Abstract
Complementary single particle measurements of organic aerosols using aerosol time-of-flight mass spectrometry (ATOFMS) and Scanning Transmission X-ray Microscopy—Near Edge X-ray Absorption Fine Structure (STXM-NEXAFS) are compared to examine the relationships between particle morphologies and chemical composition of particles having similar sources. ATOFMS measurements provide size-resolved chemical composition information for single particles. Measurements from field campaigns in polluted or urban (Riverside/SOAR 2005; Mexico City/MILAGRO 2006; Port of Long Beach 2007) and clean or marine (Arabian Sea/INDOEX 1999; Sea of Japan/ACE-Asia 2001; Trinidad Head/CIFEX 2004) locations illustrate regional differences. The majority (≥ 85 %) of the number of submicron particles are carbonaceous (including elemental and organic carbon), but represent less than 10% of the number of supermicron particles. Organic carbon (OC) particles are classified into three meta-classes corresponding to (1) combustion generated OC/EC internal mixtures, (2) biomass burning generated K/OC mixtures, and (3) OC/High Mass OC (HMOC) mixtures containing secondary markers of atmospheric processing. Normalized dot products are used to quantify similarity among fragment spectra and indicate that OC particle types are consistent across (and within) platforms. Single particle carbon STXM-NEXAFS measurements during ACE-Asia 2001 and MILAGRO 2006 yield similar source categories based on relative abundances of aromatic, alkane, and carboxylic acid functional groups. All three organic particle types correspond to a variety of very heterogeneous particle morphologies, although the highly oxygenated OC particles with likely secondary organic contributions frequently are nearly spherical, liquid-like particles. Size-resolved number fractions of the major ATOFMS OC particle types show qualitative agreement with OC particle types from STXM-NEXAFS analysis, indicating a correspondence of the OC/EC type with the presence of strong aromatic groups, of the OC/HMOC type with high carboxylic acid groups, and of the biomass burning OC type with aromatic and carbonyl groups. ATOFMS measurements can be used to establish robust statistics for offline single particle techniques, providing the atmospheric context for the functional group and morphological information obtained from STXM-NEXAFS for an improved understanding of the climate impact of organic aerosols.
INTRODUCTION
Tropospheric aerosol particles vary in their composition and phase and play a significant role in human health and the climate system (CitationAndreae and Crutzen 1997; CitationPoschl 2005; CitationSeinfeld 2004). Aerosol particles can be either directly emitted into the atmosphere from combustion sources (fossil fuel and biomass burning) and wind-driven processes (resuspension of dust and creation of ocean-derived particles), or formed through the condensation of low volatility gas phase species produced photochemically, and through cloud processing (CitationPrather et al. 2008). Though the mechanisms of the aerosol direct (CitationJacobson 2001; CitationLiousse et al. 1996; CitationPenner et al. 1998) and indirect effects (CitationJones and Slingo 1997; CitationTwomey 1977) are qualitatively understood, there exists an uncertainty of as much as a factor of 2 in the radiative forcing magnitude (CitationChung and Seinfeld 2002). A major contributing factor to this large uncertainty is the limited knowledge of the specific chemical and morphological characteristics of atmospheric aerosols, which, in turn, limits our understanding of their size distributions, optical and hygroscopic properties, and residence time in the atmosphere (CitationPrather et al. 2008; CitationTurpin et al. 2000). Carbonaceous particles (elemental carbon, EC and organic carbon, OC) constitute between 20 and 80% of submicron aerosol mass (CitationJacobson et al. 2000; CitationTurpin and Lim 2001) with total anthropogenic emissions estimated at 42 Tg yr−1 (CitationBond et al. 2004). The absorbing fraction of carbonaceous aerosols has been estimated as the second largest contributor to global warming (CitationJacobson et al. 2000; CitationRamanathan and Carmichael 2008); however, the light absorbing properties are strongly dependent on the mixing state of these particles (CitationBond and Bergstrom 2006; CitationSchnaiter et al. 2005). Therefore an understanding and chemical characterization of individual aerosol particles is essential to evaluate their impact on the climate.
Aerosol time-of-flight mass spectrometry (ATOFMS) with laser desorption/ionization (LDI) has proven to be a valuable analytical tool for online size-resolved chemical characterization of ambient aerosol samples (CitationSullivan and Prather 2005; CitationZimmermann et al. 2003). Using this technique, the classification of several different particle types is possible through knowledge of their chemical fingerprints, and questions of mixing states between dust and crustal species, elemental carbon, as well as organic species that comprise the bulk of ambient aerosols can be addressed (CitationGross et al. 2000a; CitationGross et al. 2000b; CitationSilva and Prather 2000; CitationSuess and Prather 1999). In ATOFMS, organic and inorganic aerosol material is desorbed and ionized from single particles by an intense laser pulse (laser desorption/ionization process, LDI) and the positive and negative ions are subsequently analyzed by a dual polarity time-of-flight mass spectrometer. The positive mass spectrum shows cations such as metal ions, ammonium, and carbon-containing clusters while the negative spectrum contains carbon-containing clusters as well as additional peaks from secondary anions like sulfate, phosphate, nitrate, and certain organic species. In addition to chemical heterogeneity, aerosol particles also exhibit varied physical configurations (including spheres, agglomerations, inclusions, and shells) that have implications for their optical properties (CitationMishchenko et al. 2004; Citation2007) and hygroscopicity (CitationCruz and Pandis 1998; CitationSjogren et al. 2007). Scanning Transmission X-Ray Microscopy with Near-Edge X-ray Absorption Fine Structure (STXM-NEXAFS) is a highly sensitive chemical analysis technique that can be used to non-destructively probe particle morphology and functional group composition in individual particles relevant to the atmosphere (CitationKilcoyne et al. 2003; CitationRussell et al. 2002; CitationTakahama et al. 2007; CitationTakahama et al. 2008). STXM-NEXAFS provides advantages in higher chemical resolution for organic components compared to electron microscopy—providing functional group composition at a scale of 30 nm, in addition to its capability for analysis at atmospheric pressure and the lower likelihood of radiation damage. However, the number of particles that can be analyzed is limited by access to a synchrotron radiation facility with a scanning x-ray microscopy end-station.
This article presents a comparison of the chemical characteristics of the organic aerosol fraction measured using ATOFMS from six field campaigns carried out between 1999 and 2007 in different geographic regions: INDOEX (Indian Ocean, March 1999), ACE-Asia (Sea of Japan, March–April 2001), CIFEX (Trinidad Head, CA, April 2004), SOAR-I and SOAR-II (Riverside, CA, 2005), MILAGRO (Mexico City, March 2006), and in Long Beach, CA (August 2007). The dates, coordinates, and sampling environment for each project are summarized in . Nearby STXM-NEXAFS measurements from ACE-Asia and MILAGRO are compared to the ATOFMS measurements to examine the relationships between particle morphologies and chemical compositions having similar source origins to improve our understanding of organic aerosols further. The purpose of this study is to establish statistically robust trends in size, composition, and mixing states of organic aerosols essential for quantifying their climate impact and to link these trends to detailed particle morphology descriptions. Though not exhaustive, the subset of measurements considered here provides an essential first step in the preparation of a complete global climatology of single particle measurements from contrasting regions.
TABLE 1 Summary of representative ATOFMS and STXM-NEXAFS field measurements used in this analysis. Column 6 shows the number (and fraction of total) of submicron organic carbon particles measured at each platform and their distribution among particle classes. OC/EC mix, K/Biomass, and OC/HMOC for ATOFMS and Combustion, Biomass burning, and secondary fractions for STXM-NEXAFS are illustrated counter-clockwise
EXPERIMENTAL METHODS
The aerosol time-of-flight mass spectrometer (ATOFMS) as described by Gard et al. (1997) was used to determine the size and chemical composition of individual aerosol particles during the selected field campaigns. Ambient aerosol samples were drawn into a vacuum chamber through a nozzle inlet (with size cut-offs at 0.2 and 3 μ m), resulting in a supersonic expansion that accelerates individual particles to terminal velocities. The aerodynamic diameter of each particle is determined by measuring the time-of-flight between two continuous scattering Nd:YAG lasers operating at 532 nm. The firing of a third pulsed Nd:YAG laser (266 nm) is triggered based on the particle velocity, resulting in the ablation and ionization of the particle in the center of the mass spectrometer. Positive and negative ions produced from each particle are separately transferred to two flight tubes, and spectra are measured simultaneously using time-of-flight mass spectrometry. Recent versions of the ATOFMS's data acquisition systems permit the analysis of up to a few hundred individual particles per minute (CitationPratt et al. 2009). Project specific details for the selected field studies used in this work are summarized in . ATOFMS measurements were analyzed using the Matlab-based toolkit YAADA (www.yaada.org). To determine chemical characteristics, a subset of particles were classified using the ART-2a clustering algorithm (CitationSong et al. 1999) with resulting clusters matched to the entire dataset. The subset typically represented between 2.5 and 5% of all collected particles. Between 50 and 60 of the largest clusters represent in excess of 90% of all particles, with the remainder comprising a large number of sparsely populated clusters. These clusters were manually grouped into a smaller number based on the major ion peaks in their mass spectra (CitationGuazzotti et al. 2003; CitationMoffet et al. 2008a; CitationSu et al. 2005).
Particles for STXM-NEXAFS analysis were collected on silicon nitride windows (Si3N4; Silson Ltd.) mounted on a rotating impactor (Streaker; PIXE International, Inc.) by drawing air into the impactor at 1 liter per minute (lpm). Sample windows were analyzed at the Advanced Light Source at Lawrence Berkeley National Laboratories (Berkeley, CA) in a He-filled chamber maintained at 1 atm. Photon transmission was measured at energy levels between 278 and 305 eV and converted to optical density as described in earlier work (CitationMaria et al. 2004; CitationRussell et al. 2002; CitationTakahama et al. 2007). Spectra were adjusted for background absorbance (278 eV–283 eV), normalized using the total carbon content (CitationMaria et al. 2004), and subsequently classified using the Ward hierarchical clustering algorithm (CitationWard 1963). These clusters were manually condensed into a smaller number representing similarities in atmospheric particles based on qualitative information such as sampling times and conditions, chemical similarity between spectra, and location (CitationTakahama et al. 2007).
ATOFMS Particle Types
Single particle clusters containing carbonaceous particles were identified by the presence of carbon cluster fragments (Cn, with n typically between 1 and 3, but as large as 12 in some cases) in both the positive and negative ion spectra. Particles with spectra lacking these markers can originate from dust, combustion-generated metallic particles, or sea salt; but their spectra are omitted from this study. It is important to note that the presence of water suppresses negative ion formation (CitationNeubauer et al. 1998) occasionally resulting in particles that do not produce a negative spectrum (CitationAult et al. 2009; CitationMoffet et al. 2008b); these particles were excluded from this analysis. Carbonaceous particles that were dominated by strong Cn fragments with mass to charge ratio (m/z) 12, 24, and 36, and lacking tracer fragments associated with organic carbon: (C2H3: +27, C3H3: +39, and C2H3O: +43) were classified as elemental carbon (EC), with the remainder constituting organic carbon (OC) particles (CitationMoffet et al. 2008a; CitationSodeman et al. 2005; CitationSu et al. 2005). The presence (or absence) of alkali metal ions (such as Na+, K+, or Ca+) and secondary tracers (such as Cl−, NO2 −, NO3 −, HSO4 −, or SO4 −) was not considered in this preliminary classification, and these species may be internally mixed with either the non-carbonaceous, EC, or OC particles. The number fractions of OC particles for the selected field campaigns are summarized in .
Carbon-containing particles dominate the submicron size fraction, contributing between 80% (INDOEX 1999) and 95% (Long Beach 2007) by number. Non-carbonaceous particles constitute the majority of supermicron particles in each data set with number fractions ranging from 80% in polluted urban environments (SOAR 2005 and MILAGRO 2006) up to 98% in a clean marine environment (ACE-Asia). Sea salt particles constituted the majority of both size fractions during ACE-Asia (CitationSullivan and Prather 2007), but the submicron number fraction of carbonaceous particles was significantly higher (varying between 20% and 40% depending on the air mass) compared to less than 10% for the supermicron particles, consistent with the size partitioning of carbon-containing particles observed at the other sites. The submicron EC number fraction ranges between 5% (INDOEX 1999) and 23% (SOAR II 1905) and is typically smaller than the OC number fraction in both size fractions for the field campaigns in this study.
The organic carbon particles were further resolved into three meta-classes that were observed in different proportions during each of the field campaigns. The average positive ion mass spectra for all particles within these three meta-classes are illustrated in , along with the standard deviation as a measure of the variability within each class. The differences in mass spectra correspond to chemical differences between the particles that can be related qualitatively to their sources to the atmosphere.
FIG. 1 Average normalized positive ion spectra for OC meta classes measured using the ATOFMS corresponding to (a) EC/OC mixture, (b) K/OC biomass burning, and (c) Processed/OC type of particles. The dashed lines indicate the standard deviation from the average spectra within each particle class. Negative ion spectra are not illustrated.
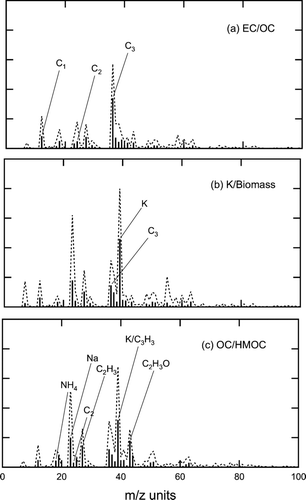
EC/OC Mixture
The Elemental Carbon/Organic Carbon (ECOC) type is characterized by a positive ion mass spectrum that is dominated by carbon atom clusters (Cn) with smaller signal contributions from OC, nitrate, sulfate, and ammonium tracer fragments. The similarities of these mass spectra to test spectra for vehicular exhaust (CitationSodeman et al. 2005) and elemental carbon suggest that these particles are produced from primary sources of fossil fuel combustion, having undergone addition of secondary condensates by atmospheric processing which is small relative to the EC amount.
K/OC Mixture
These particles have a large peak at +39 that is attributed to K+ in the positive ion spectra, and additionally may contain negative ion fragments at −45, −59, and −73 that have been associated with levoglucosan fragments. These tracers are typically associated with combustion products specific to biomass burning or biofuel combustion (CitationBein et al. 2008; CitationGuazzotti et al. 2003; CitationHudson et al. 2004; CitationSilva et al. 1999; CitationSilva and Prather 2000) and indicate that this meta-class contains particles produced from the burning of plant matter.
OC/High Mass OC Mixture
These particles are characterized by hydrocarbon ion series starting with the base carbon peak at +12 but also containing major OC peaks at +27 (C2H3) and +43 (C2H3O), with the latter oxygenated fragment typical of atmospheric oxidation (CitationSilva and Prather 2000). Many of these particles also contain peaks at +39 (K or C3H3) suggesting possible condensation of secondary organic species on primary aerosol cores. A smaller fraction of these particles contain positive ion fragments up to and beyond +150 indicative of high mass compounds that may be formed via aqueous phase processing during long range transport (CitationPratt and Prather 2009).
To summarize, particles are identified as OC by the presence of peaks at +12, +24, +36, and +27; the subset of these particles with a peak at +43 are classified as OC/HMOC; the subset of remaining particles with a peak at +39 are classified as K/Biomass; and the remainder are classified as EC/OC mixtures. The existence of the same OC meta-classes at each of the six measurement sites allows for a cross-platform comparison of OC particle spectra to address the similarity between organic particles derived from similar sources in polluted urban environments and clean marine environments. A simple quantitative metric analogous to the vigilance factor used to classify the particle spectra is the normalized Euclidean distance between ion fragment spectra within each cluster. If the spectra within each cluster are expressed as vectors then a similarity number α can be defined as
FIG. 2 The similarity number defined by Equation (Equation1) calculated for ATOFMS OC meta-classes separately for six different field campaigns, and for a grand average of all particles. Empty markers (left column in each set) show values calculated using entire spectrum, Solid markers (right in column in each set) show values calculated from positive ions only. Boxes are used to show approximate range of values.
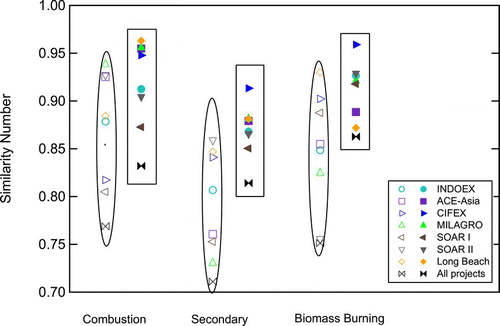
illustrates the size-resolved distribution of OC meta-classes for PM2.5 particles for each field project, with the relative fractions normalized by the total number of OC type particles. A few general trends emerge based on the synthesis of these field measurements: (1) the OC/EC mixture class constitutes the largest segment of OC particles at small sizes and decreases with increasing aerodynamic diameters (following the same trend as EC particles); (2) OC/EC mixtures constitute a larger fraction of OC particles in polluted urban environments (e.g., Mexico City and Riverside); (3) the OC/High Mass OC mixture class constitutes a larger fraction of OC particles at larger diameters and decreases at smaller sizes; (4) the OC/High mass OC mixtures contribute to the majority of particles in cleaner environments (INDOEX and CIFEX—consistent with the formation of larger particles from cloud and fog processing during transport); and (5) the K/Biomass mixtures typically make up less than 20% of all OC particles, with the exception being in Mexico City where this class contributes in excess of 40% of OC particles—this local variation has been attributed to forest fires and emissions from local trash burning being significant sources of organic compounds during MILAGRO (CitationDeCarlo et al. 2008; CitationMoffet et al. 2008a; CitationYokelson et al. 2007). The relatively higher number contribution of the oxygenated meta-classes (biomass burning and OC/HMOC mixtures) in clean marine environments compared to polluted urban environments is consistent with comparative studies using Aerosol Mass Spectrometry (AMS) measurements (CitationZhang et al. 2007) that find a higher fraction of oxygenated organics in remote environments than in polluted environments. The larger contribution of the EC/OC class compared to the OC/HMOC in the field studies considered here is consistent with a smaller contribution of secondary aerosol to the total organic mass during the spring months compared to the late summer and fall (CitationShields et al. 2008; CitationZhang et al. 2007).
FIG. 3 Size-resolved normalized number fraction of organic particles measured using the ATOFMS during (a) INDOEX 1999 (spring), (b) CIFEX 2004 (spring), (c) ACE-Asia 2001 (spring; polluted marine region), (d) SOAR-II 2005 (fall), (e) MILAGRO 2006 (spring), and (f) Long Beach 2007 (fall). Bar segments from bottom to top illustrate of EC/OC, K/Biomass, and OC/HMOC fractions.
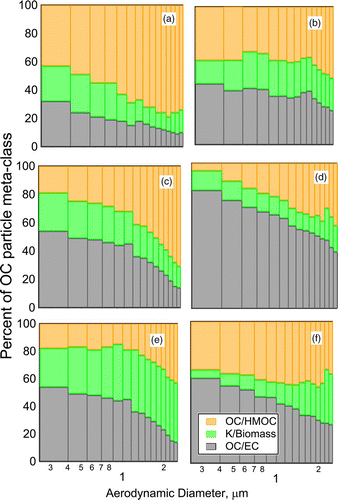
STXM-NEXAFS Particle Types
Spectra from STXM-NEXAFS were determined by averaging rasterized scans over each particle and classified using a clustering algorithm following subtraction of the pre-carbon edge absorbance and normalization by the mean (CitationTakahama et al. 2007; CitationTakahama et al. 2010). In addition to spectral classification, the functional group composition of the carbonaceous fraction was determined from the relative absorption measured by fitting Gaussian peaks to the particle spectra (CitationBraun 2005; CitationRussell et al. 2002; CitationTivanski et al. 2007). Based on a training set of reference spectra, CitationTakahama et al. (2007) categorized carbonaceous particles into four source related meta-classes, with a small number labeled “other.” The “ultisol” meta-class shows similarities to soil particles collected in the Caribbean (CitationAde and Urquhart 2002) and is considered a subset of dust particles. The three remaining meta-classes constitute OC particles as defined within this study. The normalized absorption spectra for the OC particle classes are illustrated in .
FIG. 4 Average STXM-NEXAFS absorption spectra scaled by maximum absorbance spectra for (a) Combustion generated, (b) Biomass burning, and (c) Secondary particles. Average spectra for ACE-Asia 2001, MILAGRO 2006, and both projects combined are illustrated using dark, light, and black lines. Dotted vertical lines show spectra features corresponding to R(C = C)R at 284 and 285 eV, R(C = O)R at 286.7 eV, R(C–H)R at 287.7 eV, R(C = O)OH at 288.7 eV, RNH-ROH at 289.5 eV, and K+ at 297.4 and 299.9 eV.
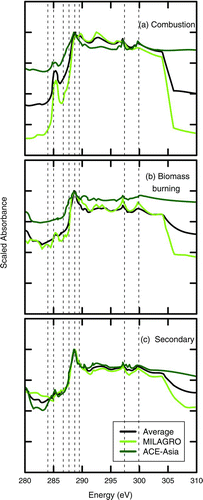
Combustion Particles
The first meta-class of OC particles shows significant absorbance at 284 and 285 eV, which is attributed to the R(C═C)R aromatic/alkene bond, indicating the presence of sp2 hybridized carbon associated with soot and black carbon (CitationAndreae and Gelencser 2006; CitationMaria et al. 2004; CitationTakahama et al. 2007). In addition to the strong aromatic absorbance, particles in this class also showed peaks at 286.7 and 287.7 eV, attributed to R(C═O)R carbonyl and R(C-H)R alkane groups, respectively, with a smaller relative abundance.
Biomass Burning Particles
Particle spectra in this class showed multiple transitions with absorption peaks corresponding to R(C═C)R, R(C═O)R, and R(C═O)OH bonds (288.7 eV), although the peaks were weaker than those identified for the soot containing particles (CitationTakahama et al. 2007). These particle types show spectral features similar to reported observations for phenols, biogenic fulvic acids, and the products of wood burning (CitationAde and Urquhart 2002; CitationBraun 2005; CitationTivanski et al. 2007), and are therefore attributed to emissions from biomass burning.
Highly Oxygenated or Secondary Particles
The third class of OC particles shows the highest relative abundance of absorbance due to a R(C═O)OH carboxylic acid signature, which is typically associated with the gas phase oxidation of volatile organic compounds that partition into the aerosol phase. Particles dominated by this signal are associated with either secondary organic aerosol or primary aerosol particles that have undergone significant atmospheric processing and aging.
Since STXM-NEXAFS allows non-destructive scanning, the morphology and heterogeneity of carbon distribution within single particles can be measured via image analysis. illustrates example single particle images for the three types of OC particles observed in Mexico City during MILAGRO 2006. A complete description of particle morphology analysis and results based on particles collected during six field experiments has been prepared by CitationTakahama et al. (2010). Based on this more extensive work, a few general trends relevant to the particles considered here can be established—(1) OC particle types show varied morphology (spherical, elongated, fractal aggregates) for all meta classes; (2) combustion and biomass burning type particles are typically heterogeneous, containing inclusions and layers; (3) secondary particles are often spherical and homogenous; and (4) the small number of shell-core type particles observed were all secondary.
FIG. 5 STXM-NEXAFS generated images of particles collected during ACE-Asia 2001 and MILAGRO 2006. Darker pixels indicate higher scaled carbon absorption relative to the Si3N4 collection windows. Example images illustrate (a–c) combustion particles, (d–f) biomass burning particles, and (g–i) secondary particles.
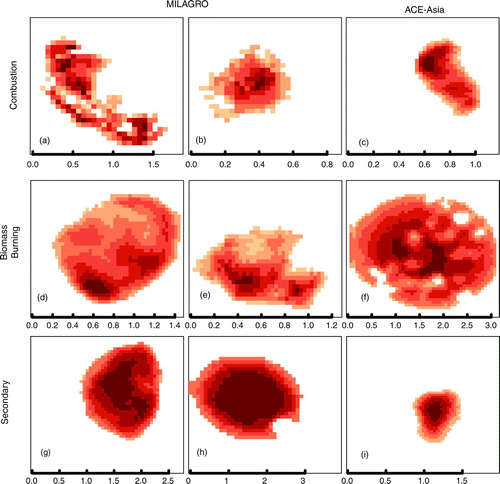
Comparison of ATOFMS and STXM-NEXAFS
The analysis of ATOFMS and STXM measurements at multiple sites indicates that while the specific chemical composition of organic aerosols is complex and varying, this system can be simplified by its representation by a relatively small set of representative meta-classes. Both techniques yield three predominant types of organic particles associated with similar source processes, suggesting that a relationship exists between particles analyzed by these two complementary techniques. Based on their respective classification criteria, the particle classes can be related to each other as: EC/OC mixtures from ATOFMS to Combustion from STXM, K/Biomass mixtures to Biomass burning, and OC/HMOC mixtures to Highly Oxygenated (or Secondary) particle types, as summarized in .
TABLE 2 Positive ion fragments (ATOFMS) and organic functional groups (STXM-NEXAFS) used to identify corresponding organic aerosol particle types
shows a size-resolved comparison of the number fraction of the three OC particle types collected during the ACE-Asia and MILAGRO field campaigns at nearly co-located platforms. The same qualitative trends are observed at both platforms, i.e., combustion and EC/OC type particles contribute a larger number fraction at smaller sizes, secondary and OC/HMOC type particles contribute a larger fraction at the larger end of submicron sizes; and biomass burning type particles constitute a much larger number fraction of organic particles during the MILAGRO study. Two significant caveats must be considered when conducting this comparison, which contribute to the lack of a close quantitative agreement between the methods. First, the ATOFMS sizes particles based on aerodynamic diameter, while STXM particle sizes are geometric measures of the impacted size using image contrasts with the substrate. Second, the high sampling rate of the ATOFMS results in a robust statistical distribution—a total of 14,552 and 126,611 OC particles were sampled during ACE-Asia and MILAGRO, respectively, whereas the 101 and 81 particles analyzed by STXM provide only an approximate characterization of the relative abundance of different types.
FIG. 6 Size resolved number fraction of organic particles collected during (a) and (b) ACE-Asia 2001 and (c) and (d) MILAGRO 2006. Bar segments from bottom to top in panels (a) and (c) show OC/EC, K/Biomass, and OC/HMOC particles as classified by the ATOFMS, and in panels (b) and (d) show combustion, biomass burning, and secondary particles classified from STXM-NEXAFS spectra.
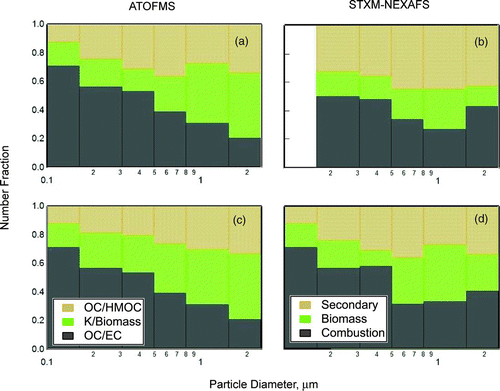
The existence of a qualitative agreement between OC particle types from both methods and their attribution to similar sources can be used as justification for relating the functional groups measured by STXM-NEXAFS to the tracer ion fragments from ATOFMS. For the combustion type particles, the R(C═C)R and R(C─H)R bonds can be linked to the formation of Cn and C2H3 fragments, and the R(C═O)OH group in secondary particles can be linked to the formation of the C2H3O oxygenated fragment. These relationships between chemical bonds and bond fragments are consistent with those observed from Fourier Transform Infrared Spectroscopy (FTIR) and Aerosol Mass Spectrometry (AMS) (CitationRussell et al. 2009). This establishment of analogous particle types can improve our understanding of organic aerosols by combining the specific advantages of each method—ATOFMS measurements can be used to establish robust statistics for size resolved number fractions, which can be related to functional group and morphological information obtained from STXM-NEXAFS.
CONCLUSIONS AND FUTURE DIRECTIONS
Consistent trends in organic aerosol composition and morphology are shown from this synthesis of measurements from six field campaigns representing both polluted and clean environments. Most carbonaceous aerosol particles (OC and EC) particles exist in the submicron size fraction, contributing between 20% (ACE-Asia, marine environment) and 85% (MILAGRO/SOAR, polluted urban environments) of all particles. OC particles can be consistently represented by three source related meta-classes for ATOFMS and STXM-NEXAFS analysis: combustion (fossil fuel burning), biomass burning, and secondary aerosols (SOA—that may contain primary aerosol cores). Secondary aerosol particles contribute a significant number fraction to the total organic aerosol, ranging between 20% (MILAGRO/SOAR, polluted urban environments) and 50% (INDOEX, clean marine environment). The ATOFMS submicron mode in MILAGRO 2006 comprises 37% OC/EC, 30% OC/HMOC, and 33% biomass burning particles and the submicron mode in ACE-ASIA 2001 comprises 50% OC/EC, 17% OC/HMOC, and 33% biomass burning particles.
Single particle carbon spectra obtained from nearby Scanning Transmission X-Ray Microscopy-Near Edge X-ray Absorption Fine Structure (STXM-NEXAFS) measurements from ACE-ASIA 2001 and MILAGRO 2006 yield similar source categories based on relative abundances of aromatic, alkane, and carboxylic acid functional groups. The similarities in OC particle types observed using both techniques provides a basis for combining the quantitative information on particle number distribution and characteristic EC and OC fragments of ATOFMS with the functional group, morphology, and heterogeneity characteristics observed by STXM-NEXAFS. ATOFMS OC particle types and STXM-NEXAFS particle categories show a correspondence of the OC/EC type with strong aromatic group absorbance, of the OC/HMOC mixture type with high carboxylic acid group absorbance, and of the biomass burning OC type with aromatic and carbonyl groups. All three organic particle types correspond to a range of particle morphologies some of which contain heterogeneous inclusions, although the highly oxygenated or secondary OC type frequently are nearly spherical, liquid-like particles, sometimes with enhanced carboxylic acid groups near the surface.
The complete climate impact of organic aerosols can only be evaluated through the knowledge of the global distribution of OC resolved in time, space, particle size, mixing state, as well as water uptake and optical properties, which at present can only be estimated from global models. The uncertainty in model predictions is increased due to limitations in parameterizing organic aerosols—for example, most models appear to over-emphasize primary emissions and lack an explicit representation of secondary aerosols (CitationKanakidou et al. 2005; CitationTextor et al. 2006), though more recent work has improved the sophistication of climate models by explicitly including contributions from SOA (CitationBeekmann et al. 2007; CitationMatsui et al. 2009). Comparative studies of single particle measurements constitute a valuable resource by providing not only general trends in aerosol composition in various environments, but more complete information about the mixing states and morphology of the aerosol. Information about the relative contributions of different aerosol constituents, as well as their distribution both within and among individual particles, provides a greater understanding of atmospheric aerosols that can be used to better constrain model representations of organic particles and their climate impact.
Acknowledgments
This comparative study was funded by a grant from BP. Additional support from the National Science Foundation, the James S. McDonnell Foundation, and the California Air Resource Board funded our participation in the field studies used in this work. The authors would like to acknowledge all the members of the Russell and Prather research groups at UCSD responsible for collecting the measurements summarized here, including Satoshi Takahama, Ryan Moffett, Sergio Guazzotti, Sharon Quin, Keith Coffee, John Holecek, Matt Spencer, Andy Ault, Cassandra Gaston, David Sodeman, and Monica Rivera, as well as collaborators Patricia Quinn and Tim Bates of NOAA PMEL.
REFERENCES
- Ade , H. and Urquhart , S. G. 2002 . Chemical Applications of Synchotron Radiation , Singapore : World Scientific Publishing .
- Andreae , M. O. and Crutzen , P. J. 1997 . Atmospheric Aerosols: Biogeochemical Sources and Role in Atmospheric Chemistry . Science. , 276 : 1052 – 1058 .
- Andreae , M. O. and Gelencser , A. 2006 . Black Carbon or Brown Carbon? The Nature of Light-Absorbing Carbonaceous Aerosols . Atmos. Chem. Phys. , 6 : 3131 – 3148 .
- Ault , A. P. , Moore , M. J. , Furutani , H. and Prather , K. A. 2009 . Impact of Emissions from the Los Angeles Port Region on San Diego Air Quality During Regional Transport Events . Environ. Sci. Technol. , 43 : 3500 – 3506 .
- Beekmann , M. , Kerschbaumer , A. , Reimer , E. , Stern , R. and Moller , D. 2007 . PM Measurement Campaign HOVERT in the Greater Berlin Area: Model Evaluation with Chemically Specified Particulate Matter Observations for a One Year Period . Atmos. Chem. Phys. , 7 : 55 – 68 .
- Bein , K. J. , Zhao , Y. J. , Johnston , M. V. and Wexler , A. S. 2008 . Interactions Between Boreal Wildfire and Urban Emissions . J. Geophys. Res.—Atmos. , 113 DOI: 10.1029/2007JD008910
- Bond , T. C. , Streets , D. G. , Yarber , K. F. , Nelson , S. M. , Woo , J. H. and Klimont , Z. 2004 . A Technology-Based Global Inventory of Black and Organic Carbon Emissions from Combustion . J. Geophys. Res.—Atmos. , 109 DOI: 10.1029/2003JD003697
- Bond , T. C. and Bergstrom , R. W. 2006 . Light Absorption by Carbonaceous Particles: An Investigative Review . Aerosol. Sci. Technol. , 40 : 27 – 67 .
- Braun , A. 2005 . Carbon Speciation in Airborne Particulate Matter with C (1s) NEXAFS Spectroscopy . J. Environ. Monitor. , 7 : 1059 – 1065 .
- Chung , S. H. and Seinfeld , J. H. 2002 . Global Distribution and Climate Forcing of Carbonaceous Aerosols . J. Geophys. Res.—Atmos. , 107 DOI: 10.1029/2001JD001397
- Cruz , C. N. and Pandis , S. N. 1998 . The Effect of Organic Coatings on the Cloud Condensation Nuclei Activation of Inorganic Atmospheric Aerosol . J. Geophys. Res.—Atmos. , 103 : 13111 – 13123 .
- DeCarlo , P. F. , Dunlea , E. J. , Kimmel , J. R. , Aiken , A. C. , Sueper , D. , Crounse , J. , Wennberg , P. O. , Emmons , L. , Shinozuka , Y. , Clarke , A. , Zhou , J. , Tomlinson , J. , Collins , D. R. , Knapp , D. , Weinheimer , A. J. , Montzka , D. D. , Campos , T. and Jimenez , J. L. 2008 . Fast Airborne Aerosol Size and Chemistry Measurements Above Mexico City and Central Mexico During the MILAGRO Campaign . Atmos. Chem. Phys. , 8 : 4027 – 4048 .
- Gross , D. S. , Galli , M. E. , Silva , P. J. and Prather , K. A. 2000a . Relative Sensitivity Factors for Alkali Metal and Ammonium Cations in Single Particle Aerosol Time-of-flight Mass Spectra . Anal. Chem. , 72 : 416 – 422 .
- Gross , D. S. , Galli , M. E. , Silva , P. J. , Wood , S. H. , Liu , D. Y. and Prather , K. A. 2000b . Single Particle Characterization of Automobile and Diesel Truck Emissions in the Caldecott Tunnel . Aerosol Sci. Technol. , 32 : 152 – 163 .
- Guazzotti , S. A. , Suess , D. T. , Coffee , K. R. , Quinn , P. K. , Bates , T. S. , Wisthaler , A. , Hansel , A. , Ball , W. P. , Dickerson , R. R. , Neususs , C. , Crutzen , P. J. and Prather , K. A. 2003 . Characterization of Carbonaceous Aerosols Outflow from India and Arabia: Biomass / Biofuel Burning and Fossil Fuel Combustion . J. Geophys. Res.—Atmos. , 108 DOI: 10.1029/2002JD003277
- Holecek , J. C. , Spencer , M. T. , Furutani , H. , Ramanathan , V. and Prather , K. A. 2005 . Single Particle Chemical Analysis of Aerosols Generated from Rain Water Collected at Trinidad Head, CA During the Cloud Indirect Effects Experiment (CIFEX) . Abstr. Pap. Am. Chem. S , 229 : U127 – U127 .
- Hudson , P. K. , Murphy , D. M. , Cziczo , D. J. , Thomson , D. S. , de Gouw , J. A. , Warneke , C. , Holloway , J. , Jost , J. R. and Hubler , G. 2004 . Biomass-Burning Particle Measurements: Characteristic Composition and Chemical Processing . J. Geophys. Res.—Atmos , 109 DOI: 10.1029/2003JD004398
- Jacobson , M. C. , Hansson , H. C. , Noone , K. J. and Charlson , R. J. 2000 . Organic Atmospheric Aerosols: Review and State of the Science . Rev. Geophys. , 38 : 267 – 294 .
- Jacobson , M. Z. 2001 . Global Direct Radiative Forcing due to Multicomponent Anthropogenic and Natural Aerosols . J Geophys. Res.—Atmos. , 106 : 1551 – 1568 .
- Jones , A. and Slingo , A. 1997 . Climate Model Studies of Sulphate Aerosols and Clouds . Philos. T. Roy. Soc. B. , 352 : 221 – 228 .
- Kanakidou , M. , Seinfeld , J. H. , Pandis , S. N. , Barnes , I. , Dentener , F. J. , Facchini , M. C. , Van Dingenen , R. , Ervens , B. , Nenes , A. , Nielsen , C. J. , Swietlicki , E. , Putaud , J. P. , Balkanski , Y. , Fuzzi , S. , Horth , J. , Moortgat , G. K. , Winterhalter , R. , Myhre , C. E. L. , Tsigaridis , K. , Vignati , E. , Stephanou , E. G. and Wilson , J. 2005 . Organic Aerosol and Global Climate Modelling: A Review . Atmos. Chem. Phys. , 5 : 1053 – 1123 .
- Kilcoyne , A. L. D. , Tyliszczak , T. , Steele , W. F. , Fakra , S. , Hitchcock , P. , Franck , K. , Anderson , E. , Harteneck , B. , Rightor , E. G. , Mitchell , G. E. , Hitchcock , A. P. , Yang , L. , Warwick , T. and Ade , H. 2003 . Interferometer—Controlled Scanning Transmission X-Ray Microscopes at the Advanced Light Source . J. Synchrotron. Radiat. , 10 : 125 – 136 .
- Liousse , C. , Penner , J. E. , Chuang , C. , Walton , J. J. , Eddleman , H. and Cachier , H. 1996 . A Global Three-Dimensional Model Study of Carbonaceous Aerosols . J. Geophys. Res.—Atmos. , 101 : 19411 – 19432 .
- Liu , S. , Takahama , S. , Russell , L. M. , Gilardoni , S. and Baumgardner , D. 2009 . Oxygenated Organic Functional Groups and Their Sources in Single and Submicron Organic Particles in MILAGRO 2006 Campaign . Atmos. Chem. Phys. , 9 : 6849 – 6863 .
- Maria , S. F. , Russell , L. M. , Gilles , M. K. and Myneni , S. C. B. 2004 . Organic Aerosol Growth Mechanisms and Their Climate-Forcing Implications . Science , 306 : 1921 – 1924 .
- Matsui , H. , Koike , M. , Takegawa , N. , Kondo , Y. , Griffin , R. J. , Miyazaki , Y. , Yokouchi , Y. and Ohara , T. 2009 . Secondary Organic Aerosol Formation in Urban Air: Temporal Variations and Possible Contributions from Unidentified Hydrocarbons . J. Geophys. Res.—Atmos. , 114 DOI: 10.1029/2008JD010164
- Mishchenko , M. I. , Videen , G. , Babenko , V. A. , Khlebtsov , N. G. and Wriedt , T. 2004 . T-Matrix Theory of Electromagnetic Scattering by Particles and its Applications: A Comprehensive Reference Database . J. Quant. Spectrosc. Ra. , 88 : 357 – 406 .
- Mishchenko , M. I. , Videen , G. , Babenko , V. A. , Khlebtsov , N. G. and Wriedt , T. 2007 . Comprehensive T-Matrix Reference Database: A 2004–06 Update . J. Quant. Spectrosc. Ra. , 106 : 304 – 324 .
- Moffet , R. C. , de Foy , B. , Molina , L. T. , Molina , M. J. and Prather , K. A. 2008a . Measurement of Ambient Aerosols in Northern Mexico City by Single Particle Mass Spectrometry . Atmos. Chem. Phys. , 8 : 4499 – 4516 .
- Moffet , R. C. , Qin , X. Y. , Rebotier , T. , Furutani , H. and Prather , K. A. 2008b . Chemically Segregated Optical and Microphysical Properties of Ambient Aerosols Measured in a Single-Particle Mass Spectrometer . J. Geophys. Res.—Atmos. , 113 DOI: 10.1029/2007JD009393
- Neubauer , K. R. , Johnston , M. V. and Wexler , A. S. 1998 . Humidity Effects on the Mass Spectra of Single Aerosol Particles . Atmos. Environ. , 32 : 2521 – 2529 .
- Penner , J. E. , Chuang , C. C. and Grant , K. 1998 . Climate Forcing by Carbonaceous and Sulfate Aerosols . Clim. Dynam. , 14 : 839 – 851 .
- Poschl , U. 2005 . Atmospheric Aerosols: Composition, Transformation, Climate and Health Effects . Angew. Chem. Int. Edit. , 44 : 7520 – 7540 .
- Prather , K. A. , Hatch , C. D. and Grassian , V. H. 2008 . Analysis of Atmospheric Aerosols . Annu. Rev. Anal. Chem. , 1 : 485 – 514 .
- Pratt , K. A. , Mayer , J. E. , Holecek , J. C. , Moffet , R. C. , Sanchez , R. O. , Rebotier , T. P. , Furutani , H. , Gonin , M. , Fuhrer , K. , Su , Y. X. , Guazzotti , S. and Prather , K. A. 2009 . Development and Characterization of an Aircraft Aerosol Time-of-Flight Mass Spectrometer . Anal. Chem. , 81 : 1792 – 1800 .
- Pratt , K. A. and Prather , K. A. 2009 . Real-Time, Single-Particle Volatility, Size, and Chemical Composition Measurements of Aged Urban Aerosols . Environ. Sci. Technol. , 43 : 8276 – 8282 .
- Ramanathan , V. and Carmichael , G. 2008 . Global and Regional Climate Changes Due to Black Carbon . Nat. Geosci. , 1 : 221 – 227 .
- Russell , L. M. , Maria , S. F. and Myneni , S. C. B. 2002 . Mapping Organic Coatings on Atmospheric Particles . Geophys. Res. Lett. , 29 DOI: 10.1029/2002GL014874
- Russell , L. M. , Bahadur , R. , Hawkins , L. N. , Allan , J. D. , Baumgardner , D. , Quinn , P. K. and Bates , T. S. 2009 . Organic Aerosol Characterization by Complementary Measurements of Chemical Bonds and Molecular Fragments . Atmos. Environ. , 43 : 6100 – 6104 .
- Schnaiter , M. , Linke , C. , Mohler , O. , Naumann , K. H. , Saathoff , H. , Wagner , R. , Schurath , U. and Wehner , B. 2005 . Absorption Amplification of Black Carbon Internally Mixed with Secondary Organic Aerosol . J. Geophys. Res.—Atmos. , 110 DOI: 10.1029/2005JD006046
- Seinfeld , J. H. 2004 . Air Pollution: A Half Century of Progress . Aiche. J. , 50 : 1096 – 1108 .
- Shields , L. G. , Qin , X. Y. , Toner , S. M. and Prather , K. A. 2008 . Detection of Ambient Ultrafine Aerosols by Single Particle Techniques During the SOAR 2005 Campaign . Aerosol Sci. Technol. , 42 : 674 – 684 .
- Silva , P. J. , Liu , D. Y. , Noble , C. A. and Prather , K. A. 1999 . Size and Chemical Characterization of Individual Particles Resulting from Biomass Burning of Local Southern California Species . Environ. Sci. Technol. , 33 : 3068 – 3076 .
- Silva , P. J. and Prather , K. A. 2000 . Interpretation of Mass Spectra from Organic Compounds in Aerosol Time-of-Flight Mass Spectrometry . Anal. Chem. , 72 : 3553 – 3562 .
- Sjogren , S. , Gysel , M. , Weingartner , E. , Baltensperger , U. , Cubison , M. J. , Coe , H. , Zardini , A. A. , Marcolli , C. , Krieger , U. K. and Peter , T. 2007 . Hygroscopic Growth and Water Uptake Kinetics of Two-Phase Aerosol Particles Consisting of Ammonium Sulfate, Adipic and Humic Acid Mixtures . J. Aerosol. Sci. , 38 : 157 – 171 .
- Sodeman , D. A. , Toner , S. M. and Prather , K. A. 2005 . Determination of Single Particle Mass Spectral Signatures from Light-Duty Vehicle Emissions . Environ. Sci. Technol. , 39 : 4569 – 4580 .
- Song , X. H. , Hopke , P. K. , Fergenson , D. P. and Prather , K. A. 1999 . Classification of Single Particles Analyzed by ATOFMS Using an Artificial Neural Network, ART - 2A . Anal. Chem. , 71 : 860 – 865 .
- Su , Y. X. , Sipin , M. F. , Prather , K. A. , Gelein , R. M. , Lunts , A. and Oberdorster , G. 2005 . ATOFMS Characterization of Individual Model Aerosol Particles Used for Exposure Studies . Aerosol. Sci. Technol. , 39 : 400 – 407 .
- Suess , D. T. and Prather , K. A. 1999 . Mass Spectrometry of Aerosols . Chem. Rev. , 99 : 3007 – 3035 .
- Sullivan , R. C. and Prather , K. A. 2005 . Recent Advances in Our Understanding of Atmospheric Chemistry and Climate Made Possible By On-Line Aerosol Analysis Instrumentation . Anal. Chem. , 77 : 3861 – 3885 .
- Sullivan , R. C. , Guazzotti , S. A. , Sodeman , D. A. and Prather , K. A. 2007 . Direct Observations of the Atmospheric Processing of Asian Mineral Dust . Atmos. Chem. Phys. , 7 : 1213 – 1236 .
- Sullivan , R. C. and Prather , K. A. 2007 . Investigations of the Diurnal Cycle and Mixing State of Oxalic Acid in Individual Particles in Asian Aerosol Outflow . Environ. Sci. Technol. , 41 : 8062 – 8069 .
- Takahama , S. , Gilardoni , S. , Russell , L. M. and Kilcoyne , A. L. D. 2007 . Classification of Multiple Types of Organic Carbon Composition in Atmospheric Particles by Scanning Transmission X-Ray Microscopy Analysis . Atmos. Environ. , 41 : 9435 – 9451 .
- Takahama , S. , Gilardoni , S. and Russell , L. M. 2008 . Single-Particle Oxidation State and Morphology of Atmospheric Iron Aerosolsle . J. Geophys. Res.—Atmos. , 113 DOI: 10.1029/2009JD012622
- Takahama , S. , Liu , S. and Russell , L. M. 2010 . Coatings and Clusters of Carboxylic Acids in Carbon-Containing Atmospheric Particles from Spectromicroscopy and Their Implications for Cloud-Nucleating and Optical Properties . J. Geophys. Res.—Atmos. , 115
- Textor , C. , Schulz , M. , Guibert , S. , Kinne , S. , Balkanski , Y. , Bauer , S. , Berntsen , T. , Berglen , T. , Boucher , O. , Chin , M. , Dentener , F. , Diehl , T. , Easter , R. , Feichter , H. , Fillmore , D. , Ghan , S. , Ginoux , P. , Gong , S. , Kristjansson , J. E. , Krol , M. , Lauer , A. , Lamarque , J. F. , Liu , X. , Montanaro , V. , Myhre , G. , Penner , J. , Pitari , G. , Reddy , S. , Seland , O. , Stier , P. , Takemura , T. and Tie , X. 2006 . Analysis and Quantification of the Diversities of Aerosol Life Cycles Within AeroCom . Atmos. Chem. Phys. , 6 : 1777 – 1813 .
- Tivanski , A. V. , Hopkins , R. J. , Tyliszczak , T. and Gilles , M. K. 2007 . Oxygenated Interface on Biomass Burn Tar Balls Determined by Single Particle Scanning Transmission X-Ray Microscopy . J. Phys. Chem. A. , 111 : 5448 – 5458 .
- Turpin , B. J. , Saxena , P. and Andrews , E. 2000 . Measuring and Simulating Particulate Organics in the Atmosphere: Problems and Prospects . Atmos. Environ. , 34 : 2983 – 3013 .
- Turpin , B. J. and Lim , H. J. 2001 . Species Contributions to PM2.5 Mass Concentrations: Revisiting Common Assumptions for Estimating Organic Mass . Aerosol Sci. Technol. , 35 : 602 – 610 .
- Twomey , S. 1977 . Influence of Pollution on Shortwave Albedo of Clouds . J. Atmos. Sci. , 34 : 1149 – 1152 .
- Ward , J. H. 1963 . Hierarchical Grouping to Optimize an Objective Function . J. Am. Stat. Assoc. , 58 : 236 – 244 .
- Yokelson , R. J. , Urbanski , S. P. , Atlas , E. L. , Toohey , D. W. , Alvarado , E. C. , Crounse , J. D. , Wennberg , P. O. , Fisher , M. E. , Wold , C. E. , Campos , T. L. , Adachi , K. , Buseck , P. R. and Hao , W. M. 2007 . Emissions from Forest Fires Near Mexico City . Atmos. Chem. Phys. , 7 : 5569 – 5584 .
- Zhang , Q. , Jimenez , J. L. , Canagaratna , M. R. , Allan , J. D. , Coe , H. , Ulbrich , I. , Alfarra , M. R. , Takami , A. , Middlebrook , A. M. , Sun , Y. L. , Dzepina , K. , Dunlea , E. , Docherty , K. , DeCarlo , P. F. , Salcedo , D. , Onasch , T. , Jayne , J. T. , Miyoshi , T. , Shimono , A. , Hatakeyama , S. , Takegawa , N. , Kondo , Y. , Schneider , J. , Drewnick , F. , Borrmann , S. , Weimer , S. , Demerjian , K. , Williams , P. , Bower , K. , Bahreini , R. , Cottrell , L. , Griffin , R. J. , Rautiainen , J. , Sun , J. Y. , Zhang , Y. M. and Worsnop , D. R. 2007 . Ubiquity and Dominance of Oxygenated Species in Organic Aerosols in Anthropogenically-influenced Northern Hemisphere midlatitudes . Geophys. Res. Lett. , 34 DOI: 10.1029/2007GL029979
- Zimmermann , R. , Ferge , T. , Galli , M. and Karlsson , R. 2003 . Application of Single-Particle Laser Desorption/Ionization Time-of-Flight Mass Spectrometry for Detection of Polycyclic Aromatic Hydrocarbons from Soot Particles Originating from an Industrial Combustion Process . Rapid Commun. Mass. Sp. , 17 : 851 – 859 .