Abstract
Resuspension is an important source of indoor particles. We measured the resuspension of 1 to 20 μm particles on common indoor materials and explored the importance of turbulence to the resuspension process. Experimental variables included materials (linoleum, carpet, and galvanized sheet metal) and bulk air velocity (5, 10, 15, 20, and 25 m/s). At each of these conditions the turbulence intensity in the boundary layer was varied between a low, medium, and high state and ranged from 9 to 34% at the surface. For comparison of resuspension from the considered surfaces and at different flow conditions, we use the relative resuspension, which quantifies resuspension without requiring knowledge of the number of particles initially seeded on the surface. The relative resuspension compares the fraction of particles resuspended at the experimental conditions to the maximum achieved with a controlled impinging jet. In general, the results show that for the ranges considered, increasing velocity caused the largest increase in resuspension, followed by increasing turbulence intensity and then increasing particle diameter. All three material types showed consistent patterns with carpet having the largest resuspension for a given set of conditions, followed by linoleum and then by galvanized sheet metal. High turbulence and high velocity conditions minimized the differences between materials. An understanding of the relative magnitudes of these effects allows for better analysis and mitigation of indoor resuspension.
INTRODUCTION
The term resuspension refers to a phenomenon in which particles, initially on a surface, join the passing fluid stream. Resuspension is important for several manufacturing processes, healthcare delivery systems, radionuclide transport, and geological systems (i.e., CitationSehmel 1980), but also acts as a source of indoor particles (CitationThatcher and Layton 1995; CitationFerro et al. 2004; CitationNazaroff 2004; CitationGomes et al. 2007). Indoor particles participate in deposition-resuspension cycles that are an important source of human inhalation exposure (CitationNazaroff 2004). Six decades of resuspension research has suggested or demonstrated the important role of velocity (CitationBagnold 1954), particle diameter (CitationBagnold 1954; CitationCorn 1961), acceleration (CitationChiou and Tsai 2001; CitationIbrahim and Dunn 2006), turbulence (CitationBagnold 1954; CitationCleaver and Yates 1973; CitationBraaten et al. 1990), vibration (CitationGomes et al. 2007), relative humidity (CitationCorn and Stein 1965), adhesion between the particle and substrate (CitationCorn 1961; CitationCorn and Stein 1965; CitationSehmel 1980), and particle seeding density (CitationIbrahim et al. 2004). Despite this history, relatively little is known about fundamentals of resuspension processes in the indoor environment and particularly about the role of air turbulence.
In indoor environments, particles are resuspended from human activity and operating heating, ventilation, and air conditioning (HVAC) systems (CitationBatterman and Burge 1995; CitationThatcher and Layton 1995; CitationKrauter and Biermann 2007; CitationQian and Ferro 2008). In particular, human activities, such as walking, dusting, and vacuuming, have been identified and studied as resuspension sources of particles (CitationThatcher and Layton 1995; CitationFerro et al. 2004; CitationCorsi et al. 2008; CitationRosati et al. 2008). There are also numerous models of resuspension and many investigators have used laboratory experiments to explore their applicability (e.g., CitationBraaten et al. 1990; CitationWu et al. 1992; CitationPhares et al. 2000; CitationIbrahim et al. 2003). Such experiments typically involve smooth surfaces, such as polished metal and glass, and monodisperse spherical particles deposited in a single layer. These previous resuspension investigations often use metrics such as resuspension rate, resuspension fraction, or detachment fraction, as metrics of resuspension (CitationSehmel 1980; CitationNicholson 1988; CitationBraaten et al. 1990; CitationWu et al. 1992; CitationPhares et al. 2000; CitationIbrahim and Dunn 2006; CitationQian and Ferro 2008). All of these metrics require counting of the number or mass of seeded particles on the sample. Another approach is to characterize resuspension with the threshold velocity, typically defined as the velocity required to resuspend a single particle (CitationBraaten 1994) or the velocity to resuspend half the particles on the surface (CitationIbrahim et al. 2003).
This investigation focuses on the gap between room-scale resuspension and fundamental studies of resuspension under idealized conditions. In particular, we focus on the role of air turbulence, as this factor has been suggested to be critically important to resuspension (CitationCleaver and Yates 1973; CitationWu et al. 1992; CitationBraaten 1994; CitationIbrahim and Dunn 2006). In this work we measure the resuspension of 1–20 μm particles from two flooring materials and HVAC duct material at different velocities and turbulence conditions. In the experiments, we vary turbulence intensity, the ratio of the root-mean-square fluctuations in flow velocity to its mean velocity, independently of velocity in order to assess the impact of this parameter. We use the relative resuspension fraction, Γ, the ratio of particles removed under the given flow conditions to the total particles available for removal by the passing flow, to compare resuspension from different surfaces and airflow conditions. The overall goal is to explore some of the fluid mechanics that lead to indoor particle resuspension.
METHODOLOGY
To determine the import of turbulence intensity on particle resuspension, experiments evaluated particle resuspension (1) from three substrate types, (2) over five velocities, and (3) at three turbulence intensities. The substrates were linoleum, carpet, and galvanized sheet metal, the velocities studied were 5 m/s, 10 m/s, 15 m/s, 20 m/s, and 25 m/s. The range of velocities was selected based on our previous investigation (CitationLohaus et al. 2008), which showed that a velocity of more than 5 m/s is required to resuspend 50% of 3.2 μm particles from linoleum and wood surfaces. These large velocities can exist in HVAC components and for short periods of time at indoor surfaces, such as the floor during a foot stepping or a door closing. Furthermore, to compare the impact of turbulence to the impact of velocity intensity, particle resuspension at three different turbulence intensities were analyzed for each considered velocity.
The velocity and turbulence were measured at seven points above the substrate at distances from 0.0005 m to 0.015 m from the surface. The turbulence intensities achieved at each of these velocities at the surface (measured in the region of 0.005 to 0.001 m from the surfaces) were approximately 9% (low), 11% (medium), and >30% (high) for linoleum and galvanized sheet metal. Due to the complex surface of the carpet samples, turbulence intensities were slightly different, approximately 16% (low), 24% (medium), and 29% (high). Substrate samples were seeded with particles and then the particle-laden samples were placed in a wind tunnel, in which particle concentration in the air downstream of the samples was measured. For each of the three substrates, fifteen airflow conditions (5 velocities and 3 turbulence condition for each velocity) were investigated. For each airflow condition, twelve duplicate samples were tested. Also, fifteen additional duplicate trials for various substrates and flow conditions were collected to ascertain consistency and repeatability.
Seeding
Samples were seeded with KCl salt particles in three identical seeding chambers constructed for uniform dispersion of particles on substrate surfaces. The salt particles were generated by a TSI Large-Particle Aerosol Generator Model 8108. The salt solution used in the generator was 15% KCl salt (Fisher Scientific catalog #P330–3) by weight. Filtered air, needed for particle formation, was provided at a pressure of 220 kPa. This particle generator setup provided drying air flow rates of 1.1 L/s and aspirating air flow rates of 0.31 L/s. The particle generator was allowed to operate for 30 minutes before seeding to reach a steady-state operation.
The top of each 0.41 m × 0.41 m × 0.45 m seeding chamber had a 500 mm2 circular opening for the large-particle generator outlet as well as small exhaust holes on the sides. The particle generator was connected with a tube to the particle supply opening and a constant stream of particles was supplied to the chamber for a period of 30 minutes. The chamber particle dispersion fans, suspended 76 mm from the chamber floor, provided an upward jet that hit the stream of supply particles and dispersed them in the chamber volume. At the bottom of the chamber, 98 mm × 98 mm substrate samples that had been cleaned with isopropyl alcohol, to minimize surface contamination and electrical charge effects, were arrayed around the edge of each seeding chamber. The seeding time period was adjusted to provide sufficient concentration of particles on the substrate in the range of particle sizes from 1 to 20 μm, but also to prevent deposition of particles on top of each other. Several samples were examined with an optical microscope to ascertain that seeding did not result in particle-to-particle contact. In addition, to ensure uniformity of particle distribution on substrate samples, the fans were left on for an additional five minutes after the particle generator stream was removed. The seeding chambers were left sealed overnight, for a period of at least 16 h before samples were used in the wind tunnel which secured that small particles settled on the substrates.
Wind Tunnel Exposure
The experiments were conducted in an Omega Engineering wind tunnel model number WT-4401-S-110V, shown schematically in . Before entering the wind tunnel air passed through a HEPA filter. The inlet of the tunnel converged into a measuring test chamber with a cross-sectional area of 0.1 m by 0.1 m and length of 0.25 m. To enable positioning of seeded samples without obstructing flow in the tunnel, a ramp was positioned in the converging section of the wind tunnel. The samples were placed on a shim downstream of a ramp in a way that did not change the geometry of the measuring chamber upstream and downstream of the sample. A TSI Aerodynamic Particle Sizer Model 3321 measured particle concentrations in the air at the reference point (described below) in the wind tunnel. The Aerodynamic Particle Sizer has a range of 0.542 to 19.81 μm and divides particles into 52 bins, which were further condensed into six bins to ensure that each bin had enough particles to allow for analysis.
Following a 40 s period of background concentration measurement at 0.7 m/s, the velocity in the wind tunnel was increased to the studied velocity, either 5, 10, 15, 20, or 25 m/s with a uniform acceleration rate of 5 m/s2. This velocity was sustained for 40 additional seconds, before being set to the isokinetic sampling rate for the particle sizer, 6.6 m/s, for the final 40 s of the experiment. As the velocity was set to 6.6 m/s an impinging jet nozzle forced compressed air onto the sample (). The impinging jet nozzle with the diameter of 0.002 m was positioned 0.06 m above the sample at the vertical centerline of the tunnel and at an angle of 49° from the ceiling of the chamber. It provided a jet at the substrate that pushed the air on the sample in the direction of the airflow in the tunnel. The air velocity 0.002 m above the middle of the sample was of 40 m/s with an average turbulence intensity of 15%. The duration of impinging jet in each experiment was 10 s long. Pilot experiments that used several consecutive impinging jets showed that all measurable particle resuspension occurred during the first 10 s of the first impinging jet. The resuspension resulting from this large velocity is used as a maximum for each scenario. To secure that no particles were injected with the impinging jet, air supplied by impinging jet was filtered. A schematic timeline of events for each trial is shown in and the methodology for assessing resuspension is provided in the Data Analysis section.
Calibration experiments and simulations identified the reference sampling point in the wind tunnel. It was selected as the point which has the highest concentration of particles and minimal disturbance of the air flow field in the tunnel. A set of computational fluid dynamics simulations determined the effect of various sampling points on airflow field, and calibration experiments with particle resuspension and various position of sampling tube provided the position of reference point in the wind tunnel. The highest concentration of resuspended particles was along the vertical centerline of the tunnel, 0.01 m behind the sample and just above the sample surface. The inlet diameter of sampling tube was 0.004 m. The concentration at this point was the highest for both resuspension caused by the air velocities in the wind tunnel and resuspension caused by the impinging jet.
Variation of Turbulence
The wind tunnel was configured in three different ways to generate three different levels of turbulence intensity. The first configuration, the low turbulence intensity case, involved a honeycomb flow straightener 0.50 m upstream of the leading edge of the sample, before the wind tunnel constricts into the test chamber. With the flow straightener, turbulence intensities at all velocities studied were less than 1% in the bulk flow and on the order of 10% close to the surface. The second configuration, the medium turbulence intensity case, did not use the flow straightener and produced turbulence intensities on the order of 5% at all velocities in the bulk flow. In this case, the turbulence intensity approximately 1 mm from the surface ranged from 10% to 20%, depending on the bulk velocity and substrate. In the final configuration a 13 mm tall removable obstacle, a thin solid bar about 2 mm thick, was located 0.23 m upstream of the leading edge of the sample. This created a turblulent wake behind the obstacle, which could add to the additional resuspension mechanism over the substrate (CitationHuang et al. 2005). The fence generated non-homogenous velocity and turbulence profiles in the study chamber, but also generated a minimum of 27% turbulence intensity approximately 0.001 m from the surface for velocities over 10 m/s.
Velocity and turbulence were measured with a one-dimensional constant-temperature hot-wire anemometer, DanTec Dynamics MiniCTA probe 55P16. The anemometer sampling frequency was 1024 points per second, and the measured velocity fluctuations were used to calculate the turbulence intensity. The accuracy of the sampling system is limited by the accuracy of the Pitot tube and pressure gage used to calibrate the sensor, which was 3%.
Experimental Matrix
A nine-cell matrix consisting of three substrates (linoleum, carpet, and galvanized sheet metal) and three turbulence intensities (low—with flow straightener, medium—with flow straightener removed, and high—with obstacle added) was used. Within each cell, five velocities (5, 10, 15, 20, and 25 m/s) were completed on each of set of 12 samples from one seeding chamber. At least one duplicate set of data was collected for at least one velocity in each flow characteristic and substrate combination in order to assess repeatability and consistency.
Before the experiments defined in the experimental matrix, a set of seeding calibration experiments were conducted to test the uniformity of particles on the substrate. In these experiments 12 linoleum samples from seeding chamber were exposed to the same resuspension velocity of 20 m/s and low turbulence intensity. The difference defined as the standard deviation of 12 samples divided by average measured concentration was in the range of 5 to 14%, with the higher values associated with smaller particles. These results demonstrate that the particle seeding procedure provided reasonably uniform particle concentration on the substrates.
Data Analysis
Resuspension parameters, such as the resuspension rate and resuspension fraction, often depend on knowledge of the number of particles on the surface before and after resuspension events. To obviate the need for time-consuming surface particle counting and to facilitate the use of a polydisperse aerosol, the relative resuspension, Γ, was developed as shown in Equation (Equation1). Use of relative resuspension enabled time-efficient experiments that provided sufficient data needed for comparison of resuspension with different flow properties from different substrates. In Equation (Equation1), the numerator represents the number of particles of a particular size that resuspend at the velocity being measured with a correction for particles removed during the background period. The denominator represents the sum of the particles resuspended during the experiment as well as those resuspended when the impinging jet was utilized. Defined this way, the denominator represents a maximum possible resuspension. This denominator bounds Γ between 0 and 1, where 0 indicates no resuspension occurred at the study velocity and 1 indicates that all possible resuspension occurred. In the seeding process we minimized particle contact on the surface by making sure that the seeding density was low. Therefore, particle-to-particle adhesion impacts are relatively small, and the relative resuspension defined by Equation (Equation1) depends primarily on forces that air velocity imposes on particles and not on particle seeding density on the substrate. The time periods being averaged in Equation (Equation1) are summarized in .
After all data were collected and compiled, the 12 repeated tests were averaged for each combination of airflow parameters and substrate. Including the repetition tests, a total of 720 samples were measured and including six particle bins for each sample a total of 4320 data points were collected. Any sample with a Γ more than three standard deviations away from the mean for identical experimental conditions was discarded. This eliminates outliers that may reflect inconsistency in seeding or other issues. Using this criterion, 1.3% of all data were excluded. Negative Γ values resulting from high background concentrations were also excluded, removing 3.8% of the data, mostly in the 1 to 3 μm particle size bin where resuspension was less likely to occur. Lastly, 0.3% of the data were excluded due to operator error in velocity control or turbulence bar placement.
RESULTS
Results from the experimental trials showed the impacts of velocity, turbulence intensity, particle size, and substrate on particle resuspension. Each of these parameters is discussed separately and is used to illuminate some of the differences in resuspension measured for each scenario.
Velocity and Turbulence Intensity
compiles velocity and turbulence intensity data for each of the three substrates, linoleum in the first row, carpet in the second, and galvanized sheet metal in the third, at each of the turbulence intensities, low, medium, and high, arranged in ascending order in columns. At the junction of each substrate and turbulence condition, a smooth line plots the velocity versus the distance above the surface for each of the five bulk velocities studied.
FIG. 3 Velocity and turbulence profiles for the three substrates (rows) and turbulence cases (columns). The average turbulence intensities shown are the averages of the near-surface turbulence intensities at 15, 20, and 25 m/s bulk velocity. The size of each symbol corresponds to its turbulence intensity.
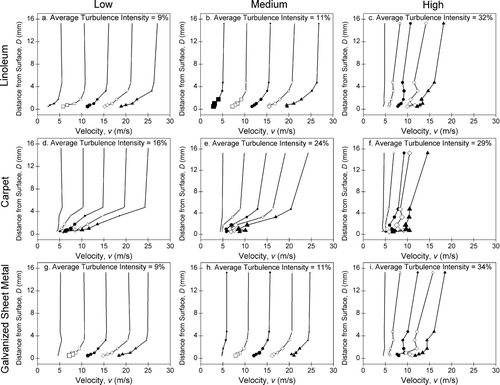
As expected with turbulent flow, the profiles in show plug flow, with the exception of the high turbulence cases. In the high turbulence cases, the positioning of an obstacle upstream of the measurement probe generates non-homogeneous flow, but the general trend of decreasing velocity closer to the surface is still present. All cases show a sharp velocity gradient in the boundary layer. Because the carpet fibers do not have a distinct surface, the carpet boundary layer is less well defined than those of linoleum and galvanized sheet metal. Besides variations attributable to the high turbulence and carpet, uncertainty caused by slight variations in probe positioning affects the smoothness of the velocity profiles in the vicinity of the surface.
FIG. 4 Relative resuspension, Γ, for linoleum samples under low, medium, and high turbulence conditions.
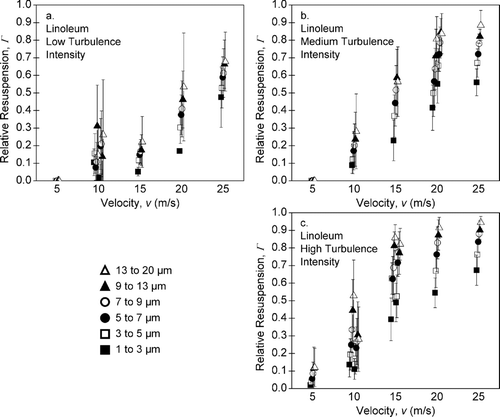
The symbol sizes in represent the magnitude of the turbulence along the velocity profile. Symbols are scaled by the average turbulence intensity presented in the upper left corner. At most velocities the turbulence intensity is largest at higher bulk velocities and closer to the surface. For linoleum, bulk turbulence intensity remains low relative to surface turbulence intensity at the low and medium turbulence configurations, a trend that holds, albeit less strongly, for carpet and galvanized sheet metal, as well. In the high turbulence configuration, bulk turbulence remains elevated. For all substrates and turbulence scenarios turbulence intensity increases with velocity.
Each plot in includes an average turbulence intensity value. The average turbulence intensity value is the average of the near-surface turbulence intensities measured at 15, 20, and 25 m/s bulk flow. For low turbulence cases over macroscopically smooth surfaces such as linoleum and galvanized sheet metal, the average turbulence intensity was 9%. Over the rougher carpet, the average turbulence intensity was 16%. In the medium turbulence intensity case, the flows over linoleum and galvanized sheet metal again have similar turbulence intensities. In the high turbulence cases, galvanized sheet metal and linoleum present equally large average surface turbulence intensities, followed by carpet.
Resuspension
displays the relative resuspension for linoleum under all three turbulence conditions. Different symbols represent each of the six particle size bins. Each point on the plot is the mean of one set (12 samples at the same conditions) that met the quality control criteria described above and the error bars represent the standard deviation. Figures a–c present the data collected under the three turbulence configurations studied. For visual clarity, a third duplicate sample has been removed from a at 10 m/s, although these data were included in all analysis.
FIG. 5 Relative resuspension, Γ, for carpet samples under low, medium, and high turbulence conditions.
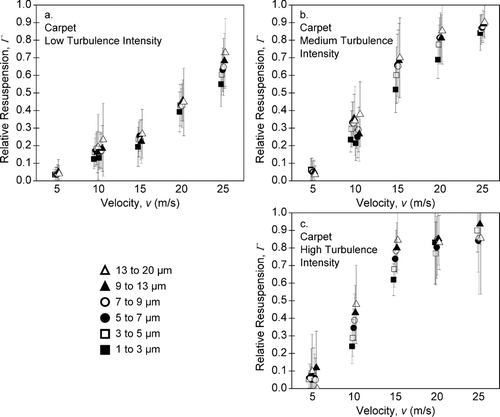
In all turbulence cases the relative resuspension increases with increasing bulk velocity. This confirms previous experimental work performed for similarly sized particles (CitationBagnold 1954). Generally, larger particle diameters have a larger Γ, when all other factors are the same. Small particles have a lower Γ, which is consistent with the work of CitationThatcher and Layton (1995) and CitationBraaten (1994), among others, who show small resuspension rates for smaller particles. Γ for the smaller particle size bins, 1 to 3 μm and 3 to 5 μm, reflect some interference from background particles.
In addition to the trend of increased resuspension with increased velocity, increasing the turbulence intensity tends to increase the relative resuspension. The difference between the peak Γ for air velocity of 25 m/s in Figures a (mean turbulence intensity = 9%) and 4c (mean turbulence intensity = 22%) is approximately 0.25 for all particle sizes, with no overlap of the error bars for some particle size bins. A similar trend is observed for smaller velocities (20, 15, 10, 5 m/s). Although the difference in turbulence intensity is modest between the low and medium cases, the change in Γ is still noticeable. The jump in turbulence intensity between the medium and high cases is larger than between low and medium but the effect on Γ is less pronounced. This may be due to the fact that Γ is close to unity for the higher velocities.
, which shows the same information for carpet, differs from mostly at 5 m/s, for which Γ is generally larger for carpet than for linoleum. It seems that the nature of the carpet causes lower adhesion. Using visual inspection with an optical microscope we found that most seeded particles were not imbedded deep into the carpet fibers, but instead were located closer to the canopy surface. Due to the uneven length of carpet fibers, these particles attached on the carpet surface fibers could be exposed to the higher velocity than particles on the smoother materials, which may explain the larger Γ for carpet. In general, the relative resuspension shown in is slightly higher than those in , with the increases heightened for lower velocities. Increasing turbulence intensity over the carpet produces the same effects as over linoleum, resuspending more particles with high turbulence than with low. Particle diameter matters less for carpet than for linoleum, as the spread of Γ at a given velocity and turbulence case is smaller. The error bars for the carpet data are also smaller than for linoleum, with the exception of the high turbulence case at 25 m/s.
FIG. 6 Relative resuspension, Γ, for galvanized sheet metal samples under low, medium, and high turbulence conditions.
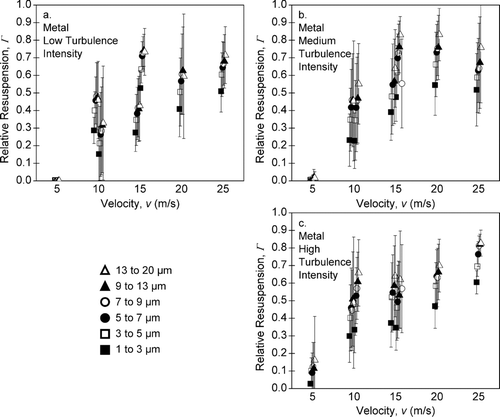
The resuspension fractions for the experiments on galvanized sheet metal, presented in , are generally lower than for linoleum or carpet. With increasing velocity, Γ does not increase as steeply as for the other substrates, suggesting other factors besides velocity may play a larger role for this material. Electrostatic effects may account for the differences in materials, causing increased or decreased adhesion to the surface (CitationQian and Ferro 2008). Even though the large particle generator has an ion generating neutralizer, many of the larger particles will still have a net electrical charge. Also, despite the cleaning of the surfaces with isopropyl alcohol prior to seeding, ions in the air during drying or during exposure may have charged portions of the metal surface causing an electrostatic enhancement of adhesion. Turbulence intensity also has a smaller impact for galvanized sheet metal than for the other materials, which may be due to its small surface roughness. Based on the literature, the surface roughness for the galvanized sheet metal is 0.15 mm (CitationASHRAE Handbook 2005) and for the linoleum coating it is 0.08 mm (CitationEl Hamdani et al. 2008). It should be noted that linoleum is a more complex surface than sheet metal with both microscopic (coating: 0.08 mm) and macroscopic (tile texture: 0.5 mm) surface roughness elements. The carpet has a more complex surface roughness than either of the two other substrates because long fibers extend far above the carpet base. We estimate that the roughness of just the carpet canopy surface was approximately 1 mm.
Threshold Velocities
Another way to characterize resuspension is the threshold velocity, v 50, defined here as the velocity for which Γ is 0.5. Threshold velocities for all tested particle sizes and turbulence intensities were calculated by linear interpolation between the bounding data points and are tabulated in . Uncertainties in the threshold velocities follow from both the uncertainties in Γ and the variations in the velocity of the bulk flow from the nominal set-point velocities of 5, 10, 15, 20, and 25 m/s.
TABLE 1 Threshold velocities
As the turbulence increases from low to medium the threshold velocities drop in all cases. In other words, at the same bulk velocity, increasing the turbulence intensity will increase the amount of particle resuspension. As turbulence intensity increases from medium to high the same general trend holds, but, as with the Γ results, the changes are smaller than when going from low to medium turbulence intensity. Based on , increasing particle diameter lowers slightly the threshold velocity for resuspension, consistent with the results for Γ.
At low turbulence intensity, galvanized sheet metal has lower threshold velocities than linoleum and carpet. However, the relative resuspension for higher velocities (20 and 25 m/s) is smaller than for the carpet and linoleum floor. This indicates that for the galvanized sheet metal significant number of particles resuspend at low velocities (10 and 15 m/s) while the remaining particles stick to the surface even at very high air velocities (20 and 25 m/s). Possible reasons for this include the impact of surface roughness on turbulence very close to the surface or the effect of electrostatic forces.
Across all three turbulence conditions metal threshold velocities vary less than those for linoleum and carpet, suggesting that perhaps turbulence intensity is not a major factor determining resuspension over the smoother metal surfaces. Threshold velocities over the flooring materials vary more with turbulence intensity than they do over metal, and are ultimately lower over carpet than over linoleum.
DISCUSSION
The resuspension metric developed in this research, Γ, varies with velocity, turbulence intensity, and particle size in a consistent manner with earlier research (e.g., CitationBraaten 1994; CitationWu et al. 1992). However, one limitation of Γ is that it is a relative parameter and therefore does not measure the absolute quantity of resuspension. Neither the total amount of particles resuspended nor the number available for resuspension is known. Thus Γ should be interpreted as a parameter that characterizes the changes in resuspension that result from changing the independent variables. Even though Γ is not an absolute quantity of resuspension there are many benefits to comparing Γ for different flow conditions and surfaces provided that the assumption of similar particle behavior under the impinging jet and in the wind tunnel can be made. Factors that may affect absolute resuspension, such as particle and surface roughness, as discussed below, may be minimized if they affect the numerator and denominator in Equation (Equation1) equally. Γ captures the effects of changing one variable at a time.
One important comparison is the impact of turbulence intensity versus that of velocity. Over the parameters considered, varying turbulence intensity caused Γ to increase by approximately 0.5, and velocity by as much as 0.94. These findings have important implications for the control or promotion of resuspension. Turbulence intensity, which is not a strict function of velocity, can affect particle resuspension enough that an effective treatment to quell or initiate resuspension should consider varying turbulence intensity. This may be easier to control than velocity, for example with flow guides and turning vanes in HVAC systems or with ventilation system diffuser types in indoor environments. High turbulence intensities such as the upper range studied here may occur following various disturbances in HVAC components, such as uneven surfaces at duct joints. Surface elements such as joints or duct bends and elbows generate eddies and a correspondingly high turbulence intensity immediately above the surface. In addition, turbulence in the form of large eddies is common in indoor environment; for example, walking generates bursts of air beneath the feet which cause highly oscillatory velocities in the region close to the surface (CitationGomes et al. 2007).
As turbulent eddies carried by bulk flow dissipate into smaller eddies, the smaller eddies penetrate deep into the boundary layer, transporting the kinetic energy of turbulence to the particles at the surface. CitationCleaver and Yates (1973) identified this transport of turbulent kinetic energy by velocity fluctuations in the air layer as a major contributor to resuspension. The sensitivity of particle resuspension to turbulence intensity found in our study corroborates that the effects of turbulence are present in the velocity boundary layer close to the surface. This finding is in agreement with the energy models of resuspension developed by CitationReeks et al. (1988) in which fluctuating drag forces can contribute towards the total energy that a particle needs to escape the forces adhering it to a surface.
Variations in substrate produced modest differences in Γ, with carpet generally having the highest relative resuspension. The definition of Γ as well as the details of the seeding procedure may have contributed to this finding. In order for resuspension to occur, the adhesion force binding the substrate and particles must be overcome (CitationCorn 1961). CitationHu et al. (2008), in their measurements of adhesion forces between 1 μm alumina particles and bacterial spores and vinyl and rubber indoor flooring materials, found the actual area of contact between particles and surfaces is less than the theoretically ideal contact area for indoor surfaces. Differences in substrates, particularly microscale surface roughness, are therefore likely to affect resuspension. Macroscale surface roughness is likely to affect the air turbulence close to the surface and may also affect resuspension. An important aspect of the experiments with carpet is that the seeding and resuspension were focused on the surface of the carpet canopy. In real carpet systems, there is evidence that some particles get pushed deeper into the canopy and become harder to remove (CitationRoberts et al. 1999). Additionally, CitationAhmadi and Guo (2007) found that particles that are not smooth spheres have a lower threshold velocity for resuspension than smooth spheres. When observed under a microscope, the KCl crystals used in our study have a crystalline structure. The microscale roughness of KCl crystals may diminish absolute resuspension; however, the relative parameter Γ may not reflect any effects of particle shape here if particles behave the same way under the velocity loading and the impinging jet conditions.
Relative humidity affects the water film on any surface, which consequently affects particle adhesion to the surface and may make particles more difficult to remove. Researchers such as CitationIbrahim et al. (2004) noted higher threshold velocities for resuspension of particles from smooth surfaces for relative humidities above 30%. However, CitationKildeso et al. (1999) noted maximum resuspension of dust from carpets at 70–75% relative humidity. This difference could be due to different material and particle properties. CitationIbrahim et al. (2004) found that particle residence time on the surface before resuspension experiments increased the threshold velocity for resuspension. They attributed this to increased particle-surface contact area due to a water layer that develops over time. Furthermore, KCl particles may also adsorb water to their surfaces (CitationHinds 1999), but do remain solid when the relative humidity remains below 84% (CitationKelly et al. 2008). The experiments presented above all took place within a small daily average relative humidity range of 61 to 69%. Under these experimental conditions the additional adhesion force caused by relative humidity has a consistent effect on total adhesion force. Additionally, the time between seeding and resuspension in the wind tunnel was generally consistent and in the range of 16 to 24 h. For two duplicate trials with galvanized sheet metal (trials at 15 m/s with low and medium turbulence) experiments were performed with a five-day residence time. In the longer residence time duplicate trials, Γ decreased by about 0.1 to 0.3 which is in general agreement with the findings of CitationIbrahim et al. (2004).
Another factor that has been associated with resuspension is vibration. CitationReeks et al. (1988) found that the natural frequency of a particle on a surface would be the relevant forcing frequency to incite increased resuspension. In order to characterize our experiments, we measured vibration of the sample surface in the wind tunnel with a PCB Piezotronics Model 352C65 accelerometer. Results showed that at 5, 10, and 15 m/s the vibrations occurred at frequencies of about 600 Hz but were of low magnitude. At higher velocities the fan vibration was the major source of vibrations and frequencies of approximately 60 Hz dominated. The measured frequencies were in the range of values below the natural frequency of particles identified by CitationReeks et al. (1988) and CitationZiskind et al. (1995). They found the natural frequency of a 10 μm particle on a flat surface to be 107 Hz, suggesting that the lower frequency vibrations did not contribute to resuspension. This is in agreement with the study of footfall-induced resuspension by CitationGomes et al. (2007), which found that vibrations at the low frequencies of 5.7 and 12.3 Hz were not by themselves significant drivers of resuspension.
In the indoor environment, vibrations from footfalls may be precluded as drivers of resuspension, but gusts and eddies as well as stronger mechanical disturbances that may arise from footfalls should be considered. CitationGomes et al. (2007) used ice clouds to visualize velocities around footfalls, finding velocities up to 2.0 m/s, while CitationZhang et al. (2008) calculated velocities of up to 18 m/s under a footfall. The lowest threshold velocities in are on the order of 10 m/s, providing evidence that walking can cause resuspension. CitationThatcher and Layton (1995) found that indoor activity can nearly double the mass concentration of coarse particles in a room, while CitationCorsi et al. (2008) measured increases in room PM10 concentrations of 7 μg/m3 for pushing a non-operating vacuum cleaner in a room. Such increase in particle concentration can lead to significant exposures, particularly for any contaminants that are associated with larger particles. In addition to footfalls, the velocities associated with HVAC systems, fans, and some buoyancy driven indoor flows can also likely contribute to resuspension.
Thus far the focus has been on reducing resuspension to reduce exposure. However, there are some situations where it may be desirable to induce resuspension in order to clean surfaces or examine the composition of settled dust. The results described here provide for an understanding of the mechanisms that contribute to resuspension. In cases in which velocity is fixed or difficult to regulate, changing flow conditions to increase or reduce turbulence intensity can affect resuspension.
CONCLUSION
We conducted a wind tunnel study to investigate the relative resuspension of 1–20 μm particles and independently varied velocity, turbulence intensity, and surface material (substrate). Increasing velocity and turbulence intensity increased resuspension for all substrates. Larger particle sizes tend to be more easily resuspended than smaller particles under the same flow conditions. Changes in the turbulence intensity increased Γ by as much as 0.5 for the same substrate and bulk velocity. This change is not as great as the change caused by simply raising bulk velocity, as much as 0.94, but allows for significant manipulation of resuspension rate in the cases where larger resuspension is desired by increasing the turbulence properties of the flow. The range of velocities studied here, 5 to 25 m/s, and the range of turbulence intensities at the surface, 9% to 34%, can exist in HVAC components and for short periods of time at indoor surfaces such as the floor during a footfall. In addition to providing valuable information about the role of turbulence intensity in the particle resuspension process, the results presented in this study can help fill gaps in current resuspension models.
An ASHRAE New Investigator Award provided partial funding for this research. We would like to thank Dr. Jinying Zhu and Seong-Hoon Kee for their graciousness in measuring wind tunnel vibrations.
Notes
1Low and medium turbulence intensity trials for galvanized sheet metal at 15 m/s each include one set of samples at which there was a longer time between seeding and exposure than for the rest of the samples studied.
REFERENCES
- ASHRAE . 2005 . “ ASHRAE Fundamentals ” . Atlanta, GA : American Society of Heating, Refrigerating and Air-Conditioning Engineers .
- Ahmadi , G. and Guo , S. 2007 . Bumpy Particle Adhesion and Removal in Turbulent Flows Including Electrostatic and Capillary Forces . J. Adhesion , 83 : 289 – 311 .
- Bagnold , R. A. 1954 . The Physics of Blown Sand and Desert Dunes , London : Methuen & Co. Ltd. . pp. 33, 88
- Batterman , S. A. and Burge , H. 1995 . HVAC Systems as Emission Sources Affecting Indoor Air Quality: A Critical Review . HVAC&R Research , 1 : 61 – 80 .
- Braaten , D. A. 1994 . Wind Tunnel Experiments of Large Particle Reentrainment-Deposition and Development of Large Particle Scaling Parameters . Aerosol Sci. Technol , 21 : 157 – 169 .
- Braaten , D. A. , Paw U , K. T. and Shaw , R. H. 1990 . Particle Resuspension in a Turbulent Boundary Layer—Observed and Modeled, J . Aerosol Sci , 21 : 613 – 628 .
- Chiou , S. F. and Tsai , C. J. 2001 . Measurement of Emission Factor of Road Dust in a Wind Tunnel . Powder Technol , 118 : 10 – 15 .
- Cleaver , J. W. and Yates , B. 1973 . Mechanism of Detachment of Colloidal Particles from a Flat Substrate in a Turbulent Flow . J. Colloid Interface Sci , 44 : 464 – 474 .
- Corn , M. 1961 . The Adhesion of Solid Particles to Solid Surfaces, II . J. Air Poll. Control Assoc , 11 : 566 – 575 .
- Corn , M. and Stein , F. 1965 . Re-Entrainment of Particles From a Plane Surface . Amer. Ind. Hyg. Assoc. J. , 26 : 325 – 336 .
- Corsi , R. L. , Siegel , J. A. and Chiang , C. 2008 . Particle Resuspension During the Use of Vacuum Cleaners on Residential Carpet . J. Occ. Environ. Hygiene , 5 : 232 – 238 .
- El Hamdani , S. E. , Limam , K. , Abadie , M. O. and Bendou , B. 2008 . Deposition of Fine Particles on Building Internal Surfaces . Atmos. Environ. , 42 : 8893 – 8901 .
- Ferro , A. R. , Kopperud , R. J. and Hildemann , L. M. 2004 . Source Strengths for Indoor Human Activities that Resuspend Particulate Matter . Environ. Sci. Technol , 38 : 1759 – 1764 .
- Gomes , C. , Freihaut , J. and Bahnfleth , W. 2007 . Resuspension of Allergen-Containing Particles Under Mechanical and Aerodynamic Disturbances from Human Walking . Atmos. Environ , 41 : 5257 – 5270 .
- Hinds , W. C. 1999 . Aerosol Technology: Properties, Behavior, and Measurement of Airborne Particles 289 – 292 . New York : John Wiley & Sons Inc., .
- Hu , B. , Freihaut , J. D. , Bahnfleth , W. P. and Thran , B. 2008 . Measurements and Factorial Analysis of Micron-Sized Particle Adhesion Force to Indoor Flooring Materials by Electrostatic Detachment Method . Aerosol Sci. Technol. , 42 : 513 – 520 .
- Huang , C. H. , Lee , C. I. and Tsai , C. J. 2005 . Reduction of Particle Reentrainment using Porous Fence in Front of Dust Samples . ASCE J. Environ. Engineer. , 131 : 1644 – 1648 .
- Ibrahim , A. H. and Dunn , P. F. 2006 . Effects of Temporal Flow Acceleration on the Detachment of Microparticles from Surfaces, J . Aerosol Sci , 37 : 1258 – 1266 .
- Ibrahim , A. H. , Dunn , P. F. and Brach , R. M. 2004 . Experiments and Validation of a Model for Microparticle Detachment from a Surface by Turbulent Air Flow . J. Aerosol Sci , 35 : 805 – 821 .
- Ibrahim , A. H. , Dunn , P. F. and Brach , R. M. 2003 . Microparticle Detachment from Surfaces Exposed to Turbulent Air Flow: Controlled Experiments and Modeling . J. Aerosol Sci , 34 : 765 – 782 .
- Kelly , J. T. , Wexler , A. S. , Chan , C. K. and Chan , M. N. 2008 . Aerosol Thermodynamics of Potassium Salts, Double Salts, and Water Content Near the Eutectic . Atmos. Environ , 42 : 3717 – 3728 .
- Kildeso , J. , Vinzents , P. , Schneider , T. and Kloch , N. P. 1999 . A Simple Method for Measuring the Potential Resuspension of Dust from Carpets in Indoor Environments . Textile Research J , 69 : 169 – 175 .
- Krauter , P. and Biermann , A. 2007 . Reaerosolization of Fluidized Spores in Ventilation Systems . App. Environ. Microbiology , 73 : 2165 – 2172 .
- Lohaus , J. H. , Novoselac , A. and Siegel , J. A. 2008 . Particle Resuspension from Indoor Flooring Materials . paper ID 342, 1
- Nazaroff , W. W. 2004 . Indoor Particle Dynamics . Indoor Air , 14 : 175 – 183 .
- Nicholson , K. W. 1988 . A Review of Particle Resuspension . Atmos. Environ , 22 : 2639 – 2651 .
- Phares , D. J. , Smedley , G. T. and Flagan , R. C. 2000 . Effect of Particle Size and Material Properties on Aerodynamic Resuspension from Surfaces, J . Aerosol Sci , 31 : 1335 – 1353 .
- Qian , J. and Ferro , A. R. 2008 . Resuspension of Dust Particles in a Chamber and Associated Environmental Factors . Aerosol Sci. Technol. , 42 : 566 – 578 .
- Reeks , M. W. , Reed , J. and Hall , D. 1988 . On the Resuspension of Small Particles by a Turbulent Flow . J. Physics D—Applied Physics , 21 : 574 – 589 .
- Roberts , J. W. , Clifford , W. S. , Glass , G. and Hummer , P. G. 1999 . Reducing Dust, Lead, Dust Mites, Bacteria, and Fungi in Carpets by Vacuuming . Arch. Environ. Contam. Tox , 36 : 477 – 484 .
- Rosati , J. A. , Thornburg , J. and Rodes , C. 2008 . Resuspension of Particulate Matter from Carpet Due to Human Activity . Aerosol Sci. Technol. , 42 : 472 – 482 .
- Sehmel , G. A. 1980 . Particle Resuspension: A Review . Environ. Intl , 4 : 107 – 127 .
- Thatcher , T. L. and Layton , D. W. 1995 . Deposition, Resuspension, and Penetration of Particles within a Residence . Atmos. Environ , 29 : 1487 – 1497 .
- Wu , Y.-L. , Davidson , C. I. and Russell , A. G. 1992 . Controlled Wind Tunnel Experiments for Particle Bounceoff and Resuspension . Aerosol Sci. Technol. , 17 : 245 – 262 .
- Zhang , X. , Ahmadi , G. , Qian , J. and Ferro , A. 2008 . Particle Detachment, Resuspension and Transport Due to Human Walking in Indoor Environments . J. Adhesion Sci. Technol , 22 : 591 – 621 .
- Ziskind , G. , Fichman , M. and Gutfinger , C. 1995 . Resuspension of Particulates from Surfaces to Turbulent Flows—Review and Analysis . J. Atmos. Sci , 26 : 613 – 644 .