Abstract
Ambient ultrafine particles (UPs or PM 0.1 ), PM 2.5 and PM 10 were investigated at the roadside of Syuefu road in Hsinchu city and in the Syueshan highway tunnel in Taipei, Taiwan. A SMPS (TSI Model 3936), three Dichotomous samplers (Andersen Model SA-241), and three MOUDIs (MSP Model 110) were collocated to determine the PM number and mass concentrations simultaneously. The filter samples were further analyzed for organic carbon (OC), element carbon (EC), water-soluble ions, and trace elements. The OC artifact was studied and quantified using the quartz behind quartz (QBQ) method for all PM fractions. Taking into account the OC artifact, chemical mass closure (ratio of the reconstructed chemical mass to the gravimetrical mass) of PM 0.1 , PM 2.5 , and PM 10 was then calculated and found to be good. The chemical analysis results of UPs at both sites showed that UPs in the present tunnel was mostly contributed from the vehicle emissions while UPs at the roadside was mainly influenced by urban sources.
INTRODUCTION
Researchers have found the associations of the exposure to ultrafine particles (UPs) with the adverse health effects due to bounded elemental/organic carbon, sulfate, elements as well as PAH (polycyclic aromatic hydrocarbons) on UPs (CitationDonaldson et al. 2002; CitationNtziachristos et al. 2007; CitationOberdörster et al. 2005). UPs are referred to as PM0.1 in this study and other researches (CitationArhami et al. 2009; CitationChow and Watson 2007). Sometimes PM0.18 was mentioned as UPs in other studies (CitationGeller et al. 2005; CitationSardar et al. 2005). It is important to assess the mass and chemical species concentrations of UPs accurately. However, only few researches are able to achieve a good chemical mass closure (ratio of the reconstructed chemical mass to the gravimetrical mass) for PM2.5 (CitationTurpin and Lim 2001). But for smaller particles such as UPs, good mass closure has not been found expect in the study of quasi-UP (or PM0.25) by CitationArhami et al. (2009).
Motor vehicles are known to be an important source of ambient UPs and PM2.5 particles which are continuously evolving both chemically and physically in the atmosphere (CitationSchauer et al. 1996). In order to characterize the motor emissions, several different methods were used, including chassis dynamometer experiments (CitationCadle et al. 1999; CitationHildemann et al. 1989; CitationKleeman et al. 2000; CitationRobert et al. 2007a, b; CitationSchauer et al. 1999, Citation2002), roadside measurements (CitationLin et al. 2005; CitationNtziachristos et al. 2007; CitationPhuleria et al. 2007; CitationZhu et al. 2002) and tunnel measurements (CitationAllen et al. 2001; CitationFraser et al. 1998; CitationGrieshop et al. 2006; CitationGeller et al. 2005; CitationHuang et al. 2006; CitationPhuleria et al. 2006; CitationWeingartner et al. 1997). Roadside and tunnel measurements provide more realistic on-road motor emission characteristics and emission factors for PM2.5 (CitationAllen et al. 2001; CitationFraser et al. 1998; CitationGrieshop et al. 2006; CitationPhuleria et al. 2006; CitationWeingartner et al. 1997), and for UPs (CitationGeller et al. 2005; CitationHuang et al. 2006; CitationLin et al. 2005, Citation2009; CitationNtziachristos et al. 2007; CitationPhuleria et al. 2007). In these studies, only CitationGeller et al. (2005) investigated chemical mass closure of PM0.18 in the Caldecott Tunnel, Oakland, CA. However, discrepancy between the chemical reconstructed and gravimetric masses was found, which was suspected due to the adsorption of organic vapor by the quartz filters during sampling. Others studied UPs in the urban areas (CitationCass et al. 2000; CitationSardar et al. 2005), but chemical mass closure was not discussed.
The POC (particulate organic carbon) was normally determined by analyzing particle samples collected on quartz filters using the thermal optical reflectance (TOR) or thermal optical transmission (TOT) method. However, sampling with quartz filters may lead to overestimation or underestimation of POC due to the adsorption of gas-phase OC on the quartz filter (positive artifact) or volatilization of POC from the collected PM (negative artifact), respectively. These artifacts were estimated to range from –80% for volatilization to +50% for adsorption for PM2.5 (CitationTurpin et al. 2000). CitationSubramanian et al. (2004) used the quartz backup filter sampler (TQQQ) to study the positive artifact using a backup quartz filter (QBQ, quartz behind quartz) behind either a main quartz filter (MQ, main quartz) or a Teflon filter (QBT, quartz behind Teflon) in the PM2.5 sampler. Comparison of the POC concentration of the TQQQ sampler with that of a denuder sampler showed that QBQ provided a good estimate of the positive artifact on the MQ when both quartz filters were in equilibrium with the sampled air for OC. But the equilibrium may not always be achieved (Mander and Pankow 2001; CitationSubramanian et al. 2004).
In order to obtain the POM (particulate organic mass) of PM2.5 to achieve a better chemical mass closure, the POC was multiplied by a factor of 1.6 ± 0.2 and 2.1 ± 0.2 (POM/POC) to account for the additional mass of associated H, O, N, and S in the particles in the urban and non-urban locations, respectively (CitationTurpin and Lim 2001). For vehicle emissions, Schauer et al. (1999, 2002) found that the primary emitted PM1.8 from both gasoline vehicles and medium duty diesel trucks had a POM/POC of about 1.2, which was lower than that of the aged urban and non-urban PM2.5. This factor was used in CitationKleeman et al. (2000) in the dynamometer study and CitationHuang et al. (2006) in the tunnel study. In CitationGeller et al. (2005), POM/POC of 1.4 was used in the tunnel study for all coarse (PM2.5–10), accumulation (PM0.18–2.5) and ultrafine (PM0.18) particle fractions.
Up to now, both the POC artifact and the chemical mass closure of PM have been studied mainly for PM2.5. It is of great importance to study these problems for PM0.1, and other PM fractions, such as PM10. The influence of the POC artifact on PM0.1 concentrations determined by the filter method is expected to be very large due to low PM0.1 concentrations. In this study, PM0.1, PM2.5, and PM10 in a highway tunnel and at a roadside were studied and the chemical compositions including organic carbon (OC), element carbon (EC), 9 water-soluble ions and 19 trace elements were obtained. The positive POC artifact was studied using the QBQ method proposed by CitationSubramanian et al. (2004) for PM2.5. Taking into account the positive artifact of OC and by using a reasonable ratio of POC to POM, the chemical mass closure for all PM fractions was examined.
METHODS
Site Description
Field samples were collected at the height of 1.5 m at the roadside of Syuefu road in Hsinchu, and the third relay station inside the Syueshan highway tunnel in Yilan, Taiwan. shows the schematic diagram of the roadside sampling site. Syuefu road has two directions with one lane of 4 m in width in each way. There are six schools along this 800 m-long road and it is one of the main roads leading to the Hsinchu Science Park. The traffic flow is heavy during the morning and evening rush hours when commuters as well as the neighborhood residents may expose to high concentration of PM. Sampling was conducted at the sidewalk at the midpoint of the 800 m-long road and about 4 m from the edge of the traffic lane.
The Syueshan highway tunnel is the longest highway tunnel in Taiwan with a total length of 12.9 km in which only shuttle buses, passenger cars and light-duty diesel trucks are allowed. shows the schematic diagram of the tunnel which has two two-lane bores and inclines downward from the west (Pinglin direction, Taipei County) to the east (Toucheng direction, Yilan County) with a slope of 1.3%. There are three ventilation and three relay stations located alternatively with an equal distance of 2 km in between or they are located at 1.5, 3.5, 5.5, 7.5, 9.5, and 11.5 km from the entrance of the tunnel. Sampling was conducted at the third relay stations located 1.4 km from the outlet (Toucheng direction, 11.5 km from the inlet of Pinglin), at a distance of 2 m from the edge of the traffic lane. The tunnel does not have distinct morning and evening rush hours except during the weekend and holidays. In each ventilation station, a huge fan was installed in one vertical well to transport ambient fresh air into the tunnel and while the other fan was installed in another well to exhaust polluted air off the tunnel. However, these fans are seldom activated. The fans in the relay stations, which exchange the air between the two bores, are also normally closed. There are two axial fans mounted on the top of the bores at every 0.5 km along the tunnel. They are activated when the temperature in the tunnel exceeds 40°C. To avoid sporadic fan operation, these fans were disabled during the sampling periods.
Sampling was conducted from January to December of 2008, during which six 24-h samples were taken at the roadside, sixteen samples were taken in the tunnel, in which ten 3-h samples were taken at daytime (during AM 09:00 to PM 09:00), three 6-h samples were taken at midnight from AM 00:00 to 06:00 and the other three 3-h samples were taken from AM 08:00 to 11:00 on holidays (rush hour).
Sampling Protocol
PM2.5–10 and PM2.5 samples were collected by using three Dichotomous samplers (Model SA-241, Andersen Inc., Georgia, USA). Hereafter they are referred to as Dichots in this study. After obtaining PM2.5–10 and PM2.5, PM10 was calculated as the sum of PM2.5–10 and PM2.5. shows the configurations of the samplers. The PM0.1 samples were collected at the afterfilters of the three 10-stage MOUDIs (Model 110, MSP Corp., MN, USA) operated in parallel. A collocated SMPS (Model 3936, TSI Inc., MN, USA) equipped with Nano-DMA (TSI Model 3085) and Ultrafine Water-based Condensation Particle Counter (UWCPC, TSI Model 3786) was used to monitor the number size distributions of ambient PM0.005–0.2 simultaneously. In the MOUDIs, the 3.2 μm cutsize stages were replaced with 2.5 μm cutsize stages and the nozzle plates of the stage 10 with 56 nm cutsize was removed since only PM0.1 was studied. Thus, the cutsizes of the MOUDIs were 18, 10, 5.6, 2.5, 1.8, 1.0, 0.56, 0.32, 0.18, and 0.1 μm. In addition, temperature and relative humidity were recorded and a video camera was used to record the traffic flow at both sites.
In two MOUDIs (M1 and M2), silicone grease (KF-96-SP, Topco Technologies Corp., Taiwan) coated aluminum foils were used as the impaction substrates in 0–9 stages to reduce solid particle bounce (CitationPak et al. 1992). The samples collected were weighed to determine mass distributions. Teflon (Zefluor P5PJ047, Pall Corp., New York, USA) and quartz filters (Tissuqartz 2500QAT-UP, 7201&7202, Pall Corp., New York, USA) were used in M1 and M2, respectively, as the afterfilters for further gravimetric (M1 only) and chemical analysis (both M1 and M2). M2 was used to determine the OC and EC concentrations of PM0.1, in which two quartz filters (MQ and QBQ) were placed in series at the afterfilter stage of M2. The third MOUDI (M3) was used to determine whether the quartz filters were equilibrium in OC concentration. A HEPA filter (HEPA Capsule 12144, Pall Corp., New York, USA) was installed at its inlet to remove all PMs. The impaction substrates from stage 0 to 9 were not used in M3 while only two quartz filters, QBH (quartz behind HEPA) and QBH1 (quartz behind QBH), were placed in series at the afterfilter stage. This setup, which is called QBH method in this study (detailed description will be shown later), was similar to the QBT method used in CitationSubramanian et al. (2004) who used only one quartz filter after the Teflon filter in the study.
The POC concentration can be calculated based on the OC concentration of the first quartz (MQ) and the second quartz (QBQ) of the M2, when the equilibrium of OC concentration between the two quartz filters and the air stream was achieved (or when the quartz filters were saturated with gaseous OC) (CitationSubramanian et al. 2004) as:
Similarly, Teflon filters were used in Dichot 1 (D1) while quartz filters were used in both Dichot 2 and 3 (D2 and D3) in fine and coarse particle channels. The QBQ method was again used in D2 (MQ and QBQ in series) to correct for the POC artifact while the QBH method was used in D3 (QBH and QBH1 in series) to check whether the quartz filters were equilibrium in OC concentration. The HEPA capsules installed at the inlet of the M3 and D3 provide high flow rate at a low pressure drop. It was found the two quartz filters, QBH and QBH1, had very close OC concentration (<10% in difference) for all PM0.1 and PM2.5 samples at both roadside and tunnel sites due to long sampling time and high face velocity (about 0.4 and 0.8 m/s for Dichot and MOUDI, respectively). Therefore, Equation (Equation1) was used to calculate the POC concentration of PM0.1 and PM2.5 for all measurements. On the other hand for coarse particles, it was observed the QBH had a higher OC concentration than the QBH1 for all roadside and tunnel samples due to a relatively lower sampling flow rate (∼0.04 m/s of face velocity) of the coarse particle channel in the D3, indicating that the equilibrium was not achieved. Thus, Equation (Equation2) was used to calculate the POC of PM2.5–10 for all samples. The application of Equation (Equation2) to correct for the positive OC artifact for PM2.5–10 may lead to a small error. Nevertheless, the error will not affect the present mass closure result of PM10 because the OC concentration of PM2.5–10 was much lower than that of PM2.5 at both sites.
In every sampling run, at least two Teflon filters, two quartz filters and two silicone grease coated aluminum foils (or >10% of sample number) were used as laboratory and field blanks, respectively, to examine the background levels of the gravimetric and chemical analysis. Teflon filters were conditioned at least 24 h in a temperature and relative humidity controlled room (22 ± 1°C, 40 ± 5% RH) before sampling. All quartz filters were pre-baked at 900°C for 3 h to reduce organic blank level. 0.3 to 0.5 mg of Silicone grease (KF-96-SP, Topco Technologies Corp., Taiwan) was coated uniformly on each aluminum foil of the M1 and M2 stages (CitationPak et al. 1992). After coating, the foils were baked in an oven at 65°C for 1.5 h (CitationMarple et al. 1991).
Because the chemical compositions as well as the mass concentration of PM0.1 were determined from different filter samples of three collocated MOUDIs, mass concentration distributions of the three MOUDIs were compared with each other. The PM0.1 concentration of MOUDI (M1) was also compared with the calculated PM0.1 concentration based on the SMPS data and the apparent particle density determined by using the method of CitationSpencer et al. (2007). CitationSpencer et al. (2007) found the particle effective density increased with decreasing absolute atmospheric water content.
Sample Analysis
Before chemical analysis, all Teflon samples of M1, D1, and blanks were weighed first to determine the mass concentrations of the PM samples. The electrostatic charge of the filters was eliminated by an ionizing air blower (Model CSD-0911, MEISEI, Japan) before weighing. A microbalance (Model CP2P-F, Sartorius, Germany) was used to weigh the filters after they were conditioned at least 24 h in the temperature and relative humidity controlled room (22 ± 1°C, 40 ± 5% RH). After gravimetric analysis, each Teflon filter was cut equally in half using a Teflon coated scissor. One half was analyzed by an ICP-MS (Model 7500 series, Agilent Technologies, Inc., USA) for elements except Si which was analyzed by ICP-AES (Model Optima 2000DV for Si, PerkinElmer, Inc., MA, USA) while the other half was analyzed by an ion chromatograph (IC, Model DX-120, Dionex Corp, Sunnyvale, CA) for ionic species. The analyzed elements included major (crustal) elements (Na, Mg, Al, K, Ca, Fe, and Si) and sub-major (anthropogenic) elements (S, Zn, Ni, Cu, Mn, Sr, Ag, Ba, Pb, V, Cr, and Ti). The analyzed ions were F–, Cl–, NO– 3, SO–2 4, NH+ 4, Na+, K+, Mg+2, and Ca+2. Except Si and Na, the detection limit for ionic and elemental species was less than 0.003 and 0.002 μg/m3, respectively. The Si and Na had a detection limit of 0.02 μg/m3 which was an order of magnitude higher than the other species.
Quartz filter samples of D2, D3, M2, M3, and blanks were analyzed by the thermal-optical reflectance (TOR) method for OC and EC concentrations without gravimetric analysis. The quartz samples were stored in a –18°C freezer immediately after sampling. The detection limit for OC and EC in this study was 0.06 and 0.01 μg/m3, respectively. The EC was estimated from the analyzed results of MQ (CitationSubramanian et al. 2004).
After obtaining the POC, the POM was calculated by multiplying it with a proper POM/POC ratio. Because the tunnel PMs were contributed mainly by vehicle emissions and the POM/POC value of 1.4 was applied for all PM fractions. For the roadside PMs, the value of 1.6 was applied because this site was located in the urban area (CitationTurpin and Lim 2001).
RESULTS AND DISCUSSION
PM Concentration and Mass Distribution
The comparison of mass distributions of ambient particles between the three parallel MOUDIs showed a good agreement for all size intervals. Using the method in CitationSpencer et al. (2007), the effective density of PM0.1 was found to be 1.25 ± 0.14 and 1.40 ± 0.10 g/cm3 in the tunnel and at the roadside, respectively, which was then used to convert the SMPS number concentrations to mass concentrations for PM0.1. Results showed the calculated PM0.1 concentrations of the SMPS were similar to those of the MOUDIs with a relative difference of less than 30%.
In this study, the average mass concentration and standard deviation of PMs were calculated from pooled samples and shown in as “average ± standard deviation.” It is seen the PM10 and PM2.5 concentrations of the MOUDI are in agreement with those of the Dichot with a relative deviation of <20%. In the tunnel, the PM concentrations were found to increase with increasing traffic flow rates, which were about 410 ± 70, 1250 ± 280, and 2040 ± 430 vehicles/h during the midnight (mean of all three 6-h measurements), daytime (mean of all ten 3-h measurements), and rush hour (mean of all three 3-h measurements) measurements, respectively. Variation of PMs mass concentration at different sampling days was small because vehicle emission was the most predominant source in the tunnel and the vehicle flow rate and fleet composition remained to be similar. In comparison, although the traffic flow rate of 1130 ± 95 vehicles/h (mean of six 24-h measurements) and fleet composition (to be shown later) were similar during the six roadside measurements, concentrations of PMs were quite different with a relatively larger standard deviation due to the influence of background sources and meteorological conditions.
TABLE 1 Comparison of PM concentration (μg/m3) between MOUDI (M) and Dichot (D) at the roadside and in the tunnel
The mass concentration of PM0.1 at the roadside is 2.21 ± 0.59 μg/m3 (mean of six 24-h measurements), which is comparable to the concentration at the urban areas in LA obtained by CitationCass et al. (2000) and CitationSardar et al. (2005), who showed that UP concentrations were 0.55–1.16 μg/m3 (PM0.056–0.1) and 0.86–3.5 μg/m3 (PM0.01–0.18), respectively. Compared to the roadside, significantly higher concentrations of 33.2 ± 6.5 and 46.5 ± 5.2 μg/m3 were observed in the tunnel during the daytime as well as the rush hour, respectively. Even in the midnight, relatively high PM0.1 concentration of 9.1 ± 1.7 μg/m3 was found in the tunnel. High PM0.1 concentration observed in the 3rd relay station suggests the air quality inside and near the tunnel exit is of serious concern.
shows the comparison of average mass distributions of particles between the roadside and the tunnel in the daytime. It is seen that particles at both sites are bimodal. The saddle point which divides the accumulation and coarse modes is about 0.4 and 1.0 μm at the tunnel and roadside, respectively. In the tunnel, the accumulation mode peaks at 0.1–0.2 μm, which is similar to that found in the dynamometer study for both gasoline and diesel vehicles (CitationKleeman et al. 2000), while the coarse mode peaks at about 2.5 μm. It is observed that the accumulation mode particles have much higher concentration than the coarse mode particles. In comparison, the concentration of the coarse mode (peaks at 6 μm) is higher than the accumulation mode (peaks at 0.3 μm) at the roadside. From , it can be concluded that the present tunnel with predominant sources of vehicle emission contains much more UPs and fine particles than coarse particles. In comparison, the background sources contributed larger fraction of coarse particles at the roadside.
Chemical Mass Closure of PMs
shows the comparison of chemical compositions (%) of all PM fractions in the tunnel (T, mean of all ten daytime measurements) and at the roadside (R, mean of all six measurements). In the figure, good chemical mass closure is seen at both sites with unknown compositions of less than 30%. The chemical mass of PM0.1, PM2.5, and PM10 is 73.8 ± 5.3, 97.8 ± 8.5, and 86.7 ± 5.0% for the gravimetric mass, respectively, in the tunnel, and it is 89.4 ± 9.6, 87.9 ± 12.0, and 75.3 ± 5.7% at the roadside. Better mass closure for PM0.1 was obtained than that in CitationGeller et al. (2005) due to the correction of the OC artifact and use of an appropriate value of POM/POC in the present study.
FIG. 4 Average chemical compositions (%) of PMs at Syuefu roadside and in the Syueshan highway tunnel. One standard deviation of each data point ranges from 1.6 to 9.7% depending on the PM fractions and chemical compositions.
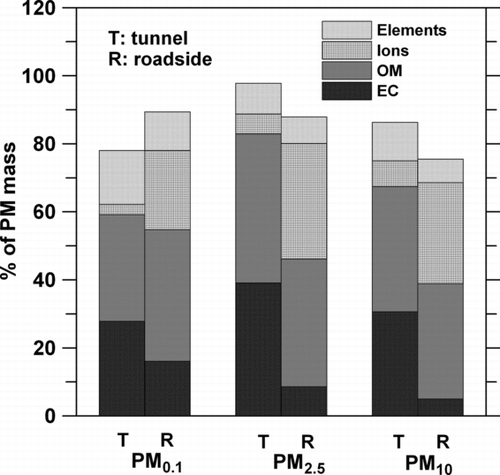
The OC artifact was found to account for 51.6 ± 10.7% and 20.0 ± 5.4% of the PM0.1 at the roadside and the tunnel, respectively. For PM2.5 and PM10, less severe artifact was found at both sites, which was 7.5 ± 2.8 and 5.8 ± 1.3% of the particle mass at the roadside while it was 12.9 ± 4.1% and 16.3 ± 4.3% in the tunnel, respectively. Severe OC artifact in PM0.1 at the roadside is due to the relatively low PM0.1 mass concentration and longer sampling period of 24 h. The achievement of good mass closure for PM0.1, PM2.5, and PM10 indicates the correction of OC artifact proposed by CitationSubramanian et al. (2004) and the use of POC to POM ratio suggested by CitationTurpin and Lim (2001) for PM2.5 (for roadside) and CitationGeller et al. (2005) for PM2.5–10, PM0.18–2.5, and PM0.18 (for tunnel) are reasonable for these two PM fractions. The present mass closure of PM2.5 was compared to the studies of PM2.5 at downtown LA, desert area of Meadview, AZ and Look-Rock, TN which did not correct for the OC artifact (CitationTurpin and Lim 2001). Similar mass closure of 77–100% in this study to those of 70–99% in CitationTurpin and Lim (2001) was found.
Chemical Characteristics of PMs
Contribution of Background Source to the Tunnel and Roadside
The potential contribution of background air to the levels measured in the present two sampling sites was investigated before discussing the contribution from vehicles. In fact, the present two sites were influenced by urban aerosols rather than the remote background air because the distance from the tunnel entrance to Taipei metropolis is only 20 km and the roadside sampling site is located inside Hsinchu city. The aerosols at the tunnel inlet were expected to have similar concentrations and compositions to the Taipei aerosols which were continuously monitored at the Taipei supersite. Thus, we used the data from the Taipei supersite as the background source of the tunnel and to compare with the results of the present roadside. The supersite is located in a 10930 m2 park at the ground level near the center of Taipei metropolis, which is influenced by typical urban aerosols. The daily concentrations of PM10 and PM2.5, and OC, EC, sulfate, nitrate concentrations of PM2.5 can be found at http://taqm.epa.gov.tw/taqm/zh-tw/PmlDaily.aspx. The average PM2.5 concentration at the supersite during the sampling days (ten days during January to December 2008) was 23.1 ± 9.6 μg/m3 which was only 1/3 of that at the 3rd relay station. The sum of sulfate and nitrate contributed 33.4 ± 6.4% mass to PM2.5 at the supersite, and was expected to contribute 11.1% to the PM2.5 mass at the 3rd relay station in the tunnel assuming water-soluble ions were negligible in fresh vehicle PM2.5 emissions in the tunnel (<2%, CitationRobert et al. 2007a). However, the value was much larger than the measured value of 4.5% at the 3rd relay station, indicating the contribution of urban PM2.5 to the tunnel PM2.5 should be much less than 1/3, and was estimated to be about 13.5%.
During the sampling days at the tunnel, the average OC/EC of PM2.5 at the supersite was 2.9 ± 0.5 (2.4–3.9), while the ratio was 1.0 ± 0.1 (0.84–1.17) at the 3rd relay station. Similar to sulfate and nitrate analysis, the OC/EC was found to be 1.22–2.35 considering that 1/3 of PM2.5 was contributed by urban aerosols, 2/3 was contributed by vehicle emissions in the tunnel at the 3rd relay station. The OC/EC of vehicle emissions in the tunnel was calculated based on the fleet compositions, OC/EC and emission factor for gasoline and diesel vehicle emissions proposed by Schauer et al. (1999, 2002) and CitationWeingartner et al. (1997), respectively. The calculated OC/EC, 1.22–2.35, was found to be much higher than the measured data, 0.84–1.17, again indicating that the contribution of urban PM2.5 to the tunnel PM2.5 should be much less than 1/3. Based on the estimated contribution of urban PM2.5 to the tunnel PM2.5, 13.5%, the OC/EC was found to be 0.88–1.89, which was closer to the measured OC/EC at the 3rd relay station.
Since the PM10 had a similar characteristics as PM2.5 in the aspect of the mass ratio of the urban to tunnel aerosols, OC/EC ratio for diesel and gasoline vehicles emissions (CitationCadle et al. 1999; CitationSchauer et al. 1999, Citation2002) and the percentage of OC and ion in the PM mass, it was expected the Taipei urban PM10 also contributed to less than 1/3 of the tunnel PM10. For the PM0.1 in the tunnel, the background source could be neglected due to a relatively lower urban PM0.1 concentration (normally 1–3 μg/m3) than that in the present 3rd relay station (33.2 ± 6.5 μg/m3).
OM and EC
shows that OM is the most abundant species for all PMs at both sites, which agrees with other urban or roadside measurements (CitationCass et al. 2000; CitationPhuleria et al. 2007; CitationSardar et al. 2005; CitationSpencer et al. 2007) and the tunnel or chassis dynamometer studies (CitationGeller et al. 2005; CitationHuang et al. 2006; CitationKleeman et al. 2000). OM accounted for 37.2 ± 5.5, 43.8 ± 6.9, and 31.4 ± 5.1% particle mass in the tunnel while it contributed 33.7 ± 6.6, 37.5 ± 9.7, and 38.7 ± 3.3% to particle mass at the roadside for PM10, PM2.5, and PM0.1, respectively. Similar and high mass percentage of OM in all PM fractions was found at both sites, indicating that the fraction of OM in PM was similar for background and vehicle emitted PMs.
EC accounted for 32.6 ± 4.5, 39.1 ± 5.8, and 27.8 ± 5.4% in PM10, PM2.5, and PM0.1 the tunnel, while it was 5.0 ± 1.3, 8.6 ± 2.3, and 16.1 ± 7.5% at the roadside, respectively. The tunnel PMs had a much higher mass percentage of EC than that at the roadside especially for PM2.5 and PM10. The highest percentage of EC in the tunnel PM2.5 was in agreement with that found in the literature (CitationHuang et al. 2006; CitationKleeman et al. 2000) in which the MMAD (mass median aerodynamic diameter) of EC was shown to be 0.2–0.4 μm (or mostly outside the range of PM0.1). On the other hand, the roadside PM0.1 had a much higher percentage of EC than that in PM2.5 and PM10 because PM0.1 contained more freshly generated UPs by vehicles (with high EC constituent) while roadside PM2.5 and PM10 contained more aged ambient fine and coarse particles (higher ion and OC constituents). The existence of considerable high concentration of OM and EC in the tunnel PM0.1 and PM2.5 is of serious environmental and health concerns.
In the tunnel, the video records showed the composition of passenger cars, light-duty diesel trucks and shuttle buses was 91.6 ± 3.6, 6.5 ± 2.7, and 1.9 ± 0.4%, respectively, in the daytime when the total vehicle flow rate was 1250 ± 280
vehicles/h. In the passenger cars, there was about 5% of diesel engine. In Table 3 of CitationWeingartner et al. (1997), the emission factor (EF, mg/km) of gasoline and diesel vehicles for PM1.3, PM2.5, PM10, and TSP (total suspended particulate) based on tunnel measurements was compared and the ratio of EF for diesel to that of gasoline vehicles was found to be 45 and 66 for PM10 and PM2.5, respectively. More recently, Robert et al. (2007a, b) found the average emission factor for diesel to gasoline vehicles for PM0.1 was about 10. Thus, although the ratio of the number of the diesel vehicles to the number of the gasoline vehicles in the present tunnel was low (∼8%), more than 45, 80, and 85% of tunnel PM0.1, PM2.5, and PM10, respectively, was contributed from diesel vehicles due to their high emission factors. Besides, CitationCadle et al. (1999) found the ratio of OC to EC (OC/EC) in PM10 for the diesel and gasoline vehicles was 0.3–0.9 and >2.0 (without correcting for OC artifact), respectively. For fine particles, Schauer et al. (1999, 2002) found the OC/EC in PM1.8 for diesel and gasoline vehicles was about 0.6 and 4.3 (without correcting for OC artifact), respectively. In addition, the OC/EC of PM0.056–0.1 was found to be about 0.3 and 1.2 for diesel and gasoline vehicles, respectively (CitationRobert et al. 2007a, b). The above OC/EC data show that PM1.8 and PM10 have a close OC/EC for diesel and gasoline vehicles, respectively. The average OC/EC of both PM1.8 and PM10 was about 0.6 and 3.5 for diesel and gasoline vehicles, respectively. Using the OC/EC values of 0.6 and 3.5 for diesel and gasoline vehicles, respectively, for both PM2.5 and PM10, the present OC/EC of PM2.5 and PM10 for the vehicle fleet of the daytime in the Syueshan Tunnel was found to be about 1.2 which is in good agreement with the measured value in the present tunnel of 1.0 ± 0.1 without correcting for the OC artifact. For PM0.1, good agreement between the measured OC/EC of 1.0 ± 0.1 and the calculated value of bout 0.8 was also found. That is, the measured OC/EC for all PM fractions of this study was reasonable. Taking into account the OC artifact, the OC/EC was 0.8 ± 0.1 for all PM fractions in the present tunnel.
For the roadside, the traffic flow rate was 1130 ± 95
vehicles/h and the average composition of motorcycles, passenger cars, light-duty diesel trucks, and shuttle buses was 56.3 ± 6.2, 40.6 ± 1.7, 2.0 ± 0.8, and 1.2 ± 0.2% (mean of six 24-h measurements), respectively. Although motorcycles dominated the vehicle number, they only contributed a very low amount to the PMs because their emission factor was relatively low, which was only about 10% of that of the passenger cars (CitationYang et al. 2005). Based on the fleet compositions, about 25, 75, and 82% of roadside PM0.1, PM2.5, and PM10, respectively, was attributed to diesel vehicle emissions. Since the close percentage of PM2.5 and PM10 mass was attributed to diesel vehicle at both sites, the OC/EC should have been close to each other. However, it was observed the roadside had much higher OC/EC values of 1.5 ± 0.7, 2.7 ± 0.6, and 4.4 ± 1.3 for PM0.1, PM2.5, and PM10, respectively, than the value of 0.8 ± 0.1 in the tunnel. Nevertheless, the average OC/EC of the PM2.5 in the present urban roadside was very close to the average value in 2008 of the Taipei supersite, which was 2.8 ± 0.6. From the comparison, it showed that the vehicle emission dominated tunnel had a lower OC/EC than the background source dominated roadside for all PM fractions. A closer OC/EC of PM0.1 than that of PM2.5 and PM10 between the two sites indicated that local emissions of vehicles increased the ratio of EC in PM0.1 much more than that in the other two PM fractions.
Water-Soluble Ions
The contribution of ionic species to total PM was lower than 10% in the tunnel, while it was greater than 25% at the roadside for all PM fractions. The ion percentages of PM10, PM2.5, and PM0.1 for the tunnel and roadside is 7.5 ± 3.0, 5.9 ± 3.2, 3.0 ± 1.6%, and 32.0 ± 7.2, 36.2 ± 6.5, 24.8 ± 9.3%, respectively, as shown in . The percentage of the total water-soluble ions to PM2.5 and PM10 mass at the roadside was very close to the data at the Taipei supersite and a central monitoring station in Taipei city. The annual average percentage in 2008 of the PM2.5 and PM10 at these two stations was 36.4 ± 10.2 and 33.0 ± 10.5%, respectively. The high water-soluble ion content in the roadside particles is because they are aged and more secondary sulfates, nitrates, and other inorganic salts are formed (CitationCass et al. 2000; CitationSardar et al. 2005; CitationLin et al. 2009). The sulfates and nitrates are normally produced by photochemical oxidation of gas phase precursors such as SO2 and NOx on pre-existing aerosols. This process takes at least several hours. Therefore, the “fresher” tunnel particles were found to have a relatively lower mass percentage of ions (3–7.5%), which agrees with other tunnel and dynamometer studies (CitationKleeman et al. 2000; CitationHuang et al. 2006; CitationGeller et al. 2005; CitationRobert et al. 2007a).
shows the comparison of water-soluble ion concentration of PM0.1, PM2.5, and PM10 between the tunnel and roadside. It is seen the SO–2 4 is the most abundant (45.3 ± 13.1% of total ionic species) ionic species in any size fractions at the roadside, followed by NH+ 4 (16.8 ± 5.5%), NO– 3
(15.1 ± 6.9%), and Ca+2 (7.5 ± 2.1%). The tunnel PMs also showed a similar trend. It was observed that although relatively higher PM concentrations in the tunnel than that at the roadside, total soluble ion concentrations of the roadside PMs were higher than those in the tunnel for both PM2.5 and PM10.
Elements
The percentage of elements in PM10, PM2.5, and PM0.1 for the tunnel and roadside was 11.3 ± 2.6, 9.1 ± 2.1, 11.6 ± 2.6%, 6.9 ± 1.9, 7.8 ± 2.0, and 11.3 ± 3.3%, respectively. Similar percentage of elements was found for all PM fractions in the tunnel indicating elements were distributed uniformly in every size fractions. This trend was also found for the roadside and tunnel OMs. However, much higher percentage of elements was found for PM0.1 than PM2.5 and PM10 at the roadside, similar to that of EC at the roadside. The finding that OM, EC, and elements had higher percentage of PM0.1 mass than that in PM2.5 and PM10 at the roadside implies that on-road vehicle emissions made a large contribution to the UP mass.
Figures and b show the element concentrations of PMs at the roadside and tunnel, respectively. The elements are divided into two groups: major (crustal) (Na, Mg, Al, K, Ca, Fe, and Si, left-hand side of the figure) and sub-major (anthropogenic) elements (S, Zn, Ni, Cu, Mn, Sr, Ag, Ba, Pb, V, Cr, and Ti, right-hand side of the figure). In the tunnel, the mean concentrations of the major elements in PM0.1, PM2.5, and PM10 were 2.49 ± 0.22, 5.13 ± 0.49, and 7.85 ± 1.02 μg/m3, respectively, and those of sub-major elements were 0.83 ± 0.16, 1.50 ± 0.23, and 2.78 ± 0.33 μg/m3, respectively. In comparison, the mean concentrations of the major elements in roadside PM0.1, PM2.5, and PM10 were 0.13 ± 0.04, 1.23 ± 0.17, and 2.95 ± 0.33 μg/m3, respectively, and those of sub-major elements were 0.10 ± 0.02, 0.57 ± 0.09, and 1.19 ± 0.18 μg/m3, respectively. As opposed to the ions, the tunnel particles had higher total element concentration than that at the roadside. Further analysis showed that PM2.5/PM10 and PM0.1/PM10 of the tunnel sub-major elements were 0.54 and 0.30, respectively, while those of the roadside were 0.48 and 0.08, respectively. Much lower PM0.1/PM10 value at the roadside than that in the tunnel indicates that the background sources contribute much more sub-major elements to PM10 than PM0.1.
FIG. 6 Concentrations of elements in all PM fractions at the roadside (a) and in the tunnel (b). Error bars represent one standard deviation.
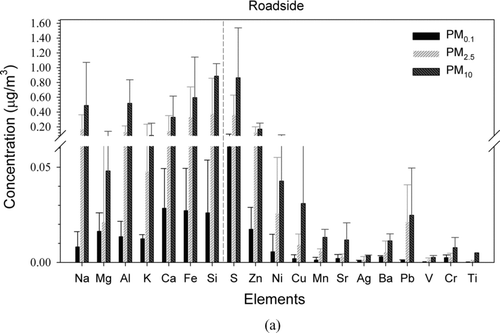
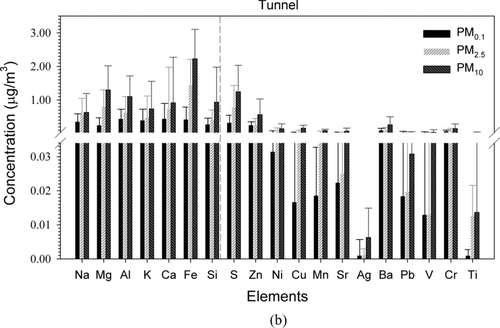
shows the ratio of the total element concentration of PMs as well as individual element concentration in the tunnel to that of the roadside (T/R). It can be seen that the T/R of PM0.1, PM2.5, and PM10 is 16.9, 3.15, and 1.93, respectively. For comparison, CitationPalmgren et al. (2003) found the emitted particles of gasoline and diesel engines were mostly in the nucleation mode (<30 nm) and ultrafine mode (30–100 nm), which is the main reason why the T/R of PM0.1 in this study is significantly higher than the T/R of PM10 and PM2.5. It is also seen that the every individual element in PM10 and PM2.5 has a similar T/R value while that of PM0.1 shows a quite different trend. Compared with the T/R value of total elements in PM0.1, individual element in PM0.1 including Na, Al, K, Cr, V, and Ba has higher T/R. Since V and Ba are the additives of fuel, high T/R values of V and Ba indicate that motor emission is the dominant source for PM0.1 in the tunnel. The present results of higher Pb and Zn T/R values for PM0.1 than PM2.5 and PM10 in the tunnel are also in agreement with CitationMonaci et al. (2000) who found the particle-bound Pb, Zn, Ni, and Ba were produced from the gasoline and diesel engines. Other elements such as Na, Al, K, Ca, V, Fe, and Si also showed higher T/R in PM0.1 than in PM2.5 and PM10, in which Si, Al, Ca, and Fe were the major bounded elements of diesel engine emission (CitationWang et al. 2003). This explains why higher T/R value for Si, Al, Ca, and Fe in PM0.1 than that in PM2.5 and PM10 was found in the tunnel in this study.
CONCLUSION
Atmospheric PM0.1, PM2.5, and PM10 were sampled at the roadside of Syuefu road in Hsinchu city and in the Syueshan highway tunnel in Taipei, Taiwan using three MOUDIs and three Dichots. The samples of all PM fractions were analyzed for organic carbon (OC), element carbon (EC), water-soluble ions, and trace elements. By adopting the QBQ methods to account for the positive artifact of OC, it was found the artifact could be as high as 51.6 ± 10.7% and 20.0 ± 5.4% of the PM0.1 mass for the roadside and tunnel, respectively. Because the PM concentration was larger at larger size fractions, the percentage of artifact was smaller for PM2.5 and PM10, which was 7.5 ± 2.8 and 5.6 ± 1.3% of the particle mass at the roadside while it was 12.9 ± 4.1% and 16.7 ± 4.4% in the tunnel, respectively. Applying the POC to POM ratio proposed by CitationTurpin and Lim (2001) for PM2.5 and CitationGeller et al. (2005) for PM0.18 to the present roadside and tunnel PM0.1, PM2.5, and PM10 samples, respectively, good chemical mass closure was achieved for all PMs. Therefore in the present study, the OC artifact was corrected accurately and a value of POM/POC was used appropriately in the present study. In the future, it is necessary to determine the OC artifact more accurately for PM0.1 using the VOC denuder method similar to CitationSubramanian et al. (2004) for PM2.5 and CitationEiguren-Fernandez et al. (2003) for PAH.
The OM is the most abundant species for all PMs at both sites. The roadside and tunnel particles have similar mass fractions of OM in every size fractions. The tunnel particles have much higher mass percentage of EC than that at the roadside especially for the PM2.5 and PM10. The highest percentage of EC in the tunnel PM2.5 is because that EC is in the accumulation mode and mostly outside the range of PM0.1. The percentage of ion in all PM fractions is much lower in the tunnel than that at the roadside because particles are aged and formed with more secondary sulfates, nitrates and other inorganic salts at the roadside. Elements of PM10 and PM2.5 have similar T/R values while elements of PM0.1 show higher T/R values. The Pb, Zn, V, and Ba has higher T/R value for PM0.1 than PM2.5 and PM10, indicating that motor emission is the dominant source for PM0.1 in the tunnel.
The financial support of the Taiwan EPA (EPA-96-U1U1-02-104 and EPA-97-U1U1-02-106) is gratefully acknowledged.
REFERENCES
- Allen , J. O. , Mayo , P. R. , Hughes , L. S. , Salmon , L. G. and Cass , G. R. 2001 . Emissions of Size-Segregated Aerosols from On-Road Vehicles in the Caldecott Tunnel . Environ. Sci. Technol. , 35 : 4189 – 4197 .
- Arhami , M. , Sillanp , M. , Hu , S. , Olson , M. R. , Schauer , J. J. and Sioutas , C. 2009 . Size-Segregated Inorganic and Organic Components of PM in the Communities of Los Angeles Harbor . Aerosol Sci. Technol. , 43 : 145 – 160 .
- Cadle , S. H. , Mulawa , P. A. , Hunsanger , E. C. , Nelson , K. , Ragazzi , R. A. , Barrett , R. , Gallagher , G. L. , Lawson , D. R. , Knapp , K. T. and Snow , R. 1999 . Composition of Light-Duty Motor Vehicle Exhaust Particulate Matter in the Denver, Colorado Area . Environ. Sci. Technol. , 33 : 2328 – 2339 .
- Cass , G. R. , Hughes , L. A. , Bhave , P. , Kleeman , M. J. , Allen , J. O. and Salmon , L. G. 2000 . The Chemical Composition of Atmospheric Ultrafine Particles . Philos. Trans. R. Soc. London, Ser. A. , 358 : 2581 – 2592 .
- Chow , J. C. and Watson , J. G. 2007 . Survey of Measurement and Composition of Ultrafine Particles . Aerosol Air Qual. Res. , 7 : 121 – 173 .
- Donaldson , K. , Brown , D. , Clouter , A. , Duffin , R. , MacNee , W. , Renwick , L. , Tran , L. and Stone , V. 2002 . The Pulmonarytoxicology of Ultrafine Particles . J. Aerosol Med.—Deposition Clearance and Effects in the Lung. , 15 : 213 – 220 .
- Eiguren-Fernandez , A. , Miguel , A. H. , Jaques , P. A. and Sioutas , C. 2003 . Evaluation of a Denuder-MOUDI-PUF Sampling System to Measure the Size Distribution of Semi-Volatile Polycylic Aromatic Hydrocarbons in the Atmosphere . Aerosol Sci. Technol. , 37 : 201 – 209 .
- Fraser , M. P. , Cass , G. R. and Simoneit , B. R. T. 1998 . Gas-Phase and Particle-Phase Organic Compounds Emitted from Motor Vehicle Traffic in a Los Angeles Roadway Tunnel . Environ. Sci. Technol. , 32 : 2051 – 2060 .
- Geller , M. D. , Sardar , S. B. , Phuleria , H. , Fine , P. M. and Sioutas , C. 2005 . Measurement of Particle Number and Mass Concentrations and Size Distributions in a Tunnel Environment . Environ. Sci. Technol. , 39 : 8653 – 8663 .
- Grieshop , A. P. , Lipsky , E. M. , Pekney , N. J. , Takahama , S. and Robinson , A. L. 2006 . Fine Particle Emission Factors from Vehicles in a Highway Tunnel: Effects of Fleet Composition and Season . Atmos. Environ. , 40 : S287 – S298 .
- Hildemann , L. M. , Cass , G. R. and Markowski , G. R. 1989 . A Dilution Stack Sampler for Collection of Organic Aerosol Emissions: Design, Characterization and Field Tests . Aerosol Sci. Technol. , 10 : 193 – 204 .
- Huang , X. F. , Yu , J. Z. , He , L. Y. and Hu , M. 2006 . Size Distribution Characteristics of Elemental Carbon Emitted from Chinese Vehicles: Results of a Tunnel Study and Atmospheric Implications . Environ. Sci. Technol. , 40 : 5355 – 5360 .
- Kleeman , M. J. , Schauer , J. J. and Cass , G. R. 2000 . Size and Composition Distribution of Fine Particulate Matter Emitted from Motor Vehicles . Environ. Sci. Technol. , 34 : 1132 – 1142 .
- Lin , C. C. , Chen , S. J. and Huang , K. L. 2005 . Characteristics of Metals in Nano/Ultrafine/Fine/Coarse Particles Collected beside a Heavily Trafficked Road . Environ. Sci. Technol. , 39 : 8113 – 8122 .
- Lin , C. C. , Huang , K. L. , Chen , S. J. , Liu , S. C. , Tsai , J. H. , Lin , Y. C. and Lin , W. Y. 2009 . NH4 +, NO3 −, and SO4 2 − in Roadside and Rural Size-Resolved Particles and Transformation of NO2/SO2 to Nanoparticle-Bound NO3 −/SO4 2 − . Atmos. Environ. , 43 : 2731 – 2736 .
- Mader , B. T. and Pankow , J. F. 2001 . Gas/Solid Partitioning of Semivolatile Organic Compounds (SOCs) to Air Filters. 3. An Analysis of Gas Adsorption Artifacts in Measurements of Atmospheric SOCs and Organic Carbon (OC) when Using Teflon Membrane Filters and Quartz Fiber Filters . Environ. Sci. Technol. , 35 : 3422 – 3432 .
- Marple , V. A. , Robow , K. L. and Behm , S. M. 1991 . A Microorifice Uniform Deposit Impactor (MOUDI): Description, Calibration, and Use . Aerosol Sci. Technol. , 14 : 434 – 446 .
- Monaci , F. , Moni , F. , Lanciotti , E. , Grechi , D. and Bargagli , R. 2000 . Biomonitoring of Airborne Metals in Urban Environments: New Tracers of Vehicle Emission, in Place of Lead . Environ. Pollut. , 107 : 321 – 327 .
- Ntziachristos , L. , Ning , Z. , Geller , M. D. , Sheesley , R. J. , Schauer , J. J. and Sioutas , C. 2007 . Fine, Ultrafine and Nanoparticle Trace Element Compositions near a Major Freeway with a High Heavy-Duty Diesel Fraction . Atmos. Environ. , 41 : 5684 – 5696 .
- Oberdörster , G. , Oberdorster , E. and Oberdorster , J. 2005 . Nanotoxicology: An Emerging Discipline Evolving from Studies of Ultrafine Particles . Environ. Health Perspect. , 113 : 823 – 839 .
- Pak , S. S. , Liu , B. Y. H. and Rubow , K. L. 1992 . Effect of Coating Thickness on Particle Bounce in Inertial Impactor . Aerosol Sci. Technol. , 16 : 141 – 150 .
- Palmgren , F. , Wåhlin , P. , Kildesø , J. , Afshari , A. and Fogh , C. L. 2003 . Characterization of Particle Emissions from the Driving Car Fleet and the Contribution to Ambient and Indoor Particle Concentrations . Phys. Chem. Earth. , 28 : 327 – 334 .
- Phuleria , H. C. , Geller , M. D. , Fine , P. M. and Sioutas , C. 2006 . Size-Resolved Emissions of Organic Tracers from Light- and Heavy-Duty Vehicles Measured in a California Roadway Tunnel . Environ. Sci. Technol. , 40 : 4109 – 4118 .
- Phuleria , H. C. , Sheesley , R. J. , Schaauer , J. J. , Fine , P. M. and Sioutas , C. 2007 . Roadside Measurements of Size-Segregated Particulate Organic Compounds near Gasoline and Diesel-Dominated Freeways in Los Angeles, CA . Atmos. Environ. , 41 : 4653 – 4671 .
- Robert , M. A. , VanBergen , S. , Kleeman , M. J. and Jakober , C. A. 2007a . Size and Composition Distributions of Particulate Matter Emissions: Part 1—Light-Duty Gasoline Vehicles . J. Air & Waste Manage. Assoc. , 57 : 1414 – 1428 .
- Robert , M. A. , Kleeman , M. J. and Jakober , C. A. 2007b . Size and Composition Distributions of Particulate Matter Emissions: Part 2—Heavy-Duty Diesel Vehicles . J. Air & Waste Manage. Assoc. , 57 : 1429 – 1438 .
- Sardar , S. B. , Fine , P. M. , Mayo , P. R. and Sioutas , C. 2005 . Size-Fractionated Measurements of Ambient Ultrafine Particle Formation during Diesel Exhaust Dilution . Environ. Sci. Technol. , 39 : 932 – 944 .
- Schauer , J. J. , Kleeman , M. J. , Cass , G. R. and Simoneit , B. R. T. 1999 . Measurement of Emissions from Air Pollution Sources. 2. C1 through C30 Organic Compounds from Medium Duty Diesel Trucks . Environ. Sci. Technol. , 33 : 1578 – 1587 .
- Schauer , J. J. , Kleeman , M. J. , Cass , G. R. and Simoneit , B. R. T. 2002 . Measurement of Emissions from Air Pollution Sources. 5. C-1-C-32 Organic Compounds from Gasoline-Powered Motor Vehicles . Environ. Sci. Technol. , 36 : 1169 – 1180 .
- Schauer , J. J. , Rogge , W. F. , Hildemann , L. M. , Mazurek , M. A. and Cass , G. R. 1996 . Source Apportionment of Airborne Particulate Matter Using Organic Compounds as Tracers . Atmos. Environ , 30 : 3837 – 3855 .
- Spencer , M. T. , Shields , L. G. and Prather , K. A. 2007 . Simultaneous Measurements of the Effective Density and Chemical Composition of Ambient Aerosol Particles . Environ. Sci. Technol. , 41 : 1303 – 1309 .
- Subramanian , R. , Khlystov , A. Y. , Cabada , J. C. and Robinson , A. L. 2004 . Positive and Negative Artifacts in Particulate Organic Carbon Measurements with Denuded and Undenuded Sampler Configurations . Aerosol Sci. Technol. , 38 ( S1 ) : 27 – 48 .
- Turpin , B. J. , Saxena , P. and Andrews , E. 2000 . Measuring and Simulating Particulate Organics in the Atmosphere: Problems and Prospects . Atmos. Environ. , 34 : 2983 – 3013 .
- Turpin , B. J. and Lim , H.-J. 2001 . Species Contributions to PM2.5 Mass Concentrations: Revisiting Common Assumptions for Estimating Organic Mass . Aerosol Sci. Technol. , 35 : 602 – 610 .
- Wang , Y. F. , Huang , K. L. , Li , C. T. , Mi , H. H. , Luo , J. H. and Tsai , P. J. 2003 . Emissions of Fuel Metals Content from a Diesel Vehicle Engine . Atmos. Environ. , 37 : 4637 – 4643 .
- Weingartner , E. , Keller , C. , Stahel , W. A. , Burtscher , H. and Baltensperger , U. 1997 . Aerosol Emission in a Road Tunnel . Atmos. Environ. , 31 : 451 – 462 .
- Yang , H. H. , Hsieh , L. T. , Liu , H. C. and Mi , H. H. 2005 . Polycyclic Aromatic Hydrocarbon Emissions from Motorcycles . Atmos. Environ. , 39 : 17 – 25 .
- Zhu , Y. , Hinds , W. C. , Kim , S. H. , Shen , S. and Sioutas , C. 2002 . Study of Ultrafine Particles near a Major Highway with Heavy-Duty Diesel Traffic . Atmos. Environ. , 36 : 4323 – 4336 .