle Standardsby Differential Mobility Analysis . J. Res. Nat. Inst. Stand. Technol. , 111 : 257 – 312 .
- Naumann , K. H. 2003 . COSIMA—A Computer Program Simulating the Dynamics of Fractal Aerosols . J. Aerosol Sci. , 34 : 1371 – 1397 .
- Neuilly , M. and CETAMA . 1998 . Modélisation et estimation des erreurs de mesure. , Lavoisier .
- Ntziachristos , L. , Giechaskiel , B. , Ristimäki , J. and Keskinen , J. 2004 . Use of a Corona Charger for the Characterisation of Automotive Exhaust Aerosol . J. Aerosol Sci. , 35 : 943 – 963 .
- Oh , H. , Park , H. and Kim , S. 2004 . Effects of Particle Shape on the Unipolar Diffusion Charging of Nonspherical Particles . Aerosol Sci. Technol. , 38 : 1045 – 1053 .
- Ouf , F. X. , Vendel , J. , Coppalle , A. , Weill , M. and Yon , J. 2008 . Characterization of Soot Particles in the Plumes of Over-Ventilated Diffusion Flames . Comb. Sci. Technol. , 180–4 : 674 – 698 .
- Rogak , S. N. and Flagan , R. C. 1992 . Bipolar Diffusion Charging of Spheres and Agglomerate Aerosol Particles . J. Aerosol Sci. , 23 : 693 – 710 .
- Stolzenburg , M. R. 1988 . An ultrafine aerosol size distribution measuring system. , Ph.D. Thesis Minneapolis : University of Minnesota .
- Unger , L. , Boulaud , D. and Borra , J. P. 2004 . Unipolar Field Charging of Particles by Electrical Discharge: Effect of Particle Shape . J. Aerosol Sci. , 35 : 965 – 979 .
- Van Gulijk , C. , Marijnissen , J. C. M. , Makee , M. , Mouljin , J. A. and Schmidt-Ott , A. 2004 . Measuring Soot with a Scanning Mobility Particle Sizer and an Electrical Low Pressure Impactor: Performance Assessment with a Model for Fractal-Like Agglomerates . J. Aerosol Sci. , 35 : 633 – 655 .
- Virtanen , A. , Ristimäki , J. , Marjamäki , M. , Vaaraslahti , K. and Keskinen , J. 2002 . Effective Density of Diesel Exhaust Particles as a Function of Size SAE Paper. 129–134
- Wen , H. Y. , Reischl , G. P. and Kasper , G. 1984a . Bipolar Diffusion Charging of Fibrous Aerosol Particles—I. Charging Theory . J. Aerosol Sci. , 15 : 89 – 101 .
- Wen , H. Y. , Reischl , G. P. and Kasper , G. 1984b . Bipolar Diffusion Charging of Fibrous Aerosol Particles—II. Charge and Electrical Mobility Measurements on Linear Chain Aggregates . J. Aerosol Sci. , 15 : 103 – 122 .
- Wiedensohler , A. 1988 . An Approximation of the Bipolar Charge Distribution for Particles in the Submicron Size Range . J. Aerosol Sci. , 19 : 387 – 389 .
- Wise , M. E. , Biskos , G. , Martin , S. T. , Russel , L. M. and Buseck , P. R. 2005 . Phase Transition of Single Salt Particles Studied Using a Transmission Electron Microscope with an Environmental Cell . Aerosol Sci. Technol. , 39 : 849 – 856 .