Abstract
Modern bioaerosol sampling and analysis techniques that enable rapid detection of low bioagent concentrations in various environments are needed to help us understand the causal relationship between adverse health effects and bioaerosol exposures and also to enable the timely biohazard detection in case of intentional release.
We have developed a novel bioaerosol sampler, an electrostatic precipitator with superhydrophobic surface (EPSS), where a combination of electrostatic collection mechanism with superhydrophobic collection surface allows for efficient particle collection, removal, and concentration in water droplets as small as 5 μ L. The sampler's performance at different sampling flow rates and sampling times was tested with two common bacteria: Pseudomonas fluorescens and Bacillus subtilis. The collection efficiency was determined using the traditional method of microscopic counting as well as the whole-cell quantitative real-time polymerase chain reaction assay (QPCR). The tests indicated that the new sampler achieves collection efficiency as high as 72%. A combination of the satisfactory collection efficiency and the small collecting water droplet volumes allowed achieving sample concentration rates that exceed 1 × 10 6 . In addition, the collection efficiency for both bacteria obtained by the two different methods was not statistically different, indicating the sampler's compatibility with the PCR-based sample analysis techniques. In addition, the whole-cell QPCR does not require DNA extraction prior to the PCR reaction which offers faster sample processing.
Very high concentration rates achieved with the new sampler as well as its compatibility with the QPCR methodology point toward its suitability for detecting low concentrations of airborne bacterial agents in various environments.
INTRODUCTION
The interest in estimating bioaerosol exposure has increased over the past few decades when it was realized that many adverse health effects such as infections, hypersensitivity pneumonitis, and toxic reactions are associated with bioaerosol exposure occurring in various domestic and industrial sector (CitationBurge et al. 1989; CitationKarol 1991). A significant relationship was found between the reduction in lung function and airborne mold concentrations (CitationDahlqvist et al. 1992). Respiratory symptoms, febrile episodes, allergic diseases such as hypersensitivity pneumonitis, and asthma due to bioaerosol exposure have been documented among workers employed in waste industry (CitationChiba et al. 2009; CitationPoulsen et al. 1995). It was also found that not only viable, but also non-viable bioaerosol particles are capable of causing adverse health effects (CitationAdhikari et al. 2009; CitationGorny et al. 2002; CitationRobbins et al. 2000).
The presence of bioaerosols in various environments is determined by sampling airborne particles and analyzing the sample by various techniques. Our desire to better understand the relationship between bioaerosol exposures and health effects as well as the need to detect harmful biological agents in case of their malicious release reinforced the need to develop and test bioaerosol monitoring systems capable of early and reliable detection of biological agents in near real-time. The development of such systems was spurred by anthrax attacks of 2001. A monitoring system satisfying the requirements above should feature a particle collection unit capable of detecting low bioaerosol concentrations and an analysis unit capable of fast and reliable identification and quantification of total (viable and non-viable) bioaerosol particles. One possible schematic of such a system is shown in . Ideally, the system should also have a small footprint and low power requirements for its wide distribution. If the system is used for extended sampling times with multi-day archiving periods, inclusion of a monitor for DNA intactness would also be desirable. A system satisfying these requirements would enable early detection of bioaerosol release and would also help us develop a dose-response relationship for bioaerosol exposures which is currently lacking.
The liquid-based collectors usually feature satisfactory collection efficiency, allow sample analysis by multiple techniques (CitationEduard and Heederik 1998), and thus are candidates for the system suggested in . A key parameter defining their ability to detect low concentrations of bioaerosol particles is their concentration rate (a ratio of particle concentration in the collection liquid versus the airborne particle concentration per time unit). Several new samplers capable of providing a concentrated sample in a small volume of liquid have recently been suggested. For example, a wetted-wall bioaerosol cyclone (CitationSeo 2007; CitationHu and McFarland 2007) has concentration rates in the range of 5 × 105 − 6 × 105 for 1 μ m PSL particles. This cyclone features a continuous liquid outflow rate of 1 mL/min. A combination of a virtual impactor and a wetted-wall cyclone of the autonomous pathogen detection system (APDS) developed by the Lawrence Livermore National Laboratory (LLNL) can achieve concentration rates of 7.5 × 105 when sampling inert 3 μ m particles (CitationMainelis et al. 2005); among the electrostatic precipitators, a briefcase-sized sampler (CitationCarlson et al. 2004) has concentration rates of about 1.5 × 104. Our earlier study presented the Electrostatic Precipitator with Superhydrophobic Surface (EPSS), which combines an electrostatic collection technique with superhydrophobic collection surface and allows efficient particle collection, removal and concentration in water droplets from 5 to 40 μ L (CitationHan and Mainelis 2008). When collecting polystyrene latex particles 3 μ m in diameter, this sampler has achieved a concentration rate of 1 × 106. Given the high concentration rate and low power requirements of this sampler, it makes a good candidate for the detection system shown in . However, the efficiency of this sampler has not yet been tested with biological particles.
Another key component in any bioaerosol detection system is sample analysis. The widely used culture-based analysis method which reports the results as Colony Forming Units (CFUs) is potentially very sensitive (one organism), but time consuming, and organisms may become damaged and non-culturable during sample collection (CitationButtner et al. 1991; Martinez et al. 1988; CitationLin et al. 2000). Moreover, the majority of naturally occurring microorganisms cannot be cultivated using standard culture methods (≥ 90–99%) or readily identified in culture (CitationAmann et al. 1995; CitationDeLong and Pace 2001). Therefore, a fast analysis method providing a total cell concentration would be a better candidate for the monitoring system suggested in . Quantitative real-time PCR (QPCR) allows not only the identification of collected species, but also their quantification, once proper standard (calibration) curves have been prepared. The conventional QPCR has been incorporated into bioaerosol detection systems, such as the APDS (CitationHindson et al. 2005). Our earlier study showed that to improve the accuracy of the bioaerosol quantification, the calibration curves should to be study-specific, i.e., they should take into account the sampling device, target bioaerosol, its counting method, and the DNA preparation method for the PCR reaction (CitationAn et al. 2006). We also showed that the QPCR analysis could be simplified and accelerated by not extracting the genomic DNA, but by using the whole-cells in the reaction (whole-cell QPCR) (CitationAn et al. 2009). As such, the whole-cell QPCR analysis method would be a candidate for an advanced bioaerosol monitoring system shown in .
Thus, the main goal of this study was to analyze the performance of the Electrostatic Precipitator with Superhydrophobic Surface (EPSS) when collecting biological aerosols and its compatibility with the whole-cell QPCR. The data obtained using the whole-cell QPCR were compared with those obtained with a more traditional total cell counting method using acridine orange epifluorescence microscopy (AOEM). The settings of the EPSS collection voltage and charging conditions were the same as in the previous study (CitationHan and Mainelis 2008) and here its performance was tested as a function of the collecting water droplet size (5 and 40 μ L), different sampling flow rates (2, 5, and 10 L/min) and the sampling time period (10, 30, and 60 min). The experiments were performed with vegetative cells of two different, commonly used test microorganisms: Gram-negative Pseudomonas fluorescens as a sensitive organism and Gram-positive Bacillus subtilis var. niger, as a hardy organism. In addition to determining the sampler's performance, the obtained data were also used to determine the feasibility of the suggested sampling and analysis methods for incorporating them into the bioaerosol monitoring system suggested in .
MATERIALS AND METHODS
Biological Test Particles
The test microorganisms used in this study, P. fluorescens and B. subtilis are representatives of sensitive and hardy organisms, respectively, and are commonly found in indoor and outdoor environments (CitationHill et al. 1999; CitationJohnson et al. 1994; CitationNeidhardt et al. 1990). P. fluorescens (ATCC 13525) were obtained from the American Type Culture Collection (Rockville, MD) and grown in nutrient broth (Becton Dickinson Microbiology Systems, Cockeysville, MD) at 26°C for 18 h in a shaking incubator. After the growth, the cells were harvested by centrifugation at 7000 rpm (6140 g) for 5 min, at 4°C (BR4, Jouan, Winchester, VA) and then washed 3 times with sterile deionized water under the same conditions. Prior to the experiments, the resulting cell pellet was resuspended in sterile deionized water to obtain suspension with the target cell concentration of ∼ 109 cells per mL, as determined by the epifluorescence microscopy.
Dry B. subtilis spores were obtained from the US Army Edgewood Laboratories (Edgewood Research, Development and Engineering Center, Aberdeen Proving Ground, MD). The B. subtilis spores were suspended in sterile deionized water, and then activated at 60°C for 25 min. The activated B. subtilis was cultured in nutrient broth for 18 h at 30°C to obtain vegetative B. subtilis. Prior to the experiments, freshly grown vegetative B. subtilis were prepared using the same method as described above.
Experimental Setup and Sample Collection
The test sampler, EPSS, is described in detail in our previous publication (CitationHan and Mainelis, 2008). Briefly, it has the shape of a closed half-pipe, where the top surface serves as a ground electrode while the narrow collection electrode (3.2 or 2.1 mm width and 254 mm length) covered by a superhydrophobic substance is positioned on the opposite plate slightly below (0.3 to 0.5 mm) the surface of the plate for improved guidance of the collecting droplet. As the positively charged particles are drawn into the sampler, they are deposited on the collection electrode by the electrostatic field action. After the collection, a tiny liquid droplet (5 or 40 μ L) introduced at the top of the collection electrode rolls down under gravity and removes the deposited particles.
The experimental setup is shown in . The test bacteria were aerosolized using a 6-jet Collison nebulizer (BGI Inc., Waltham, MA) operated at a flow rate Q A (4 L/min) and diluted with HEPA-filtered air flow Q D (36 L/min). The 40 L/min aerosol stream was passed through a 2-mCi Po-210 charge neutralizer. The electrically neutralized bioaerosols then passed through a 0.035 m duct housing a vertically oriented ionizer (Wein Products Inc., Los Angeles, CA) which imparted a positive charge on the particles under controlled operating conditions (12 V/50 mA). The positively charged bioaerosols passed through a flow straightener and entered the test chamber where they were collected by the EPSS operated at a sampling flow rate, Q S (2, 5, or 10 L/min). After a certain sampling time, t (10 to 60 min), the bacterial particles deposited on the superhydrophobic surface were removed by a rolling water droplet (5 or 40 μ L) which was collected in a vial. The collected cell number was determined using the epifluorescence microscopy and the whole-cell QPCR assay as described below.
FIG. 2 Schematic diagram of the experimental setup. The figure in left bottom corner shows an alternative set-up used to determine the bioaerosol reference concentration.
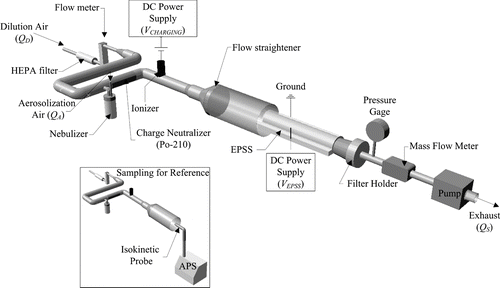
For each test, about 30 mL of fresh cell suspension was prepared for nebulization as described above. The 3.2 mm wide collection electrode was used for 40 μ L droplets, while the 2.1 mm electrode was used for 5 μ L droplets. Each collecting electrode of the EPSS was prepared by coating it with a superhydrophobic spray (HIREC-1450, NTT Corporation Inc., Japan) followed by drying at 60 °C for at least 1 hour. Before each coating, the electrode was thoroughly rinsed with ethyl acetate and then autoclaved. One stable DC power supply (BK Precision, Yorba Linda, CA) provided power to the ionizer, while another stable DC high voltage power supply (Bertan Associates, Inc., Valhalla, NY) provided collecting voltage to the EPSS (−7 kV). The operating values for these two voltages were established in the previous study as yielding the most efficient deposition of particles inside the EPSS. The entire experimental setup was housed inside a Class II Biosafety cabinet (NUAIRE Inc., Plymouth, MN).
Determination of Total Bacterial Number
The total number of cells collected by the EPSS and removed by the droplet was determined by both acridine orange epifluorescence microscopy using the Axioskop 20 (Carl Zeiss MicroImaging Inc., Thornwood, NY) and the whole-cell quantitative real-time PCR (whole-cell QPCR) assay.
When counting cells using the epifluorescence microscopy, the droplet (5 or 40 μ L) containing particles collected by the EPSS was diluted by adding sterile and purified water to increase the liquid volume to 1 mL. The resulting 1 mL sample was serially diluted in 10-fold dilutions with sterilized water to achieve a concentration comfortably countable using the epifluorescence microscopy. Each slide was prepared by filtering 1 mL aliquot of a selected dilution through a 25 mm black polycarbonate filter (Fisher Scientific, Suwannee, GA) and then staining it with 1 mL of 0.1 μ g/mL Acridine Orange solution (Becton Dickinson Microbiology Systems, Sparks, MD) for 10 min. After washing with 3 mL of sterilized water and air-drying, at least 20 microscope fields were counted twice using the 100× oil-immersion objective. The total cell number in each sample, C sample , was calculated as follows:
Here, N is the average cell count in each microscope view field, X is the number (X = 6125) of fields for the entire filter, and D 10 − fold is the dilution factor.
When a biological sample is analyzed by the QPCR, the output from the reaction is a threshold cycle (C T ) value which is defined as the PCR cycle number at which the fluorescence of the amplicon exceeds a calculated threshold value. The C T value is inversely proportional to the initial DNA (or cell) concentration with which the reaction was started. Thus, a standard (calibration) curve could be prepared relating known cell concentrations (determined by the epifluorescence microscopy) with the C T values.
Our earlier research indicated that the standard curves should be study specific, i.e., they should be prepared using the same sampling protocol that will be used to collect actual samples (CitationAn et al. 2006). In addition, we have shown that one could use whole-cell QPCR method with a universal primer (CitationAn et al. 2009). Unlike the conventional PCR where DNA has to be extracted from cells prior to the PCR reaction, in this method aliquots from untreated cell suspensions are directly used in the PCR reaction as templates. Absence of the DNA extraction step increases the labor and time-efficiency of the method.
When preparing the standard curves for the whole-cell QPCR, the volume of samples collected by the EPSS (5 μ L) was increased to 1 mL. From this 1 mL sample suspension, 100 μ L aliquot was transferred to a sterilized microcentrifuge tube and serially diluted in 10-fold water-based dilutions ranging from 100 to 10− 5 until concentration countable by the microscopy was achieved. The number of cells in each dilution was determined by the epifluorescence microscopy as described above and then related to the whole-cell QPCR output (C T value) at each dilution. During the PCR reaction, 5 μ L aliquots of each dilution were directly (without extracting genomic DNA first) used as templates and the reactions were performed in triplicate. QPCR amplification was performed using iCycler iQ™ Real-Time PCR Detection System (Bio-Rad Laboratories, Hercules, CA) using the iQ SYBR Green supermix PCR Kit (Bio-Rad Laboratories, Hercules, CA). Amplification and detection of DNA by real-time PCR was performed on iCycler iQ™ Real-Time PCR Detection System (Bio-Rad Laboratories, Hercules, CA) using the iQ SYBR Green supermix PCR Kit (Bio-Rad Laboratories, Hercules, CA) consisting of 2× SYBR Green supermix (with the hot-start enzyme, iTaq™ DNA polymerase, SYBR PCR buffer, dNTP mix, SYBR I, 20 nM fluorescein for dynamic well factor collection, and 6 mM MgCl2). Reaction mixes were prepared by combining 12.5 μ L of 2× SYBR Green supermix, primers and H2O to a total volume of 25 μ L for each reaction. The universal primers originally described by CitationNadkarni et al. (2002) including the forward primer, 5′ -TCCTACGGGAGGCAGCAGT-3′ and the reverse primer, 5′ -GGACTACCAGGGTATCTAATCCTGTT-3′ were used at final concentration of 0.25 μM for each primer and produced 466 bp amplicon (between residues 331 and 797 on the E. coli 16S rRNA gene). Amplification reaction was performed using the following program with iCycler iQ™ thermal cycler (Bio-Rad Laboratories, Hercules, CA): 10 min at 95°C; 40 cycles of (15 s at 95°C 1 min at 60°C). Data analysis was performed using iCycler iQ™ Real-Time detection system software. After completing the PCR amplification cycles, a melt curve was generated for the resulting amplicon by measuring loss of fluorescence over a temperature range of 55–92°C. If there is a contamination during the reaction, a peak, other than desired amplicon peak, would appear in melting curve thus indicating the contamination of DNA, non-specific binding, or occurrence of primer dimers. Additional information about the primer set and PCR amplification conditions are provided in our previous study (CitationAn et al. 2006). Non-specific PCR products from bacterial samples, such as primer dimers or false priming amplicons were not observed in melt curves. If a negative control (no template added in PCR reaction) had C T values of ≤ 30, the C T values from the entire reaction were discarded. The standard curves and the resulting equations obtained for the P. fluorescens and B. subtilis bacteria are shown in . Each point represents an average and standard deviation of triplicate C T values.
When analyzing performance of the EPSS using the QPCR method, the collected bacteria were removed by a 5 μ L droplet and the droplet was used directly in the PCR reaction as a template. The number of collected cells, C sample , was determined using the corresponding C T values and equations shown in :
Determination of the EPSS Collection Efficiency
Before and after each measurement with the EPSS, the reference concentration of the airborne bacteria was determined by an Aerodynamic Particle Sizer (APS, Model 3321, TSI Inc., Shoreview, MN) and an isokinetic probe as shown in bottom left of . The accuracy of the reference concentration measurements was verified by comparing the bacterial concentrations determined by the APS with those measured using a reference filter. Here, the bacteria were isokinetically collected on a 47 mm membrane filter (Pall Inc., East Hills, NY) for 10 min and were then extracted into liquid using the procedure reported by CitationWang et al. (2001): the filter was soaked in 30 mL of sterile deionized water for 10 min, followed by vortexing for 2 min and sonicating for 15 min. The number of bacteria in the resulting suspension was determined using epifluorescence microscopy. It was found that the two number concentrations (APS reading versus reference filter) agreed within 8%. Given the inherent uncertainty in microorganism counting by microscopy (standard deviation is usually ∼ 20%), this was an acceptable agreement. Thus, the collection efficiency, η, of the EPSS for each sampling condition was defined as:
Here, C sample is the number of cells in a water droplet determined either by microscopy or whole-cell QPCR, C reference is the reference cell number measured by the APS, R APS is the average cell concentration (#/cm3) which is measured by the APS every 6 s for 150 s before and after each sampling, Q s (L/min) is the sampling flow rate of the EPSS, and t (min) is the sampling time period.
In addition to the collection efficiency, another metric that describes the performance of a liquid-based aerosol collector is the concentration rate, R C , which represents the ratio of particle concentration in liquid versus the concentration of particles in air per time period. The concentration rate R C with units of min− 1 could be expressed as follows:
Since our earlier investigation (CitationHan and Mainelis 2008) observed that the majority of particles deposited on the collection electrode is removed by the 1st water droplet, the collection efficiency and the concentration rate were calculated based on the 1st water droplet.
RESULTS AND DISCUSSION
The primary goal of this study was to analyze the performance of the Electrostatic Precipitator with Superhydrophobic Surface (EPSS) when sampling bacterial aerosols. Furthermore, we wanted to investigate the compatibility of the sampler with whole-cell QPCR analysis method for their potential use in the bioaerosol monitoring system shown in .
The representative number concentrations and particle size distributions of airborne P. fluorescens and B. subtilis bacterial cells measured by the APS are shown in . As could be seen, the mean aerodynamic diameters, d ae , of the bacteria were 0.82 ± 0.02 μ m and 0.89 ± 0.03 μ m, respectively, and the particle agglomerates were absent.
FIG. 4 Particle size distributions according to the number concentration (Δ N/L) and the normalized concentration (Δ N/Δ logDp, L− 1) for two biological particles: (a) P. fluorescens and (b) B. subtilis.
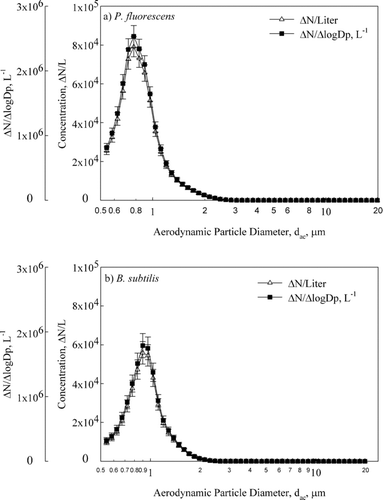
and present the collection efficiency and the resulting concentration rate of the EPSS, respectively, when sampling the two bacteria at different sampling flow rates (2, 5, and 10 L/min) and using water droplets of two different volumes (5 and 40 μ L) to remove the collected particles. The sampling time in these tests was 10 min and the number of collected particles was determined using epifluorescence microscopy. As could be seen from , the collection efficiency of the EPSS when using 40 μ L collection droplets ranged from 52% to 61% for P. fluorescens (d ae = 0.82 μ m) across the sampling flow rates. For B. subtilis (d ae = 0.89 μ m), the collection efficiencies ranged from 59% to 72% for the same sampling flow rates. When the droplet of 5 μ L was used, the collection efficiency averaged over all three sampling flow rates and both bacteria decreased by approximately 7% compared to the 40 μ L droplet. For both sampling droplet sizes the collection efficiency was somewhat higher at the higher flow rates (10 L/min) compared to the lower flow rates (2 L/min). A similar pattern was also observed when testing with the PSL particles and it was attributed to lower particle losses inside the EPSS when sampling at higher flow rates. However, the actual EPSS collection efficiency value when sampling the PSL particles of similar sizes and the same sampling flow rates was lower and ranged from 10 to 40% (CitationHan and Mainelis 2008). Clearly, the EPSS collects the bacterial particles more efficiently than the PSL particles of the same size. The observed efficiency with which the particles entering the EPSS are removed from the air stream is approximately the same for bacteria and PSL. Thus, higher collection efficiency for bacteria is likely achieved by their more efficient removal from the electrode due to weaker adhesion forces between the bacteria and the electrode compared to the adhesion forces for the PSL particles. The tests presented in were conducted in triplicate and the maximum standard deviation was 0.08.
FIG. 5 The collection efficiency of P. fluorescens and B. subtilis bacteria for different volumes of collecting water droplet, (a) 5 μ L and (b) 40 μ L, at three different sampling flow rates (2, 5, and 10 L/min) and at the 12 V/50 mA charging condition and 7 kV collection voltage. The maximum standard deviation is 0.10 from three repeats.
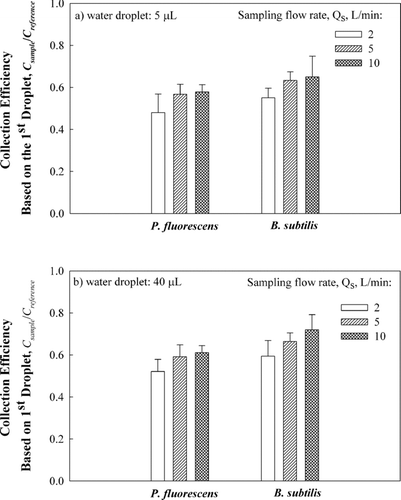
FIG. 6 Comparison of the concentration rates based on the 1st water droplet as a function of droplet size, (a) 5 μ L and (b) 40 μ L, at 2, 5, and 10 L/min sampling flow rates and at the 12 V/50 mA charging condition and 7 kV collection voltage with P. fluorescens and B. subtilis. The error bars represent the standard deviation from three repeats.
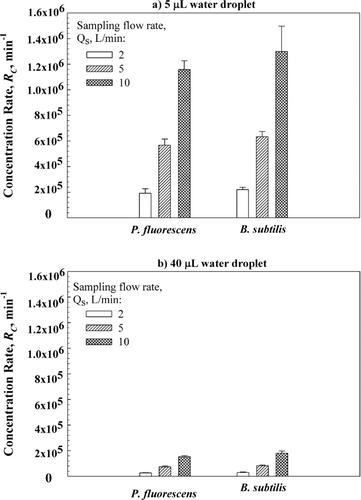
presents the concentration rates based on the collection efficiency data presented in . As could be seen, for the 40 μ L collection droplet and the sampling flow rate of 10 L/min the concentration rate for P. fluorescens was 150,000 and reached 180,000 for B. subtilis. These values are higher compared to the concentration rates of PSL particles of similar size, due to higher collection efficiency for bacteria. For 5 μ L droplet and the sampling flow rate of 10 L/min we achieved sample concentration rates as high as 1.2 million for both bacteria. A sampler featuring such high concentration rates would be able to detect low bioaerosol concentrations and thus is a suitable candidate for the bioaerosol monitoring system suggested in .
For many bioaerosol sampling applications sampling times longer than 10 min are needed. Therefore, in addition to the 10 min sampling time data, performance of the EPSS was tested for sampling times of 30 and 60 min and the data for P. fluorescens and B. subtilis are presented in and , respectively.
FIG. 7 Collection efficiency based on the 1st water droplet, (a) 5 μ L or (b) 40 μ L, as a function of sampling time (10, 30, and 60 min) at 2, 5, and 10 L/min flow rates and at the 12 V/50 mA charging condition and 7 kV collection voltage with P. fluorescens. The error bars represent standard deviations from three repeats.
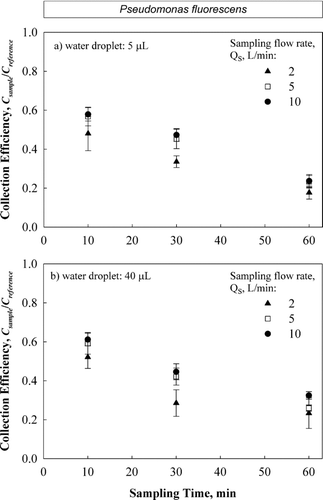
FIG. 8 Collection efficiency based on the 1st water droplet, (a) 5 μ L or (b) 40 μ L, as a function of sampling time (10, 30, and 60 min) at 2, 5, and 10 L/min flow rates and at the 12 V/50 mA charging condition and 7 kV collection voltage with B. subtilis. The error bars represent standard deviations from three repeats.
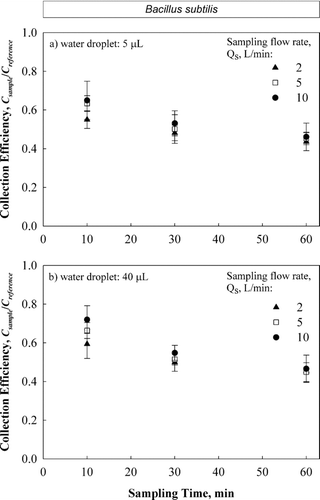
When sampling of P. fluorescens for 30 min was performed with 5 μ L droplet and the flow rates were increased from the lowest (2 L/min) to the highest (10 L/min), the collection efficiency increased from 34 ± 3% to 47 ± 3%; for 60 min sampling, the collection efficiency increased from 18 ± 3% to 24 ± 3% (). However, compared to the 10 min sampling, the collection efficiency after 30 and 60 min decreased by 22% and 60%, respectively, when averaged for all sampling flow rates. The trend for 40 μ L water droplet was similar: the collection efficiency decreased by 33% and 53% for 30 and 60 min sampling time, respectively, compared to 10 min sampling.
The data for B. subtilis presented in show a trend similar to that of P. fluorescens. When sampling for 30 and 60 min, the collection efficiencies with both 5 and 40 μ L water droplets have slightly increased at 10 L/min compared to 2 L/min. However, compared to the 10 min sampling, the collection efficiency decreased for 30 and 60 min sampling, although the decrease was less steep compared to the P. fluorescens bacteria. For 5 μ L water droplet, after 30 and 60 min of sampling, the collection efficiency decreased on average by 17% and 26%, respectively, compared to 10 min sampling time. The average decrease for 40 μ L water droplet was by 21% and 31% for 30 and 60 min sampling time, respectively.
The observed decrease in the collection efficiency for both bacteria with increasing collection time is opposite compared to the experiments with PSL particles, where at longer sampling times the collection efficiency either stayed the same or slightly increased (CitationHan and Mainelis 2008). One possible explanation could be “saturation” of the water droplet by a higher number bacteria deposited on the electrode after a longer sampling time. However, the concentrations of both bacteria were adjusted so that the total number of bacteria deposited inside the EPSS would be approximately the same for all three sampling times. Thus, the most likely explanation of the observed result is a less efficient removal of the deposited bacteria by the water droplet after their prolonged contact with the collection electrode, e.g., increase in the adhesive force with time. The adhesive force between the particles and the surface depends on the particle type, net electrical charge and the contact time (CitationHinds 1999). Although the superhydrophobic surface is supposed to provide a very small surface area contact with the particles (this phenomenon allows for a high water contact angle), it could be expected that occurring physical phenomena will be similar to other surfaces, i.e., electrostatic and van der Waals forces would gradually deform the asperities and increase the contact area thus increasing the adhesion forces (CitationHinds 1999). The phenomenon of the deposited particle adhesion to the EPSS electrode will be examined in more detail in future research.
The collection efficiency of the EPSS was also determined by quantifying the collected cells using the whole-cell QPCR to investigate compatibility of the EPSS with this sample analysis method. Here, an additional set of tests was carried out at a 10 L/min sampling flow rate, 10 min sampling time and the bacteria deposited on the sampler's electrode were collected using 5 μ L water droplets. The voltages for particle charging and collection remained the same as in the previous tests.
For each bacterium, the reference cell number as measured by the APS, the number of collected cells determined using the QPCR and the epifluorescence microscopy, as well as the resulting collection efficiency are presented in . The presented data also show uncertainty of the measurement for each replicate. When calculating the collection efficiency, the error was propagated. As could be seen in , the average collection efficiency of P. fluorescens determined by the QPCR was 66 ± 4%: approximately 15% higher compared to the average collection efficiency determined by microscopy. For B. subtilis, a hardy gram positive bacterium, the collection efficiency determined by microscopy (65 ± 7%) was higher than the average collection efficiency determined by the whole-cell QPCR (54 ± 3%). However, based on the t-test, the difference in the average collection efficiencies when taking into account propagated error was not statistically significant for either P. fluorescens or B. subtilis: p = 0.38 > 0.05 and p = 0.20 > 0.05, respectively.
TABLE 1 Collection efficiency of the EPSS for P. fluorescens and B. subtilis bacteria based on two different methods to quantify the collected bacteria. The data are presented as average ± standard deviation
As could be observed from and , the average collection efficiency determined using cell counting by microscopy (AOEM/APS) for the two separate test sets was the same for B. subtilis, but slightly different for P. fluorescens: 58% versus 51%. However, this difference was not statistically different: P = 0.27 > 0.05.
The data presented in show that the samples collected by the EPSS could be effectively analyzed using the whole-cell QPCR method, which offers certain advantages over conventional QPCR. The whole-cell QPCR method does not require the DNA extraction prior to the PCR reaction and is simpler and easier to use compared to the traditional QPCR. It is also labor and time-efficient compared to microscopy and allows processing more samples over the same time period, while being suitable to measure particle concentrations that are too low to determine using microscopy (CitationAn et al. 2006). We think the method makes a good candidate to be included in the bioaerosol detection system shown in .
The EPSS has achieved very high concentration rates when sampling two test bacteria. However, for other particles, its performance may vary depending on the nature of particles, their hydrophobicity, and the interaction between particles and the collection surface. The effect of these parameters on the sampler's performance will be investigated in future projects.
We also compared the reproducibility of the collection efficiency data obtained by the two different methodologies (AOEM versus QPCR) by calculating the coefficient of variation (COV) for each sample. As could be seen from , the mean COV value () of QPCR data was 11% for both P. fluorescens and B. subtilis, indicating tightly grouped, precise data. However, the AOEM data sets had a
of 27% for P. fluorescens and 21% for B. subtilis indicating that the data were spread over a wider range of values. This is due to the inherently higher uncertainty in cell counting by microscopy. Overall, we believe that the whole-cell QPCR provides a promising alternative to the microscopic counting method in bioaerosol quantification. One potential drawback is the need for study- and microorganism-specific standard curves. However, once a pool of standard curves for different organisms and various sampling protocols is obtained, we believe the average value could be used to get a reasonably good estimate of the airborne microorganism concentration.
CONCLUSIONS
Experiments with the two common test microorganisms (Pseudomonas fluorescens and Bacillus subtilis) have shown that the novel bioaerosol sampler with superhydrophobic collection surface can efficiently collect and concentrate airborne bacteria in small amounts of liquid (5 or 40 μ L). For 10 min sampling, the collection efficiencies for both bacteria ranged from 48 to 72% and were substantially higher compared to the collection efficiencies for PSL particles of similar size. The difference was attributed to the different nature of particles—biological and non-biological—and their differences in accepting externally imparted electrical charge. When used with 5 μ L collection droplet and 10 L/min sampling flow rate, this new sampling concept allowed achieving very high bioaerosol sample concentration rates—more than 106/min. One potential drawback of the method was the decrease in the collection efficiency with longer sampling times. Even then, a 60 min sample concentrated in one small droplet would allow obtaining information about the presence of microorganisms at low concentrations. In the ambient environment, the bacteria are often carried by larger particles and the decrease in collection efficiency might be minimal or non-existent as was observed with PSL particles. Overall, we believe the data demonstrate the ability of the proposed sampling concept to detect low microorganism concentrations while having low power requirements due to the absence of pressure drop inside the EPSS.
It was also shown that the novel bioaerosol sampling technology is compatible with modern sample analysis techniques, such as whole-cell QPCR, which provides the total number of collected cells. This techniques could be incorporated into an advanced bioaerosol monitoring system suggested in and would thus allow to measure low bioaerosol concentrations in various environments. Integration of the sampling and analysis techniques and their testing in the field will be the next step of the project.
The publication was supported by the Grant R21-OH00656 “Design of Advanced Electrostatic Sampler for Total Bioaerosols” from CDC-NIOSH. Its contents are solely the responsibility of the authors and do not necessarily represent the official view of the CDC-NIOSH.
REFERENCES
- Adhikari , A. , Jung , J. , Reponen , T. , Lewis , J. S. , Degrasse , E. C. , Grimsley , L. F. , Chew , G. L. and Grinshpun , S. A. 2009 . Aerosolization of Fungi, (1 → 3)-β -d Glucan, and Endotoxin from Flood-Affected Materials Collected in New Orleans Homes. . Environ. Res. , 109 ( 3 ) : 215 – 224 .
- Amann , R. I. , Ludwig , W. and Schleifer , K. H. 1995 . Phylogenetic Identification and In Situ Detection of Individual Microbial Cells without Cultivation . Microbiol. Rev. , 59 ( 1 ) : 143 – 169 . 1995
- An , H. R. , Mainelis , G. and White , L. 2006 . Development and Calibration of Real-Time PCR for Quantification of Airborne Microorganisms in Air Samples. . Atmos. Environ. , 40 : 7924 – 7939 .
- An , H. R. , Mainelis , G. and White , L . 2009 . Quantitative Real-Time PCR for Bioaerosol Detection: Analysis of Factors Affecting Standard Curves . Environ. Sci. Technol. , submitted
- Burge , H. A. , Feeley , J. C. , Kreiss , K. , Milton , D. , Morey , P. R. , Otten , J. A. , Peterson , K. , Tulis , J. J. and Tyndall , R. 1989 . Guidelines for the Assessment of Bioaerosols in the Indoor Environment American Conference of Governmental Industrial Hygienists: Cincinnati
- Buttner , M. P. and Stetzenbach , L. D. 1991 . Evaluation of Four Aerobiological Sampling Methods for the Retrieval of Aerosolized Pseudomonas syringae . Appl. Environ. Microbiol. , 57 : 1268 – 1270 .
- Carlson , C. , DeGange , J. , Cable-Dunlap , P. and Halverson , J. . Aerosol-to-Liquid Particle Extraction System (ALPES) . Abstracts of 2nd Joint Conference on Point Detection for Chemical and Biological Defense . March 1–5 2004 , Williamsburg, VA. pp. 81 – 82 .
- Chiba , S. , Okada , S. , Suzuki , Y. , Watanuki , Z. , Mitsuishi , Y. , Igusa , R. , Sekii , T. and Uchiyama , B. 2009 . Cladosporium Species-Related Hypersensitivity Pneumonitis in Household Environment . Intern. Med. , 48 ( 5 ) : 363 – 367 .
- Dahlqvist , M. and Johard , U. 1992 . Lung Function and Precipitating Antibodies in Low Exposed Wood Trimmers in Sweden. . Am. J. Ind. Med. , 21 ( 4 ) : 549 – 559 .
- DeLong , E. F. and Pace , N. R. 2001 . Environmental Diversity of Bacteria and Archaea. . Syst. Biol. , 50 ( 4 ) : 470 – 478 .
- Eduard , W. and Heederik , D. 1998 . Methods for Quantitative Assessment of Airborne Levels of Noninfectious Microorganisms in Highly Contaminated Work Environments. . Am. Ind. Hyg. Assoc. J. , 59 ( 2 ) : 113 – 127 .
- Gorny , R. L. , Reponen , T. , Willeke , K. , Schmechel , D. , Robine , E. , Boissier , M. and Grinshpun , S. A. 2002 . Fungal Fragments as Indoor Air Biocontaminants. . Appl. Environ. Microbiol. , 68 : 3522 – 3531 .
- Han , T. and Mainelis , G . 2008 . Design and Development of an Electrostatic Sampler for Bioaerosols with High Concentration Rate . J. Aerosol Sci , 39 ( 12 ) : 1066 – 1078 .
- Hu , S. and McFarland , A. R. 2007 . Numerical Performance Simulation of a Wetted Wall Bioaerosol Sampling Cyclone . Aerosol Sci. Technol. , 41 ( 2 ) : 160 – 168 .
- Hill , S. C. , Pinnick , R. G. , Niles , S. , Pan , Y.-L. , Holler , S. and Chang , R. K. 1999 . Real-Time Measurement of Fluorescence Spectra from Single Airborne Biological Particles. . Field Anal. Chem. Technol. , 3 : 221 – 239 .
- Hinds , W. C. 1999 . Aerosol Technology, , 2nd Ed. , New York : Wiley Interscience .
- Hindson , B. J. , Makarewicz , A. J. , Setlur , U. S. , Henderer , B. D. , McBride , M. T. and Dzenitis , J. M. 2005 . APDS: the Autonomous Pathogen Detection System. . Biosensors and Bioelectronics. , 20 ( 10 ) : 1925 – 1931 .
- Johnson , B. D. , Martin , D. and Resnick , I. G. 1994 . Efficacy of Selected Respiratory Protective Equipment Challenged with Bacillus subtilis subsp. niger. . Appl. Environ. Microbiol. , 60 : 2184 – 2186 .
- Karol , M. H. 1991 . Allergic Reactions to Indoor Air Pollutants. . Environ. Health Perspect. , 95 : 45 – 51 .
- Lin , X. , Reponen , T. , Willeke , K. , Wang , Z. , Grinshpun , S. A. and Trunov , M. 2000 . Survival of Airborne Microorganisms During Swirling Aerosol Collection . Aerosol Sci. Technol. , 32 : 184 – 196 .
- Mainelis , G. , Masquelier , D. , Makarewicz , A. and Dzenitis , J. 2005 . Performance Characteristics of the Aerosol Collectors of the Autonomous Pathogen Detection System (APDS) . Aerosol Sci. Technol. , 39 ( 5 ) : 461 – 471 .
- Martinez , K. F. , Sheehy , J. W. , Jones , J. H. and Cusick , L. B. 1998 . Microbial Containment in Conventional Fermentation Processes. . Appl. Ind. Hyg. , 3 : 177 – 181 .
- Nadkarni , M. A. , Martin , F. E. , Jacques , N. A. and Hunter , N. 2002 . Determination of Bacterial Load by Real-Time PCR using a Broad-Range (Universal) Probe and Primers Set. . Microbiology. , 148 : 257 – 266 .
- Neidhardt , F. C. , Ingraham , J. L. and Schaechter , M. 1990 . Physiology of the Bacterial Cell: A Molecular Approach , 27 – 33 . Sunderland, MA : Sinauer Associates, Inc .
- Poulsen , O. M. , Breum , N. O. , Ebbehoj , N. , Hansen , A. M. and Ivens , U. I. 1995 . Collection of Domestic Waste. Review of Occupational Health Problems and Their Possible Causes. . Sci. Total Environ. , 170 : 1 – 19 .
- Robbins , C. A. , Swenson , L. J. , Nealley , M. L. and Gots , R. E. 2000 . Health Effects of Mycotoxins in Indoor Air: A Critical Review. . Appl. Occup. Environ. Hygiene. , 15 : 773 – 784 .
- Seo , Y. 2007 . Design of Wetted Wall Bioaerosol Concentration Cyclone , Ph.D. Dissertation College Station, TX : Dept. of Mech. Eng., Texas A&M University .
- Wang , Z. , Reponen , T. , Grinshpun , S. A. , Gorny , R. L. and Willeke , K. 2001 . Effect of Sampling Time and Air Humidity on the Bioefficiency of Filter Samplers for Bioaerosol Collection . J. Aerosol Sci , 32 ( 5 ) : 661 – 674 .