Abstract
Recent studies suggest that inhaled or intratracheally instilled multi-walled carbon nanotubes (MWCNTs) cause adverse health effects depending on the fiber length. In the present study a simple batch particle generation system was developed to generate airborne MWCNTs for inhalational toxicology studies. The generation rate can be controlled by the amplitude of sieve shaker. Maximum concentration of respirable airborne MWCNTs was 1.2 mg m–3 at a nose-exposure chamber supplied with air at flow rate of 30 L min–1. We examined the performance of airborne MWCNT generation system and characterized properties of generated fibrous particles at mass concentrations of 0.4 mg m–3 (particle number; ca. 1700 cm–3). Monomodal shaped size distributions with peak located at electrical mobility diameter of 300 nm (in number) and aerodynamic diameter of 1–2 μm (in mass) were measured with a scanning mobility particle sizer and with a low-pressure impactor, respectively. Two hour particle generation reproducibility tests were conducted five times, in which stability and repeatability of particle size and total number concentration were within an acceptable range. Aerodynamically classified particle morphology was studied by TEM, dissociated fiber-like and agglomerated MWCNT particles were observed. The former contributes up to 38% to counted particles, and the average width and length of fiber were 80 nm and 3.7 μm, respectively, with an aerodynamic size for particle of 260–381 nm.
1. INTRODUCTION
Recently, engineered nanomaterials have been attracting attention due to their potential benefits, and it is expected that the production of nanomaterials will increase greatly. Carbon nanotubes (CNTs) are one of the engineered nanomaterials which provide considerable potential benefits in the electronics, automotive, and construction industries due to their high electrical conductivity, low density, and high strength. However, there is also a concern about adverse health effects by CNTs when inhaled. Therefore, exposure and toxicity studies of CNTs are needed to assess the health risk related to inhalation of CNTs. CitationMaynard et al. (2004) conducted an exposure study during handling of CNT as a regular work task; CNT material was removed from production vessels and handled prior to processing. They reported that the estimated airborne concentration of CNT materials generated during handling was lower than 53 μg m–3. Recently CitationHan et al. (2008) monitored exposure in a multi-walled carbon nanotube (MWCNT) research facility, and found that concentration of airborne MWCNTs ranged from 172.9 to 193.6 cm–3 in the blending laboratory. Even though the mass concentration of airborne CNTs is low in occupational settings, those workers are at risk, depending on the number concentration, if CNTs possess toxicity like asbestos.
CitationLam et al. (2004) and CitationWarheit et al. (2004) reported that intratracheal instillation of CNTs caused severe granulomatous lesions. Recently CitationPoland et al. (2008) have shown that long MWCNTs result in asbestos-like, length-dependent, pathogenic behavior including inflammation and the formation of lesions known as granulomas in mice. CitationTakagi et al. (2008) have revealed that MWCNTs formed fibrous or rod-shaped particles around 10 to 20 micrometers of length with an aspect ratio of more than three, and induced mesothelioma by intraperitoneal injection of MWCNTs. Furthermore, MWCNTs are highly cytotoxic to macrophages which play an important role in removing particulate materials from the alveolar surface (CitationHirano et al. 2008).
Inhalation studies are required to evaluate the pulmonary toxicity of nanomaterials, because nanoparticles are easily agglomerated in suspension and unexpected changes may occur by obstructing the airways. The particle shape of CNTs is fiber-like, which means structurally they are large in one dimension, in length, but have small aerodynamic diameters due to narrowness in width. Fibers, even though they are long, can readily traverse to the small airways where they have a high likelihood of interceptive deposition (CitationHinds 1999).
Several researchers have reported on the generation methods of airborne fiber. Glass fibers have been generated using a high energy fluidization (CitationBaron et al. 2002). The glass bead (nominally 70 μm in diameter)/fiber mixtures were fed into a centrifuge tube, and this was shaken by orbital shaker with the air passing through the tube. Generation rate was controlled by the feeding rate into the centrifuge tube. In CitationMaynard et al. (2004), single-walled CNTs (SWCNTs) aerosol was generated using single- or two-component vortex shaker fluidized-bed to evaluate the physical nature of the aerosol formed from SWCNTs during mechanical agitation. In two-component fluidized-bed, the SWCNTs derived from laser ablation were mixed with a small quantity of bronze beads (approximately 70 μm in diameter), placed in a centrifuge tube, and agitated using a vortex shaker. More recently, to aerosolize MWCNTs (CitationMitchell et al. 2007) and SWCNTs (CitationBaron et al. 2008) for inhalation study, they have constructed a generating device that consisted of a mill for tearing the agglomerates apart, coupled to a dry chemical screw feeder or to an acoustic feeder system. CNTs have a feature of both nanoparticles and conventional fibers (CitationDonaldson et al. 2006). Although the length is an important factor to evaluate the toxicity of CNTs, this factor is not well-documented in previous inhalation studies.
A special attention must be paid to prevent accidental exposure of operators to MWCNTs, because this material is suspected to be harmful. Therefore, simple and easy maintenance is one of the important aspects from engineering control's point of view. We designed a simple system to generate airborne MWCNTs for inhalation studies. Though this is a batch generating system, a continuous exposure over ten hours is possible and the operation and maintenance are not difficult. We also checked the performance of the airborne MWCNT generation system and characterized properties of airborne MWCNTs including fiber length for inhalation toxicity studies.
2. METHODS
2.1. Airborne MWCNT Generation System
MWCNTs were produced by manufacture, made by the floating chemical vapor deposition method developed by CitationEndo (1988). The manufacturer gives a bulk density of the MWCNTs ranging from 0.005 to 0.020 g cm–3 and particle width is 67 nm. The precise length is not determined due to tangled and curly shape, however, the length varies from 3 to approximately 30 μm when the fiber suspension was filtered thorough a Nuclepore filter with a pore size of 3 μm (CitationHirano et al. 2008). MWCNTs form an agglomerated structure and are usually flocculated, so mechanical force is needed to break this agglomeration and to aerosolize them as airborne MWCNTs. A schematic diagram of the generation system is shown in . MWCNTs are mixed with 5 mmϕ stainless steel balls in the cyclone 1 separator with a 50% cut-off particle diameter of 8.8 μm at 10 L min–1 (2.5 μm at 30 L min–1) (Sibata Scientific Technology, Tokyo) made of aluminum (, ). The cyclone 1 separator consists of a storage cup (inside volume 100 cm–3) where a mixture of MWCNTs and balls are placed (), and an air flow line passing through the cup, which performs the role of feeder as well as that of cyclone separator, generating the airborne MWCNTs. Those two parts are connected by chain clamps. The cyclone 1 separator is fixed on top of the sieve shaker (AS200, Retsch, Haan), and MWCNTs and balls were shaken up and down in the cup. Flocculated MWCNTs were packed and formed a mat-like structure spreading at the bottom of the cup after shaking as shown in . MWCNTs play a role in the soft mat, which is expected to reduce background aerosols associated with stainless steel balls and the aluminum cup. The upper layer of mat-like structure of MWCNTs was sheared by mechanical beating balls. The shortened MWCNTs were aerosolized by HEPA-filtered compressed room air (Q1) passing through the cyclone 1 separator as shown in . Finally, the particles of smaller aerodynamic diameter (50% cut-off particle diameter of 8.8 μm) were brought out from the cup. Generated airborne MWCNTs are then introduced to the cyclone 2 separator (URG-2000-30EN, Chapel Hill, NC) with a 50% cut-off particle diameter of 2.5 μm at 10 L min–1 used for supplying the respirable particles. Further HEPA-filtered compressed room air (Q2) is added from downstream of the cyclone 2 separator. In this study, flow rates of Q1 and Q2 were set to 10 and 20 L min–1, respectively, and both flows are supplied to the nose-exposure chamber (SIS-20MB, Sibata Scientific Technology) to expose rodents to particles. This amount of flow rate is enough for the maximum number of rodents (n = 16; ca. 200 cm3 min–1 per 1 rat) and to monitor particle concentration (18 L min–1). The contaminated air is exhausted outside through the HEPA filter by a blower.
FIG. 1 Schematic diagram of the generation system and nose-exposure chamber for airborne multi-walled carbon nanotubes (MWCNTs).
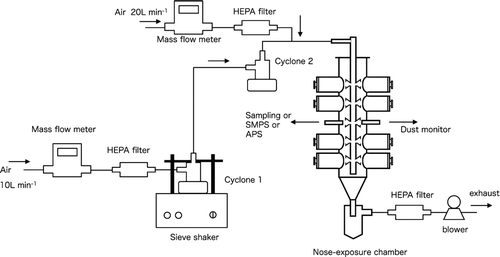
FIG. 2 (a) Configuration of the cyclone 1 separator. MWCNTs were mixed with stainless steel balls in the cyclone with a 50% cut-off particle diameter of 8.8 μm at 10 L min–1 made of aluminum. The cyclone was fixed on the sieve shaker, and HEPA-filtered compressed room air was passed through the cyclone during shaking. (b) The picture of cyclone 1 shows the top part (air-inlet and outlet) and the separable bottom part (storage cup). There are MWCNTs (0.24 g) and stainless steel balls (35 g) in the storage cup (c) before and (d) after shaking on the sieve shaker. Flocculated MWCNTs were packed and formed a mat-like structure on the bottom of the cup after shaking.
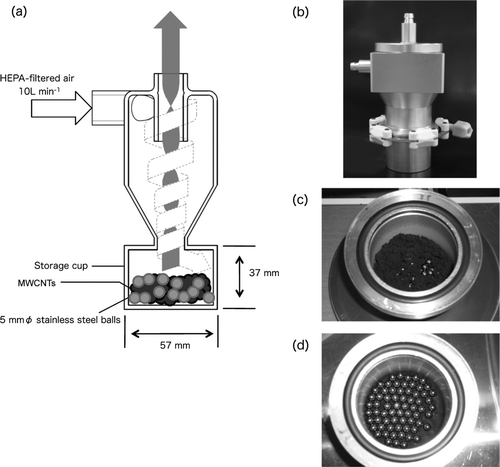
To avoid electrostatic effects during the handling of MWCNTs, we used tools made of conductive materials; in the preparation, MWCNTs were handled by shovels made of conductive plastic when taken from plastic bags containing the MWCNTs. A constant amount was weighed by scale, and then put into the cup by stainless steel spoon. After generation examination, the cyclone separator was vacuumed out using a vacuum cleaner equipped with ultra-low-penetration air (ULPA) particulate filter (CV-G104C, Hitachi, Tokyo). The operators wore respiratory protection, protective wear, arm covers, sandals, and gloves when operators were inside protective controlled area.
2.2. Monitoring and Characterization of Airborne MWCNTs
For monitoring and evaluation of particle properties, the air outlets were set at the nose-exposure chamber (). The relative particle mass concentrations were monitored with a dust monitor (AP-632T, Sibata Scientific Technology) as CPM (count per minute) at flow rate of 18 L min–1, which is based on light scattering method. There is a relationship between the CPM (converted from scattering intensity; sensitivity to scattering intensity can be artificially adjustable) and the particle mass concentration, so it is a good indicator of real time monitoring of particle mass concentration. To convert the value of CPM to mass concentration, use of the dust monitor and particle sampling with a quartz fiber filter (2500QAT-UP, Pall, Pine Bush, NY) were conducted simultaneously. The collected particles were analyzed for mass concentration and elemental carbon (EC) and organic carbon (OC) components. Further, the system background mass concentrations were measured by means of Teflon filter (FP-500, Sumitomo Electric, Osaka).
Intermittent monitoring of the number-weighted size distribution and number concentration were conducted with a scanning mobility particle sizer (SMPS 3034, TSI, Shoreview, MN; size range: electrical mobility diameter (D m ) = 10 – 486.97 nm; 50% cutoff diameter: 800 nm) at flow rate of 1 L min–1 and superior-sized particles were measured with an aerodynamic particle sizer spectrometer (APS 3321, TSI; size range: aerodynamic diameter (D ae ) = 0.3 – 20 μm) at flow rate of 5 L min–1.
The mass-weighted size distribution was measured aerodynamically using a low-pressure impactor manufactured by Dekati (DLPI; referred to hereinafter as “LPI”). As for collection media for measurement of mass weighted size distribution, gold (Au) foil (Tanaka Kikinzoku, Tokyo; purity 99.99%, 25 mmϕ) coated with vacuum grease (MOLYKOTE HP-300, Dow Corning, Midland, MI) was used to prevent particle bounce. Another size-resolved sampling was conducted on collodion membrane-coated copper grids (Nisshin EM, Tokyo; 200 mesh) for transmission electron microscopy (TEM). We set the TEM grids at stages 3 (50% cut off aerodynamic diameter; D ae = 92–154 nm), 5 (D ae = 260–381 nm), and 9 (D ae = 1.59–2.38 μm) by one stage at each sampling. Other stages were set to greased foil to prevent particle bounce.
Particle mass was obtained by incrementing the mass of the collection media after sampling. Weights were measured by an electrical microbalance (UMX 2, Mettler-Toledo, Columbus, OH; readability 0.1 μg) in an air-conditioned chamber (CHAM-1000, Horiba, Kyoto) at constant temperature and relative humidity conditions (25°C, 50%). Each sample was weighed at least twice, and the results were averaged if the difference was within 1 μg; the mean value was adopted as the weight of the sample. The water content of each sample affects the measurement of the particle weights, especially in quartz fiber filters. Therefore, quartz fiber filters were stored in the chamber for 24 h before weighing to ensure a constant water content in the filters as much as possible. Au foils and Teflon filters do not require such a procedure because the mass of water content may be negligible compared with increments of particle mass. A static discharger (TAS-182, TRINC, Hamamatsu) was used to avoid any interference from electrical charges for Teflon filters.
The amounts of OC and EC on the quartz fiber filter were determined by means of a thermal/optical method with a carbon analyzer (DRI model 2001, Reno, NV) and applying the IMPROVE protocol (CitationChow et al. 2001) : the OC1, OC2, OC3, and OC4 fractions underwent combustion at temperatures of 120, 250, 450, and 550°C, respectively, in a pure helium atmosphere, whereas the EC1, EC2, and EC3 fractions underwent combustion at 550, 700, and 800°C, respectively, in an atmosphere of 98% helium and 2% oxygen. EC is shown as sum of EC1, EC2, and EC3.
We used TEM (JEM-2010, JEOL, Tokyo) and energy dispersive X-ray spectroscopy (EDS; JED-2300T, JEOL) to characterize particle morphology and elemental composition, respectively.
3. RESULTS AND DISCUSSION
3.1. Performance of Airborne MWCNT Generation System
Airborne MWCNT generation rate can be controlled by the amplitude of the sieve shaker. The MWCNTs (0.24 g) were mixed with 5 mmϕ stainless steel balls (35 g) and then the mixture entered the aluminum cup. shows amplitude of sieve shaker linearly related to the value of count per minute (CPM). Amplitude of sieve shaker changed from 1 cm to 2 cm, then, the value of CPM ranged from 120 to 300, which corresponded to 0.4 to 1.2 mg m–3 of particle mass concentration by means of quartz fiber filter as shown in . These concentrations were > 8 times higher to the value reported by CitationMaynard et al. (2004) during handling of CNTs in a facility. The ratios of EC to Mass were 0.97 ± 0.1 (average ± SD, n = 4), indicating that the particle mass was mostly derived from MWCNTs. The system background (configurations were the same as generating airborne MWCNTs except for lack of shaking) mass concentration was 0.003 mg m–3 by means of Teflon filter. So, the system background value is negligible relative to during generation of airborne MWCNTs. Also, it was anticipated that the stainless steel balls and cup of aluminum could be broken and aerosolized due to mechanical force during shaking. We conducted the background tests to check for the contributions of other particles, by putting 5 mmϕ stainless steel balls of 35 g without MWCNTs in the aluminum cup. Other configurations were the same as those generating airborne MWCNTs. No increase of mass concentrations was observed relative to the value of system background at amplitude 1–2 cm, and the small increase (0.03 cm–3) of particle number concentration at amplitude of 1 cm (that of system background was 0.01 cm–3) was measured with SMPS (D m = 10–490 nm). So these results indicate that contributions of background particles are small.
FIG. 3 Relationship between (a) amplitude of the sieve shaker and relative particle concentration (CPM) of airborne MWCNTs and (b) mass concentration by means of quartz fiber filter and CPM. Airborne MWCNTs were supplied with air at flow rate of 30 L min–1 and the concentration was monitored at nose-exposure chamber.
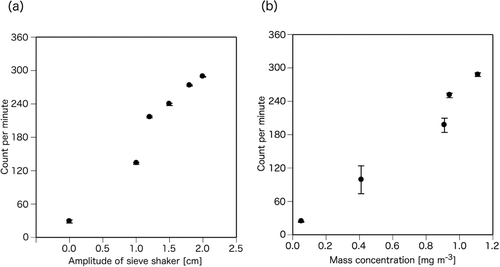
The following describes results of the system performance check and physicochemical characterization of airborne MWCNTs at concentration of 0.4 mg m–3 (amplitude of sieve shaker was about 1 cm). First, stability and reproducibility were checked for inhalation studies. MWCNTs (0.24 g) were mixed with 5 mmϕ stainless steel balls (35 g) and the mixture entered the aluminum cup, where a 2 h generation test was repeated 5 times. and show the time series of CPM and average and SD during 2 h, respectively. The test started from the system background, and it ended in the system background. The duration of the continuous examinations were limited to 2 h to avoid causing stress to the animals in our inhalation study. The value of CPM during 2 h was mostly stable and reproducibility of average value of CPM was good. We estimated the generation efficiency by comparing generating amount of airborne MWCNTs (total flow rate multiplied by concentration in the nose-exposure chamber) and decrease in amount of MWCNTs in the cup after generation. Estimated generation efficiency was 34.5%, and loss occurs at cyclone 2 and deposition in line. Even though the kind of CNT is different, the generation efficiency was higher than that of SWCNTs generating system (10–12%) (CitationBaron et al. 2008). This cup performs the role of feeder as well as that of cyclone separator, so larger particles did not come out from the cup (the 50% cut off diameter is 8.8 μm at flow rate of 10 L min–1). Large agglomerate MWCNTs are shaken with balls until they are sheared into smaller particles of aerodynamic diameter. shows the mass-weighted size distribution measured with LPI (aerodynamic measurements). The size distribution was monomodal with a modal diameter at around 1–2 μm. Due to the cyclone separator, the mass concentration of particle diameter over 2.5 μm suddenly decreased. The total concentration of 0.44 mg m–3 was similar to quartz filter sampling of 0.41 mg m–3 at the same amplitude of sieve shaker. shows number-weighted size distributions measured with SMPS monitoring (electrical mobility measurements) and APS (aerodynamic measurements). In SMPS (D m = 10–490 nm) result, the modal diameter of around 300 nm was measured. Total number concentration was 1328 cm–3 during generation, so system background value of 0.01 cm–3 was also negligible when generating the airborne MWCNTs. In APS (D ae = 0.3–20 μm) result, the modal diameter ranges to the smallest of measureable size. Sum of particle number concentrations of airborne MWCNTs by these instruments were ca. 1700 cm–3, which was about 9 times higher than maximum concentration measured by CitationHan et al. (2008) where they monitored at a MWCNT research facility. As for comparison between SMPS and APS, though there was a large difference in the particle number concentration in the closest respective measurement size range, we can find that the modal diameter was not larger than 1 μm on the basis of particle number. CitationBaron et al. (2008) also showed a disparity in the particle size distributions for SWCNT particles between mobility analysis by SMPS and aerodynamic sizing by APS in the overlap size range. They pointed out that the mobility analysis had uncertainties caused by lack of understanding of charging properties of very-low-density, open-structured, and conductive particles. Also, due to the light absorption properties, APS may underestimate the concentration of SWCNT particles. They concluded that additional work was needed to understand how to compare the SMPS and APS spectra. The data of APS (number) and LPI (mass) are comparable when particle density and the dynamic shape factor are taken into account because both instruments are based on aerodynamic measurements. We compare each data set by converting APS data on assumption that the values of particle density and dynamic shape factor are not changed over the total size range. Both normalized size distributions showed a similar monomodal shape with modal diameter near 1 μm (). Additional work is required to compare size distribution in absolute value from known size-resolved particle density and the dynamic shape factor.
FIG. 4 (a) Time-course changes in relative particle concentration (CPM) of airborne MWCNTs for 2 hours with 5 reproducibility tests. (b) The average and SD of CPM. Airborne MWCNTs were generated by the shaker at amplitude of 1 cm and supplied with air at flow rate of 30 L min–1. Concentrations were monitored at the nose-exposure chamber.
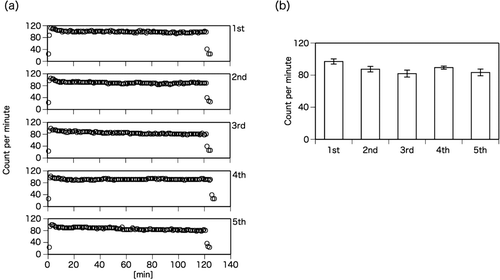
FIG. 5 (a) Mass-weighted size distribution of airborne MWCNTs measured by LPI (mass-based aerodynamic measurement). Particles were collected on a grease-coated gold foil. (b) Size distributions of airborne MWCNTs as measured with SMPS (number-based electrical mobility measurement) and APS (number-based aerodynamic measurement). (c) Comparison between LPI and APS in normalized size distributions. Number-based aerodynamic data obtained by APS were converted to mass-based data assuming that the values of particle density and dynamic shape factor were constant over all sizes. Airborne MWCNTs were generated as shown in the legend to .
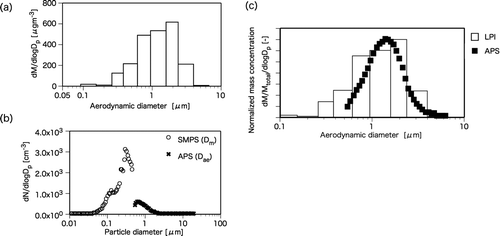
We conducted airborne MWCNT generation tests with alternative balls as listed in at the constant amount of 35 g and mixed with 0.24 mg of MWCNTs. The shape of airborne MWCNTs size distributions (data not shown) and particle sizes () were independent of the kind of balls, but the values of CPM and number concentrations were variable among them ( and ). The tests with 5 mmϕ stainless steel balls were repeated 3 times to check the reproducibility. Low concentrations were found in the silver balls and glass balls. This is because these balls cannot produce mechanical force large enough to shear the MWCNTs due to smaller size or lighter weight in each ball. These results confirm that the concentration during use of 3 mmϕ stainless steel balls was lower than that during use of 5 mmϕ ones. Further, the values of CPM increased as amount of 5 mmϕ stainless steel balls increased (). On the contrary, no airborne MWCNTs were generated when putting MWCNTs alone in the cup (data not shown). These results indicate that mechanical force is needed to generate the airborne MWCNTs. And it is noted that the values of CPM were less dependent on amount of stainless steel balls over 35 g, thus we determine that the appropriate amount of stainless steel balls was 35 g.
TABLE 1 The properties of balls mixing with MWCNTs to generate airborne particles
FIG. 6 The sensitivity in the particle concentration measurement. Airborne MWCNTs were generated by the shaker (amplitude, 1 cm) and supplied with air (flow rate, 30 L min–1) and the concentration was monitoring at nose-exposure chamber. (a–c) Types of balls are shown in . The tests with 5 mmϕ stainless steel balls were repeated 3 times to check the reproducibility; (a) Geometric mean diameter and modal diameters measured with SMPS (D m = 10–486.97 nm); (b) relative particle concentration (CPM); (c) Particle number concentrations measured with SMPS (D m = 10–486.97 nm). (d) Amount of 5 mmϕ stainless steel balls in relative particle concentration (CPM).
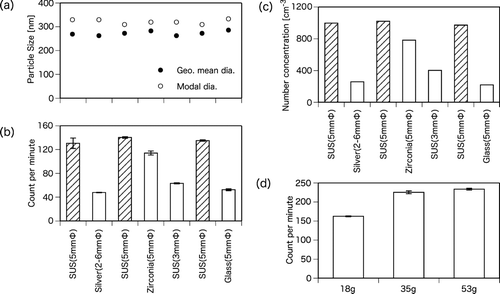
3.2. Morphological Analysis of Airborne MWCNTs by TEM
shows TEM image of the original MWCNTs sample. Fibers were entangled, which indicates that the mechanical force is needed to shear the agglomeration and to aerosolize respirable size particles. The width of fiber was 73.8 ± 20 nm (average ± SD, n = 17), this value was similar to nominal characteristics reported by maker (67 nm). In LPI measurement, samples of each aerodynamic diameter (D ae ) size range were mainly categorized into dissociated fiber-like MWCNT particles (, , and ) and agglomerated MWCNT particles (, , ). The D ae of particles in and ranged from 92 to 154 nm corresponding to the nanoparticle size range, those in and were D ae = 260–381 nm corresponding to mode in number, and those in and were D ae = 1.59–2.38 μm corresponding to mode in mass. We counted the dissociated fiber-like and agglomerated MWCNT particles for the number of 50–150 particles in some grid openings as shown in in each D ae size range, and we measured the contribution of dissociated fiber-like MWCNT particles to observed particles. Because recent study suggests that particle toxicity depends on the degree of agglomeration of particles (CitationFoucaud et al. 2007), it is important to show how MWCNTs fibers are dispersed in the media. The ratios among the D ae size ranges showed a nearly constant value. Percentages of dissociated fiber-like MWCNT particles in D ae = 92–154 nm, 260–381 nm, and 1.59–2.38 μm were 31.5%, 37.8%, and 28.6%, respectively. These values are requisite when we assess the quantitative relationship between number concentration of dissociated fiber-like MWCNT particles and their toxicity.
FIG. 8 TEM image for aerodynamic-classified airborne MWCNTs. Airborne MWCNTs were generated as shown in the legend to and collected at nose-exposure chamber by LPI. (a, c, e) Dissociated fiber-like MWCNT particles and (b, d, f) agglomerated MWCNT particles. (a, b) Particles were collected on the stage 3 (D ae = 92–154 nm, magnification × 30000), (c, d) the stage 5 (D ae = 260–381 nm, magnification × 30000), and (e, f) the stage 9 of LPI (D ae = 1.59–2.38 μm, magnification × 10000).
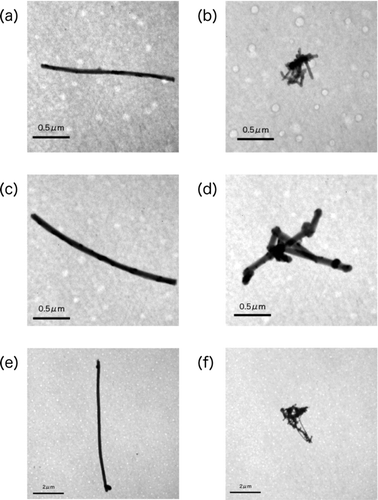
To measure the detailed properties of dissociated fiber-like MWCNT particles, image processing was conducted using TEM image as shown in , , and . The width of fiber and projected surface area were measured, and we calculated the length of fiber and the aspect ratio (length/width). We measured 12–24 samples for each preparation and averaged the width, length, and aspect ratio. shows the average and SD for each value in different D ae size ranges. Though the width of fiber was increased as D ae increased (), the difference between the length of fiber of D ae = 92–154 nm and that of 260–381 nm was not so large (). In three size ranges, the width and length of fiber were 60–120 nm and 3.7–11 μm, respectively, which results in the aspect ratio of 50–100 (). The width of airborne MWCNTs was similar to that of original MWCNTs, which indicates that mechanical force did not change the width of each fiber when a mat-like structure was formed and the fiber was sheared. The measured width and length of fiber are comparable in range to those measured at a working environment where handling of MWCNTs takes place (CitationHan et al. 2008). We concluded that airborne MWCNTs generated by this system can be surrogates of those that are present in the workplace on the basis of particle morphological properties. Also, it is important to characterize the length of fiber from the aspect of toxicity. The probability of pleural sarcoma correlated best with the number of fibers that measured 0.25 μm or less in diameter and more than 8 μm in length, and correlated relatively high with those up to 1.5 μm in diameter and lengths greater than 4 μm in the 72 experiments using the respirable size of minerals particles and wide variety of chemical and structural varieties (CitationStanton et al. 1981). The width and length of the airborne MWCNTs generated by this method corresponds to the size range where they have toxicity, therefore, we can validate whether Stanton's results also apply to airborne MWCNTs.
FIG. 10 Results for (a) width, (b) length, and (c) aspect ratio (length/width) of dissociated fiber-like MWCNT particles at different aerodynamic diameter in LPI samples. Data are shown as averages with SD (N = 12–24).
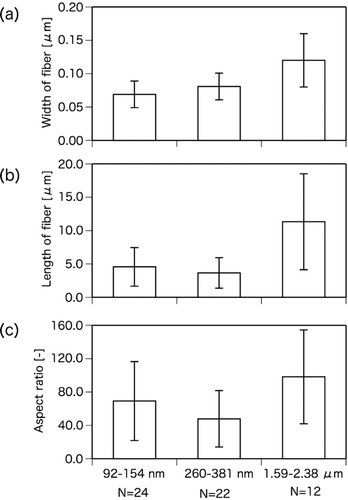
The aerodynamic diameter can be converted to equivalent volume diameter when the particle density and dynamic shape factor are known. The equivalent volume diameter of airborne MWCNTs is expected to be closer to width dimension rather than length dimension judging from morphological results. They are large in particle length but have small aerodynamic diameter (The fiber lengths/D ae is 6–38 times), which indicates that it is difficult to predict the aerodynamic behavior from this dimension. These results show that the inhaled fiber particles may enter deeply in spite of their lengths of fiber, and are easily deposited by the intersections that are specific for airborne particles of fiber-like structure.
The EDS analysis showed that carbon and oxygen were detected in all LPI samples, which were also detected in the original MWCNTs sample. Additionally, in some samples silicon, which was not detected in the original MWCNTs sample, was detected, although the origin of silicon was not clear. One possibility is the contribution of the silicone grease used in the nose-exposure chamber.
4. CONCLUSION
A simple batch particle generation system was developed for generation of airborne MWCNTs. The reproducibility tests over 2 h indicated that particle size and total number concentrations were stable. The averaged width and length of fiber were 80 nm and 3.7 μm, respectively, at aerodynamic size for particles of 260–381 nm. Using these techniques an inhalation study for risk evaluation of the airborne MWCNTs can be conducted.
Acknowledgments
This work was funded in part by the National Institute for Environmental Studies under the program for assessing the health risks associated with the disposition of environmental nanoparticles. We would like to thank Dr. S. Kobayashi and Dr. Y. Kondo (Social and Environmental Systems Division, National Institute for Environmental Studies) for their maintenance of the microbalance, Dr. K. Tamura (Environmental Health Sciences Division, National Institute for Environmental Studies) for advice about LPI sampling, and Dr. S. Hasegawa (Asian Environmental Research Group, National Institute for Environmental Studies) for maintaining the carbon analyzer. We also thank S. Kinoshita (National Institute for Environmental Studies), Y. Ideno (Sibata Scientific Technology), and T. Fujii (Horiba Techno Service) for technical support of this study.
REFERENCES
- Baron , P. A. , Deye , G. J. , Aizenberg , V. and Castranova , V. 2002 . Generation of Size-Selected Fibers for a Nose-Only Inhalation Toxicity Study . The Annals of Occup. Hyg. , 46 ( suppl. 1 ) : 186 – 190 .
- Baron , P. A. , Deye , G. J. , Chen , B. T. , Schwegler-Berry , D. E. , Shvedova , A. A. and Castranova , V. 2008 . Aerosolization of Single-Walled Carbon Nanotubes for an Inhalation Study . Inhal. Toxicol. , 20 : 751 – 760 .
- Chow , J. C. , Watson , J. G. , Crow , D. , Lowenthal , D. H. and Merrifield , T. 2001 . Comparison of IMPROVE and NIOSH Carbon Measurements . Aerosol Sci. Tech. , 34 : 23 – 34 .
- Donaldson , K. , Aitken , R. , Tran , L. , Stone , V. , Duffin , R. , Forrest , G. and Alexander , A. 2006 . Carbon Nanotubes: A Review of Their Properties in Relation to Pulmonary Toxicology and Workplace Safety . Toxicol. Sci. , 92 : 5 – 22 .
- Endo , M. 1988 . Grow Carbon-Fibers in the Vapor-Phase . Chemtech. , 18 : 568 – 576 .
- Foucaud , L. , Wilson , M. R. , Brown , D. M. and Stone , V. 2007 . Measurement of Reactive Species Production by Nanoparticles Prepared in Biologically Relevant Media . Toxicol. Lett. , 174 : 1 – 9 .
- Han , J. H. , Lee , E. J. , Lee , J. H. , So , K. P. , Lee , Y. H. , Bae , G. N. , Lee , S. B. , Ji , J. H. , Cho , M. H. and Yu , I. J. 2008 . Monitoring Multiwalled Carbon Nanotube Exposure in Carbon Nanotube Research Facility . Inhal. Toxicol. , 20 : 741 – 749 .
- Hinds , W. C. 1999 . Aerosol Technology: Properties, Behavior, and Measurement of Airborne Particles, , 2nd Edition , New York : Wiley .
- Hirano , S. , Kanno , S. and Furuyama , A. 2008 . Multi-Walled Carbon Nanotubes Injure the Plasma Membrane of Macrophages . Toxicol. Appl. Pharm. , 232 : 244 – 251 .
- Lam , C. W. , James , J. T. , McCluskey , R. and Hunter , R. L. 2004 . Pulmonary Toxicity of Single-Wall Carbon Nanotubes in Mice 7 and 90 Days after Intratracheal Instillation . Toxicol. Sci. , 77 : 126 – 134 .
- Maynard , A. D. , Baron , P. A. , Foley , M. , Shvedova , A. A. , Kisin , E. R. and Castranova , V. 2004 . Exposure to Carbon Nanotube Material: Aerosol Release during the Handling of Unrefined Single-Walled Carbon Nanotube Material . J. Toxicol. Env. Heal. A , 67 : 87 – 107 .
- Mitchell , L. A. , Gao , J. , Wal , R. V. , Gigliotti , A. , Burchiel , S. W. and McDonald , J. D. 2007 . Pulmonary and Systemic Immune Response to inhaled Multiwalled Carbon Nanotubes . Toxicol. Sci. , 100 : 203 – 214 .
- Poland , C. A. , Duffin , R. , Kinloch , I. , Maynard , A. , Wallace , W. A. H. , Seaton , A. , Stone , V. , Brown , S. , MacNee , W. and Donaldson , K. 2008 . Carbon Nanotubes Introduced into the Abdominal Cavity of Mice Show Asbestos-Like Pathogenicity in a Pilot Study . Nat. Nanotechnol. , 3 : 423 – 428 .
- Stanton , M. F. , Layard , M. , Tegeris , A. , Miller , E. , May , M. , Morgan , E. and Smith , A. 1981 . Relation of Particle Dimension to Carcinogenicity in Amphibole Asbestoses and Other Fibrous Minerals . J. Natl. Cancer. I. , 67 : 965 – 975 .
- Takagi , A. , Hirose , A. , Nishimura , T. , Fukumori , N. , Ogata , A. , Ohashi , N. , Kitajima , S. and Kanno , J. 2008 . Induction of Mesothelioma in p53+/− Mouse by Intraperitoneal Application of Multi-Wall Carbon Nanotube . J. Toxicol. Sci. , 33 : 105 – 116 .
- Warheit , D. B. , Laurence , B. R. , Reed , K. L. , Roach , D. H. , Reynolds , G. A. M. and Webb , T. R. 2004 . Comparative Pulmonary Toxicity Assessment of Single-Wall Carbon Nanotubes in Rats . Toxicol. Sci. , 77 : 117 – 125 .