Abstract
The aerosol aspiration into a thin-walled sampler horizontally oriented in low windspeed environment is numerically investigated. The air flow is assumed to be incompressible and either potential or viscous. Depending on the model the velocity field is computed either by the boundary element or by the finite volume method. The aspiration efficiency A is calculated in the region of a very small ratio R a of wind to sampling velocities when the gravity influence becomes noticeable. The numerical results are compared with the known experimental data and approximate formulas. It is shown that a combination of approximate formulas for aspiration efficiencies in calm and moving air proposed by CitationSu and Vincent (2004b) and CitationMedvedev (2002) gives a fair approximation of the numerical results in the range of small R a.
INTRODUCTION
The low wind velocity regime, intermediate between the moving and calm air cases, is important for practical indoor aerosol sampling. In indoor environments the air flow velocity is about 0.1–0.2 m/s and the settling velocity V s of coarse particles can reach the values that are close to gas velocity (for particles with a size of 80 μ mV s ∼ 0.17 m/s (CitationSchmees et al. 2008)). This means that the particle velocity can be comparable with V s and the gravity force affects the aspiration efficiency.
The influence of the gravity force on the aerosol sampling from a slow moving air has been investigated experimentally by CitationGrinshpun et al. (1990) and theoretically by CitationWen and Dunnet (2003). Both works are devoted to the case of a tube sampler facing vertically upwards in the air flow that moves vertically downwards. For the vertically upward oriented sampler the influence of gravity gives an increase of aspiration efficiency due to additional entry of particles into the tube.
So, there is a gap in the knowledge of performances of horizontally oriented samplers operating in a slow moving air. But the study of aspiration into horizontally oriented samplers is important in order to understand the aerosol inhalability in indoor environments. CitationSchmees et al. (2008) presented some of preliminary experiments with a human mannequin in a new wind tunnel that provides a uniform air flow for an ultra low windspeed in the range below 0.5 m/s. It was found that the results of aspiration efficiency measurement of a personal sampler located on the mannequin lie close to those obtained previously for faster moving air.
To get full understanding of the aerosol aspiration in the range of small ratio of wind and sampling velocities, the intensive theoretical studies are needed. As the first step in this direction we have studied numerically the performances of a thin-walled sampler operating in a very low wind environment. Thin-walled samplers have been widely investigated previously and there is a large set of experimental data to validate the developed mathematical model.
The review of the works devoted to the aerosol sampling into the thin-walled tubes is given by CitationVincent (2007) and CitationGrinshpun et al. (1993). The majority of the investigations are related to the sampling from a moving air. To calculate the aspiration efficiency of thin-walled tube, the potential flow model was used in CitationVitols (1966) and CitationYan et al. (1994). The numerical solution of the Navier-Stokes equations was applied to study the aerosol aspiration into the tube in the moving air by CitationRader and Marple (1988), CitationLiu et al. (1989), CitationMedvedev (2002), and CitationKramer and Afshine (2004). In the work of CitationBelyav and Levin (1974) an approximate formula based on the experimental data for the aspiration efficiency in the range 0.18 < R a < 6 was proposed. Later this formula was validated by many numerical investigations, and a nice correlation of the aspiration efficiencies calculated by the formula and models was found. The calculations of CitationMedvedev (2002) and CitationKramer and Afshine (2004) show that the formula of CitationBelayev and Levin (1974) poorly describes the aspiration efficiency at very small R a . In the works of CitationGibson and Ogden (1977) and CitationDavies and Subari (1982) a nonmonotonic behavior of the dependence A(R a ) was observed for R a < 0.2. To describe the experimental data CitationDavies and Subari (1982) proposed an approximate dependence A(R a , St) that gives the increase of A as R a tends to zero.
All studies mentioned above were made without consideration of the gravity influence. The investigation of the gravity influence on the aspiration efficiency of a horizontally oriented sampler in low wind environment is the aim of the present article. The problem of aerosol sampling into a thin-walled tube facing the wind is solved by using the models of potential and viscous flow of an incompressible fluid. By neglecting the influence of particles on the carrier phase due to small concentration, the fluid velocity fields are obtained by the boundary element method for a potential flow and by using the CFD code FLUENT (version 6.3.26, Fluent Inc., NH) for solving numerically Navier-Stokes equations for a viscous flow. The aspiration efficiency in the range of small values of the ratio R a without and with gravity contribution is calculated on the base of the limiting particle trajectory method.
FORMULATION OF THE PROBLEM
The aerosol flow into a thin-walled tube with radius R t and infinite length is considered (). The sampling velocity U s (the average velocity at the interior cross-section of the tube on the large distance from the inlet) and wind velocity U 0 far from the sampler are directed along the X-axis that coincides with the axis of symmetry of the tube. Due to axial symmetry, the upper half of the meridian plane (X,R) is calculated (), (X,R are the axial and radial coordinates).
In the undisturbed flow far from the sampler, the particles move along the direction determined by the velocity vector
1 =
0 +
s
(
s
= τ
is the settling velocity) (). Let S
p
be the area of the cross-section of the limiting particle trajectory tube far from the sampler. The limiting particle trajectory surface divides the aerosol flux into two parts: one is sampled into the tube and the other contains the particles that do not enter the sampler. At the known area S
p
and flow rate through the tube Q = U
s
π R
t
2 the aspiration efficiency is calculated by the formula
Under the assumption that the Stokes law of aerodynamic drag is valid, the equations of motion of spherical particles can be written as follows
By solving the Cauchy problem for Equation (Equation4) with the initial conditions at t = 0
NUMERICAL RESULTS
Within the potential flow model the equation for stream function is solved by the boundary element method (CitationYan et al. 1994). For the viscous flow model the Navier-Stokes equations are solved by the finite volume method on the base of the SIMPLER algorithm using the CFD code FLUENT. The tube radius is R t = 0.005 m, the sampling velocity is U s = 1 m/s. The sizes of the calculation domain L 1 = 20R t , L 2 = 40R t , L 3 = 20R t are taken so large that their further increase does not affect the solution. Meshing the calculation domain provides the convergence of the numerical solution with the relative residual of 10− 9. The found gas velocity field is used to solve Equations (4) with initial conditions (5). In integrating the equations of particle motion, the axial and radial components of the air velocity are determined by interpolating the velocity field obtained from the solution of the Navier-Stokes equations.
The Case g = 0
If the gravity force is absent, then the cross-section of the surface of limiting particle trajectories far from the sampler has a circular shape and its area can be found by calculating the single limiting trajectory in meridian plane (x,r). The value r 0 of the initial ordinate of the limiting trajectory allows us to find the aspiration efficiency by the formula A = r 0 2 R a . Two types of the aspiration efficiency A i and A e are calculated. Pure inertial aspiration efficiency A i is found on the base of the limiting particle trajectory that hits the tube edge. To find the overall aspiration efficiency A e , which takes into account the rebound of particles from outer wall of the tube, the limiting particle trajectory that hits the flow stagnation point N is calculated. The aspiration efficiency A e is determined under the assumption that all particles impacted on the tube wall between the tube edge and the point N will bounce and enter the tube. In reality, some of the particles impacted on the outer wall will not bounce and will not enter the sampler, depending on particle and wall properties or hydrodynamic conditions. Because of this, the aspiration efficiency, which accounts for the secondary aspiration, will be less than A e .
The dependences of A i and A e on the parameter R a for two carrier phase models at various Stokes numbers are shown in . The dependence A i (R a ), found within the viscous flow model is slightly higher than the corresponding dependence, calculated within the potential flow approximation. This difference becomes noticeable at small Stokes numbers (St0 = 0.1). The streamlines in the vicinity of the tube edge found by two flow models differ at small values R a (). For the viscous flow model the fluid vortex is generated near the tube edge. Because of this, the corresponding limiting particle trajectories that hit the tube edge noticeably differ (). The fluid velocity distributions in the vicinity of the stagnation point N, located far from the tube edge, are, however, practically the same for both models. This gives close dependencies A e (R a ), found from the potential and viscous flow approaches, for various Stokes numbers.
The aspiration efficiencies A i and A e for thin-walled tube at St = 0.22 and 0.76 within the potential flow model are numerically investigated by CitationDunnett (1990). The calculations show that the influence of secondary aspiration appears at R a < 0.5. The results of our calculations in a wide range of the Stokes number St = 0.1–10 confirm this conclusion ().
Two well-known works of CitationGibson and Ogden (1977) and CitationDavies and Subari (1982) presented the results of experimental studies of the aerosol sampling in the moving air for the range of small values R a up to R a = 0.02. Experimental dependencies A(R a ) at various St showed nonmonotonic behavior with decrease of R a . The comparison of dependencies A i (R a ) and A e (R a ) calculated within the two developed models with experimental data of CitationGibson and Ogden (1977) and CitationDavies and Subari (1982) is given in . As was mentioned above we can assume that the curve A e (R a ) is the aspiration efficiency found taking into account the secondary aspiration while A i (R a ) gives the aspiration efficiency without contribution of the particle bounce from sampler surface. Our numerical model predicts the increase of the aspiration efficiencies A i (R a ) and A e (R a ) in the region of a small velocity ratio as it was observed in experiments. It is seen that the A i (R a ) and A e (R a ) slightly differ at St = 0.174 and 0.625. This means that the influence of secondary aspiration is minor in these cases. Both curves A i (R a ) and A e (R a ) are in a fair agreement with the experimental data. At larger values of the Stokes number, St = 1.79 and 2.935, the influence of secondary aspiration increases due to the increase of the number of the particles that hit the sampler surface. Because of this, the experimental data are closer to the curve A e (R a ). In the work of CitationGibson and Ogden (1977) the maximal value of the dimensionless settling velocity was R c = 0.012 while the minimal value of the velocity ratio was R a = 0.03. In CitationDavies and Subari (1982) these values were R c = 0.017 and R a = 0.06, correspondingly. This means that the condition R c R a , when gravity influence can be neglected, is fulfilled.
Note that our numerical model gives higher values of aspiration efficiency for a small velocity ratio than the values observed in experiments of CitationGrishpun et al. (1990). The increase of aspiration efficiency at R a < 0.2 is explained in CitationGrishpun et al. (1990) by the secondary aspiration influence. Special experiments made in this work with greased outer tube wall (with the aim of avoiding the particle bounce) give a monotonic decrease of A i (R a ) that agrees with the formula of CitationBelyaev and Levin (1974). From the dependencies A i (R a ), presented in and , it is seen that the increase of the aspiration efficiency at small values of R a may be caused by primary aspiration without the influence of particle bounce. The particle rebound from the outer wall amplifies this increase.
FIG. 6 The comparison of the dependencies A i (R a ) and A e (R a ) with experimental data from CitationGibson and Ogden (1977) and CitationDavies and Subari (1982).
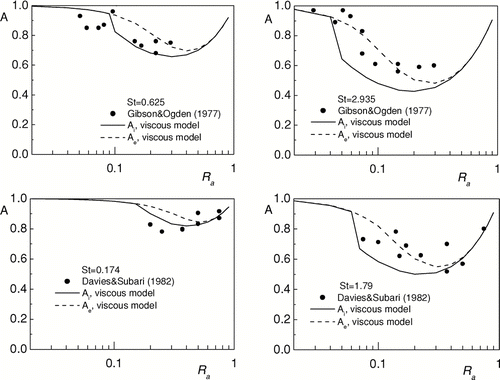
It is interesting to compare the dependencies A i (R a ) and A e (R a ) obtained from the developed numerical models with the known approximate formulas for thin-walled samplers in the range of small values R a . Presently, there are several formulas for the dependence of aspiration efficiency on the Stokes number and parameter R a that are recommended by various authors in the region R a < 1. All formulas have the form
TABLE 1
The comparison of approximate dependencies of aspiration efficiencies from and dependencies A i (R a ) and A e (R a ), calculated from present numerical models is given . It is seen that the theoretical curves A i (R a ) in the small R a region correlate better with coefficients A 1 and A 4 for the Stokes numbers St0 = 0.1,1,10. The coefficient A 2 (the formula by CitationBelyav and Levin (1974)) is close to the curve A i (R a ) at St0 = 10. The approximate formula A 3 of CitationDavies and Subari (1982) agrees with the curve A e (R a ) only for St0 = 0.1. At large values of St0 the curves A e (R a ) and A 3(R a ) differ considerably. The coefficient A 3 tends to 1 at R a → 0 while the limits of A i (R a ) and A e (R a ) at R a → 0 are less than unity. The conclusion can be made from this analysis that the formulas of CitationDavies (1982) and CitationMedvedev (2002) can be used to estimate the aspiration efficiency of thin-walled sampler in the region of a small velocity ratio.
The Gravity Force Influence
In the presence of the gravity force a 3D problem for particle trajectories is solved in the potential flow approximation. The cross-sectional areas of limiting particle trajectories surface far from the sampler with decrease of R
a
from R
a
= 0.2 up to R
a
= 0 at St = 1 and R
c
= 0.1 are shown in . In order to find areas S
p
, the particle paths are calculated beginning from the points uniformly distributed in planes orthogonal to the direction of the velocity vector
1. The maximal number of initial points of trajectories that provides a good accuracy of the calculation of the aspiration efficiency was equal 200*100 in two directions (the symmetry of S
p
is taken into account). The initial points of sampled particles forms the area S
p
that is shown in . The used value of St is chosen to demonstrate the most interesting behavior of areas S
p
with changing the velocity ratio. The initially circular cross-sectional area S
p
(R
a
= 0.2) changes its size and shape. The increase of the size of areas S
p
is connected with decreasing the velocity ratio. Their shapes are changed by gravity action. A narrow area of sampled particles appears near the main circle at R
a
= R
c
= 0.1. helps to understand the cause of appearance of a doubly connected area S
p
. It is seen that there are two zones of the trajectories of sampled particles in the meridian plane. The zone of the sampled particles is separated by the zone of trajectories that are deposited on the lower part of the outer wall of the tube. The same phenomena for spherical sampler operating in still air was observed and described by CitationGaleev and Zaripov (2003). At R
a
= 0.08 both areas are connected. The common area S
p
is extended and there appears the area of starting points of particles deposited on the upper part of the outer wall of the tube inside the area S
p
(R
a
= 0.04). The shape of area S
p
at R
a
= 0 is the same as in the works of CitationSu and Vincent (2004a) and Dunnet (1992) where calm air sampling into the tube was investigated. The obtained areas are used to calculate the aspiration efficiency by Formula (1).
FIG. 8 The cross-sectional area of the limiting particle trajectory surface far from the sampler at St = 1 and various R a .
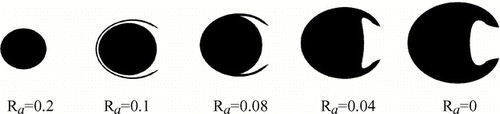
FIG. 9 The particle trajectories at St = 1 for R a = 0.1. Dashed lines are the trajectories of particles deposited on the sampler surface.
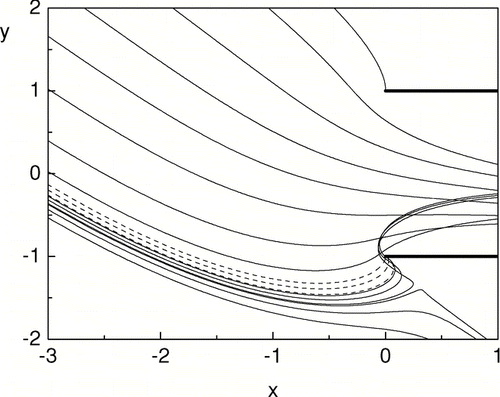
The dependencies A(R a ), calculated with and without gravity influence at various Stokes numbers for fixed value of Fr are given in . The chosen value of the Froude number corresponds to the values R c = 0.01,0.1,1 at the values of St = 0.1,1,10. As was mentioned above, the size and shape of the areas S p and thus the aspiration efficiency depend on the velocity ratio and gravity force. The influence of gravity is practically absent for small values of St and R c (St = 0.1, R c = 0.01). For larger values of St and R c the gravity force noticeably affects the aspiration efficiency at R c > R a . For St = 1 and R c = 0.1 the aspiration efficiency calculated taking into account the gravity force slightly higher than one without gravity in the region R c ∼ R a . It is due to the contribution of a narrow area of sampled particles around the main circular area S p . Further, the area S p decreases due to subtracting the area of initial positions of particles that are deposited on the upper part of the tube surface. In this case the gravity decreases the aspiration efficiency. For St = 10 and R c = 1 the dependence of the aspiration efficiency on R a calculated without gravity is higher than the curve A(R a ) found with gravity contribution in the entire range R a ∈ [0,1]. The influence of gravity action for various values of R c and fixed value of St can be seen from . Note that the variation of R c = 0.1,0.2,0.5 at fixed St = 1 for the sampler with R t = 0.005 m corresponds, for example, to the combinations of water droplets of diameters and the sampling velocities: d ∼ 57 μm, U s ∼ 0.99 m/s; d ∼ 68 μm, U s ∼ 0.7 m/s; d ∼ 85 μm, U s ∼ 0.44 m/s.
FIG. 10 The dependencies of the aspiration efficiency on R a with and without gravity influence for various St and fixed Fr (a) and for fixed St and various R c (b).
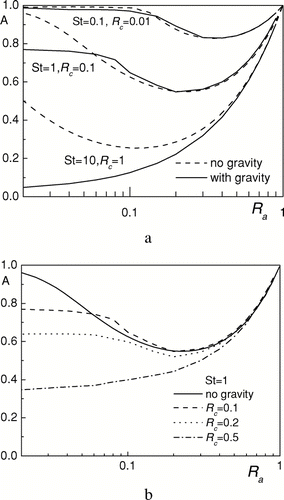
As R a decreases up to zero the aspiration efficiency, calculated taking into account gravity action, tends to the values that correspond to the calm air sampling. Our results at R a = 0 are in agreement () with the semi-empirical formula for aspiration efficiency of the thin-walled tube oriented horizontally in calm air proposed by CitationSu and Vincent (2004b)
The curves A(R a ) for the range R a ∈ [0,1] in the case of gravity action can be approximated by Formula (8) that is the linear combination of the aspiration efficiencies A 0 for sampling in calm air (7) and A m = A 4 for that in the moving air (CitationMedvedev 2002)
CONCLUSIONS
The aspiration efficiency for the thin-walled sampler operating in low windspeed environment is numerically investigated using the viscous and potential flow models for describing the air flow. It is shown that the difference of the aspiration efficiencies found by two models appears in the region of low ratio of wind and sampling velocity at small Stokes numbers. The known formula of CitationBelyaev and Levin (1974) gives for small velocity ratio the values of the aspiration efficiency that are less than those obtained from the numerical model. The aspiration efficiency in this region at various Stokes numbers is rather well described by the formulas of CitationDavies (1968) and CitationMedvedev (2002). The influence of gravity for horizontally oriented sampler decreases the aspiration efficiency for the velocity ratio less than the non dimensional settling velocity. The linear combination of the aspiration efficiencies for the calm and moving air can be used to estimate the aspiration coefficient with gravity contribution in the range R a ∈ [0,1].
Acknowledgments
The work was supported by the RFBR (grants No. 07-07-00183, 08-01-00163).
REFERENCES
- Belyaev , S. P. and Levin , L. M. 1974 . Techniques for Collecting of Representative Aerosol Samples . J. Aerosol Sci. , 5 : 325 – 338 .
- Davies , C. N. and Subari , M. 1982 . Aspiration Above Wind Velocity of Aerosols with Thin-Walled Nozzles Facing and at Right Angles to the Wind Direction Sampler . J. Aerosol Sci. , 13 : 59 – 71 .
- Davies , C. N. 1968 . The Entry of Aerosols Into Sampling Tubes and Heads . British J. of Appl. Phys. , 25 : 921 – 932 .
- Dunnett , S. J. 1992 . A Mathematical Study of the Sampling Characteristics of A Thin-Walled Sampler in Calm Air . Aerosol Sci. Tech. , 17 : 93 – 104 .
- Dunnett , S. J. 1990 . A Mathematical Study of Particle Rebound for Thin-Walled Samplers . Aerosol Sci. Tech. , 12 : 686 – 693 .
- Galeev , R. S. and Zaripov , S. K. 2003 . Theoretical Study of Aerosol Sampling by an Idealised Sampler in Calm Air . J. Aerosol Sci. , 34 : 1135 – 1150 .
- Gibson , H. and Ogden , T. L. 1977 . Some Entry Efficiencies for Sharp-Edged Samplers in Calm Air . J. Aerosol Sci. , 8 : 361 – 365 .
- Grinshpun , S. , Lipatov , G. N. and Sutugin , A. G. 1990 . Sampling Errors In Cylindrical Nozzles . Aerosol Sci. Tech. , 12 : 716 – 740 .
- Grinshpun , S. , Willeke , K. and Kalatoor , S. 1993 . A General Equation for Aerosol Aspiration by Thin-Walled Sampling Probes in Calm and Moving Air . Atmos. Environ. , 27A : 1459 – 1470 .
- Kramer , M. and Afshine , A. 2004 . Sampling Characteristics of Inlets Operated at Low U/U 0 Ratios: New Insights from Computational Fluids Dynamics (CFX) Modeling . J. Aerosol Sci. , 35 : 683 – 694 .
- Liu , B. Y. H. , Zhang , Z. Q. and Kuehn , T. H. 1989 . A Numerical Study of Inertial Errors in Anisokinetic Sampling . J. Aerosol Sci. , 20 : 367 – 380 .
- Medvedev , A. A. 2002 . Numerical Study of Aerosol Particle Sampling from a Low-Speed Flow . Atmospheric and Oceanic Optics. , 15 : 663 – 666 .
- Rader , D. J. and Marple , V. A. 1988 . A Study of the Effects of Anisokinetics Sampling . Aerosol Sci. Tech. , 8 : 283 – 299 .
- Schmees , D. K. , Wu , Y.-H. and Vincent , J. H. 2008 . Experimental Methods to Determine Inhalability and Personal Sampler Performance for Aerosols in Ultra-Low Windspeed Environments . J. Environ. Monit. , 10 : 1426 – 1436 .
- Su , W. C. and Vincent , J. H. 2004a . Experimental Measurements and Numerical Calculations of Aspiration Efficiency for Cylindrical Thin-Walled Aerosol Samplers in Perfectly Calm Air . Aerosol Sci. Tech. , 38 : 766 – 781 .
- Su , W. C. and Vincent , J. H. 2004b . Towards a General Semi-Empirical Model for the Aspiration Efficiencies of Aerosol Samplers in Perfectly Calm Air . J. Aerosol Sci. , 35 : 1119 – 1134 .
- Vincent , J. 2007 . Aerosol Sampling: Science, Standarts, Instrumentation and Applications , 616 John Wiley & Sons .
- Vitols , V. 1966 . Theoretical Limits of Errors Due to Anisokinetic Sampling of Particulate Matter . J. Air Pollution Control Association. , 16 : 79 – 84 .
- Wen , X. and Dunnett , S. 2003 . The Aspiration Efficiency of a Tube Sampler Operating in a Slow Moving Air Stream Facing Vertically Upwards . J. Aerosol Sci. , 34 : 1235 – 1244 .
- Yan , B. and Dunnett , S. J. 1994 . A Numerical Investigation Into the Sampling Performance of a Tube Sampler . Aerosol Sci. Tech. , 20 : 291 – 302 .