Abstract
The application of oxidative reactions of Oxone® [2KHSO5 KHSO4 K2SO4] as an active decontaminant has been mainly utilized through the aqueous solution. However, little is known about reactivity of aerosolized Oxone particles although the use of appropriate aerosolized oxidants would have significant value in remediating contaminated surfaces. In this study, the feasibility of producing active chlorine species from saline-Oxone aerosols was studied using a 2-m3 Teflon film chamber. To evaluate the oxidative capability of a saline-Oxone aerosol, the aerosol was collected onto filters impregnated with a dye (e.g., metanil yellow) that reacts with active chlorine in the aerosol, and monitored by UV-Visible spectroscopy. Spectral data showed that the dye compound was 86% oxidized by active chlorine within 4 min. There was no significant difference in oxidation capability between with buffer and without buffer system, although the presence of phosphate buffer somewhat retarded the formation rate of active chlorine in saline-Oxone aerosol particles. Saline-Oxone aerosols were found to be highly acidic as determined by UV-Vis spectroscopy, indicating that the aerosol produced mostly an undissociated form of active chlorine species (e.g., HOCl and Cl2) that was susceptible to partitioning into the gas phase. Our study concludes that the aerosolized saline-Oxone is a feasible chlorine oxidant, but the efficiency of such an approach still needs investigation.
1. INTRODUCTION
Oxone® [2KHSO5 KHSO4 K2SO4, molecular weight (MW) = 614.76 g/mol] is a triple salt compound containing the active oxidative reagent, potassium monopersulfate (KHSO– 5). Oxone is commonly used for a variety of industrial and consumer applications such as swimming pool shock oxidizer (CitationWojtowicz et al. 1983; CitationGay 1994), bleach component in denture cleanser (Eoga 1985), and laundry formulations (CitationEckhardt et al. 1989). Oxone has been utilized for efficient oxidation of organic compounds such as the conversion of alkenes to diols, sulfides to sulfoxides, and ketones to esters (CitationKennedy and Stock 1960; CitationWebb and Ruszkay 1998; CitationJiang 1989). CitationTravis et al. (2003) demonstrated the oxidation of aldehydes to carboxylic acids or ester products using Oxone aqueous solution. In addition, Oxone has been used to remove NO x and SO2 through aqueous absorption and oxidation in wet scrubbing processes (CitationAdewuyi and Owusu 2003; CitationMartin 2005). Synergetic reagents have been frequently used to enhance the oxidative capability of Oxone. For example, CitationSchulze et al. (2006) presented an efficient method for oxidizing alcohols using a mixture of sodium chloride (NaCl) and Oxone. CitationDelcomyn et al. (2006) and CitationWallace et al. (2005) showed that Oxone solutions buffered with sodium bicarbonate containing a chloride salt or acetone rapidly inactivated viruses, bacteria, and fungi and proposed that the resulting oxidant solutions appeared to be effective bleach substitutes.
In the presence of saline aqueous solution, Oxone produces active chlorine species such as hypochlorous acid (HOCl) and hypochlorite ion (OCl–) through the reaction between peroxymonosulfate ion (HSO– 5) in Oxone and chloride ion as shown in (CitationDelcomyn et al. 2006). The induced active chlorine species (HOCl and OCl–) are more powerful oxidants than Oxone alone (CitationDelcomyn et al. 2006). HOCl and OCl– are evenly distributed at pH 7.5 (25°C) (CitationFeng et al. 2007), so a buffer such as sodium bicarbonate has often been used to control the distribution of HOCl and OCl– in aqueous chemistry. shows the possible reactions between saline and Oxone in aerosol. Unlike in aqueous solution, the low water content in aerosol, which is controlled by ambient humidity, results in low pH and produces an undissociated form of active chlorine species such as HOCl and Cl2 rather than OCl– (CitationWang et al. 2007). Resulting HOCl and Cl2 can evaporate to the gas phase as shown in thereby reducing the available active chlorine species in the aerosol.
The application of oxidative reactions of Oxone has been mainly utilized through the aqueous solution. However, little is known about the water uptake properties and reactivity of Oxone aerosol particles suspended in ambient air. In general, the use of appropriate aerosolized oxidants would have significant value in remediating chemically or biologically contaminated surfaces. For example, oxidative aerosols can penetrate into contaminated interior spaces of structures and buildings where dissemination of liquid decontaminants would otherwise be difficult. The objectives of this study are (1) to investigate oxidative reactivity of active chlorine species from aerosolized saline-Oxone particles; (2) to understand the physical and chemical properties of the aerosolized saline-Oxone including particle acidity and humidity effects on particle water content and phase; and finally, (Equation3) to determine saline-Oxone aerosols’ potential value as a decontaminant.
FIG. 2 UV-Visible spectroscopy to monitor the oxidation capability of active chlorine and aerosol acidity in both internally and externally mixed aerosols. For externally mixed aerosol, Oxone particles were collected on the dyed filter containing the preexisting saline or saline-buffer particles. For the internally mixed aerosol, the saline-Oxone aerosol is sampled on the dyed filter using the different sampling systems (F1-F2-F3 and F1-D-F2).
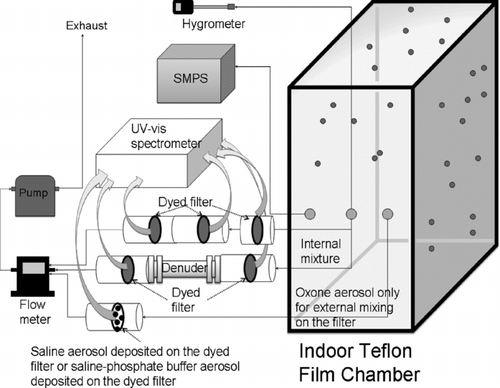
TABLE 1 Experimental conditions to study aerosol reactivity and acidity using UV-Vis spectroscopy for the aerosol sample collected on the dyed filter with metanil yellow or thymol blue a
2. EXPERIMENTAL SECTION
2.1. UV-Vis Spectroscopy to Monitor the Evolution of Active Chlorine in Saline-Oxone Aerosol
shows the experimental setup to monitor the reactivity of internally or externally mixed saline-Oxone aerosol using a 2-m3 Teflon film indoor chamber. Internally mixed saline-Oxone aerosols were produced by atomizing a single solution of saline and Oxone, while externally mixed aerosols were produced by separately atomizing and separately impacting Oxone and saline solutions. The chamber was flushed with air purified in house using two clean air generators (Aadco Model 737, Rockville, MD; Whatman Model 75-52, Haverhill, MA) prior to experiments. The humidity of the indoor chamber air was controlled by passing the clean dry air through a water bubbler. To study the oxidizing capability of saline-Oxone aerosol, metanil yellow (MY, Sigma Aldrich) dye which can react with active chlorine produced from saline-Oxone aerosol was used (CitationSleiman et al. 2007). A 16 mm sampling filter (Gelman Sciences Pallflex, Type TX40HI20-WW) was dyed prior to particle collection in a MY aqueous solution (1.98 mg/10 mL water) for 10 min and dried in room air (CitationJang et al. 2008).
For the study of the reaction in the internally mixed aerosol, the mixture (saline: Oxone weight ratio = 1:1) made of 1% saline (sea salt, Sigma-Aldrich) and 2% Oxone (Potassium monopersulfate triple salt, ⩾ 47% KHSO5 basis, Sigma-Aldrich) aqueous solutions was atomized into the indoor Teflon chamber using a medical nebulizer (LC STAR, Pari Respiratory Equipment, Inc., Midlothian, VA). The internally mixed saline-Oxone aerosol was sampled on two or three MY dyed filters in series with or without a three channel annular denuder (URG-2000): the filter-filter (F1-F2), or filter-filter-filter (F1-F2-F3), or filter-denuder-filter (F1-D-F2) system. The denuder was coated with K2CO3 to eliminate acidic gases such as HOCl. The sampling time was 2–3 min and the flow rate was 10–11 L/min.
For the study of reaction in the externally mixed aerosol, saline particles or saline particles buffered with phosphate (Sigma-Aldrich, 0.1 M) were directly impacted on MY-dyed filters using the nebulizer and weighed using an analytical balance (MX5 Mettler-Toledo Ltd., England) to measure the collected particle mass. Then Oxone solution (2% W/V) was atomized by a nebulizer into the Teflon chamber and the Oxone aerosol suspended in the chamber was collected on the dyed filter that contained the saline or the saline-buffer particles as described above using a pump (Gast, DOA-P704-AA) at 13 L/min for 2–3 min.
Aerosol particle size distribution and concentration were monitored with a scanning mobility particle sizer (SMPS) (TSI, Model 3080) coupled to a condensation nuclei counter (TSI, Model 3025A and Model 3022). The aerosol sampling flow rate was 0.3 L/min, and the sheath airflow rate was 2 L/min. Oxidation of MY-dyed filters from either internally or externally mixed saline-Oxone particles was measured by reflectance-UV-Visible spectroscopy (Lambda 35, Perkin Elmer). The slit width and wavelength interval of spectral data were each 1 nm with a UV-Vis spectrum scan from 280 to 800 nm.
2.2. UV-Vis Spectroscopy to Monitor the Acidity of Externally Mixed Saline-Oxone Aerosol
Acidity of externally mixed saline-Oxone aerosols was measured by collecting particles on a thymol blue (TB) (Sigma Aldrich) dyed filter and analyzing with reflective UV-Visible spectroscopy (CitationJang et al. 2008). The filters were dyed with a TB solution prepared by adding 2 mg of TB dye to a mixture of 5 mL water and 5 mL ethanol. The experimental conditions and resulting data using UV-Vis spectroscopy are shown in .
2.3. Measurement of Water Amount in Saline-Oxone Particles using FTIR
shows the FTIR setup (Nicolet Magma 560, Nicolet) to monitor water content in particles collected on a silicon window. Sodium chloride (Fisher, 99.5%), saline, Oxone, and saline-Oxone (1:2 weight ratio) with or without buffer solutions were atomized using a medical nebulizer. Sodium bicarbonate (Sigma-Aldrich, 99.7%) and phosphate (Sigma-Aldrich, 0.1 M) were used as buffers. The resulting NaCl, saline, Oxone, and saline-Oxone particles were collected on a silicon FTIR window (13 × 2 mm, Sigma Aldrich) by impaction, dried directly on the window with clean air (Breathing air, Airgas), and weighed using an analytical balance before and after particle collection to measure the particle mass. FTIR spectra of the impacted particles were obtained in the absorption mode varying relative humidity (RH) from 20% to 90% with the particle face of the silicon window exposed to the air in the small flow chamber (0030-104, Thermo Spectra-Tech). The RH inside the flow chamber was controlled by combining humid air from a water bubbler and dry air from a dry air tank (Breathing air, Airgas) with a total air flow rate from 0.3 to 1.0 L/min. Humidity and temperature were measured with an electronic thermohygrometer (Dwyer series 485). shows the experimental conditions and results for the FTIR studies.
3. RESULTS AND DISCUSSION
3.1. Active Chlorine Formation and its Oxidizing Effect
To evaluate the feasibility of oxidative reactions on a surface initiated by impacted Oxone particles, the formation of active chlorine (e.g., HOCl and Cl2) in the oxidant aerosol was studied. Internally or externally mixed oxidant aerosol was collected on the MY-dyed filter and monitored with reflective UV-Visible spectroscopy (CitationJang et al. 2008). Unfortunately, MY exhibits spectral changes due to both reaction with active chlorine (CitationSleiman et al. 2007) and change in proton concentrations ([H+], M). The spectral changes due to [H+] can be separated because UV absorption maxima of unprotonated-MY and protonated-MY (HMY+) appear at 420 and 550 nm, respectively. The absorption cross-section ratio for MY and HMY+ was determined by measuring spectral shifts when acidic inorganic particles composed of sulfuric acid and ammonium hydrogen sulfate were collected on a MY-dyed filter. The acidic inorganic particle caused only spectral shifts as a result of [H+] changes. It is important to note that the absorption at 550 nm was negligible before collection of acidic particles (i.e., MY was initially not protonated). Under the conditions of these experiments, a cross-section ratio (σ420/σ550) of 0.21 was measured using a least-squares curve fitting method described previously (CitationJang et al. 2008). The absorption spectrum was fit to two Gaussian functions with peak center frequency, peak absorbance, and peak width as adjustable parameters.
is the UV-Visible absorbance of the MY-dyed filter at 420 nm before collection of particles, and A
420,exp
is that at 420 nm after collection of particles (acids and oxidants). The difference between
and A
420,exp
can be decoupled into acidity effect and oxidation effect (ΔA
420,oxi
) as shown in Equation (Equation1) below.
ΔA
420,oxi
is normalized to the available dry Oxone aerosol mass ( that is estimated from the particle composition, the total aerosol mass (), and the aerosol water content (see section “Humidity effect”) at a given experimental RH condition. Figures and show the ΔA′420,oxi
values for the internally and externally mixed oxidant aerosols, respectively. The gas-particle partitioning of active chlorine (e.g., HOCl and Cl2) generated in the internally mixed saline-Oxone aerosol particles was investigated using the F1-F2-F3 and F1-D-F2 sampling setups (see ). The reaction time for the internally mixed saline-Oxone aerosol (x-axis in ) is the total elapsed time from solution mixing to UV scanning (solution mixing: 30–45 s, aerosol atomizing: 30–50 s, stabilizing of aerosol: 9–50 min, aerosol sampling: 2–3 min, UV scanning: 1 min 40 s). The ΔA′420,oxi
values for the F1 filters for both sampling setups (closed symbols) were the same within the uncertainty of the measurement. Additionally, there was no significant time dependence of the ΔA′420,oxi
values for the F1 filters indicating that the reaction between saline and Oxone was complete within 10 min and the oxidant concentration was stable for at least 50 min. Such tendency was reconfirmed by the additional three data sets using the F1–F2 sampling system.
The oxidation of MY in the F1 filter can be caused by both gas- and condensed-phase active chlorine (e.g., HOCl and Cl2). To estimate the fraction of MY oxidized by gas-phase active chlorine in the F1 filter, F2 and F3 filters were analyzed in the two different sampling setups. F2 in the F1-D-F2 sampling set-up exhibited negligible ΔA′420,oxi values indicating that oxidation on downstream filters without the denuder is caused primarily by gas-phase oxidants because the K2CO3 coated denuder removes acidic gases (e.g., HOCl) and also possibly gas phase Cl2. Together with the F2 and F3 results without the denuder, this conclusively demonstrates that some of the oxidation on the F1 filter is caused by gas-phase oxidants. To estimate the fractional amount of oxidation on F1 from gas-phase active chlorine, the F2 and F3 filters were examined using the F1-F2-F3 system. As shown in , ΔA′420,oxi values for both F2 and F3 without the denuder are equivalent within the uncertainty of the measurements. This indicates that the depletion of gas-phase active chlorine across the filters was negligible; otherwise, the oxidation of F3 would be significantly less than F2. We conclude that the gas-phase active chlorine exposure of F1 is equivalent to that of F2 and F3. The observed oxidation of F2 and F3 was 30% of that for F1, and because the gas-phase active chlorine exposure was the same for all three filters, gas-phase active chlorine oxidation of F1 accounts for 30% of the total oxidation at our sampling and experimental conditions. Conversely, particle-bound oxidant accounts for 70% of the observed oxidation of MY on the filter.
TABLE 2 Experimental conditions to monitor the water content in saline, Oxone, and saline-Oxone particles using FTIR and the estimated water amount in various particles in the two different RH levels at 20% and 70%
FIG. 4 The ΔA’420,oxi (Equation (2)) obtained using different sampling systems (F1-F2-F3 and F1-D-F2) for the internally mixed saline-Oxone aerosol after the Oxone was mixed with saline in the aqueous solution. Error bars in F1 filters are estimated from uncertainties in the SMPS data (6%), and UV-Visible absorbance (4%) and sampling flow rate (1%). Error bars in F2 and F3 filters which evaluate oxidation effects of gas-phase oxidants are estimated from uncertainties in the sampling flow rate and UV-Visible absorbance.
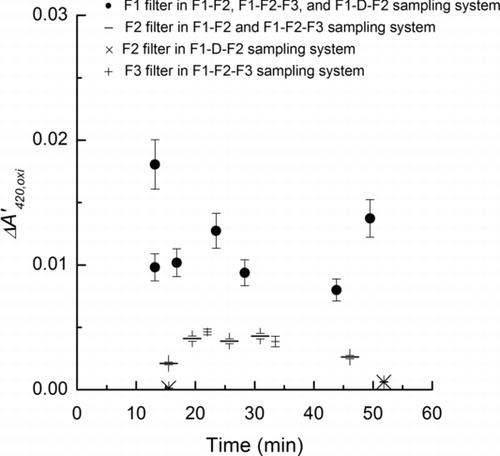
FIG. 5 Time profile of ΔA’420,oxi (Equation (2)) of the external mixture of Oxone, saline-Oxone, saline-Oxone with phosphate buffer aerosol. Error bars were estimated from uncertainties in the sampling device, balance, SMPS data, and UV-Visible absorbance.
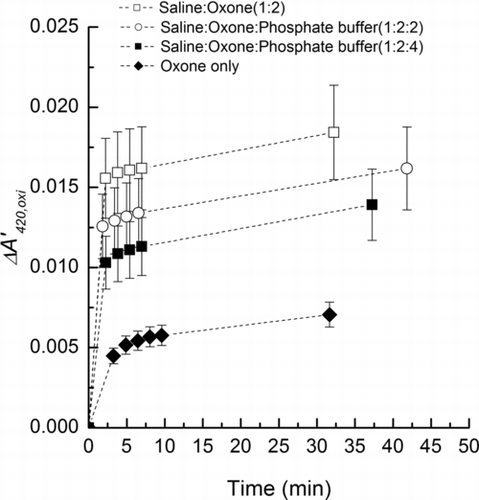
For the externally mixed particles, active chlorine formed when the Oxone particles contacted either saline or saline-phosphate buffer particles on the dyed filter. Hence, both the reaction time and loss of active chlorine via off-gassing can be considerably reduced in the externally mixed saline-Oxone aerosol compared to those in the internally mixed aerosol. shows the ΔA′420,oxi values obtained using Equations (Equation1) and (2) where the x-axis is the time after collection of the Oxone particles. Overall, higher MY oxidation was observed for Oxone particles collected in the presence of saline because saline promoted the formation of active chlorine species. However, even Oxone particles collected in the absence of saline showed some oxidizing capability. For example, the ΔA′420,oxi value of the Oxone aerosol 30 min after the reaction began was 38–51% of that for the co-deposited Oxone and saline or Oxone and saline-phosphate buffer particles. The highest oxidation was observed for the co-collected saline and Oxone particles. After the Oxone aerosol was collected on the dyed filter containing the preexisting saline, nearly 86% of the oxidation progressed within 4 min compared to oxidation within 33 min for our experimental duration. This result confirms that the formation of active chlorine from the reaction between saline and Oxone rapidly progresses. In the presence of phosphate buffer, oxidation in saline-Oxone aerosol somewhat slowly progressed. Statistical significance appears only between saline-Oxone aerosol and highly buffered saline Oxone (Saline: Phosphate = 1:4) aerosol. This result suggests that buffer system is not as efficient to retard the production rate of active chlorine in aerosol as in aqueous solution.
3.2. Buffer Stability in Saline-Oxone Aerosol
Commonly used buffers such as bicarbonate and phosphate were internally mixed with oxidant particles and monitored using FTIR to evaluate chemical stability in oxidant aerosols. shows the FTIR spectra of each buffer compared to the subtracted spectra ([spectrum of the saline-Oxone-buffer particles] − [spectrum of saline-Oxone particles]). The subtracted FTIR spectrum for the particle system containing phosphate buffer resembles the finger print of phosphate buffer alone, confirming the presence of buffer in the saline-Oxone particles. However, the subtracted FTIR spectra for saline-Oxone particles buffered with sodium bicarbonate does not show characteristic peaks at 700, 830, 1000, and 1300–1400 cm–1 for bicarbonate buffer (). This result indicates that particles buffered with bicarbonate, while potentially at the correct pH, would lose their buffering capacity since carbonic acid produced from the bicarbonate buffer rapidly decomposes into carbon dioxide in the presence of water as shown in Equations (Equation3) and (4) (CitationThomas et al. 2000).
Therefore, the stability of phosphate buffer makes it more favorable for incorporation into the saline-Oxone aerosol.
3.3. Humidity Effect
Unlike oxidation in aqueous solution, humidity influences kinetics of aerosol-phase reactions through two possible mechanisms. First, the aerosol-phase water content, which is directly related to the humidity, affects the reaction of saline-Oxone aerosol through phase transitions in the particles. Second, the enrichment of inorganic constituents in particles due to the low water content in aerosol can influence kinetics of formation of active chlorine and/or thermodynamic equilibria of inorganic species.
To monitor the humidity effect on saline-Oxone aerosol, FTIR integrated with a small flow chamber capable of maintaining RH between 20% and 90% was used (). The intensity of the broad OH stretching (centered at 3350 cm–1) and OH bending (centered at 1650 cm–1) bands originating from the condensed-phase water in the impacted particles increases with increasing RH (Criczo and Abbatt 2000; CitationKreidenweis et al. 2008). Water uptake into NaCl particles has been studied previously, so it provides a convenient reference for these measurements of particles impacted on a silicon window. In our study, the observed deliquescent RH (DRH) for the impacted NaCl particles was 83–87% and the efflorescent RH (ERH) was 55–57%. The resulting DRH and ERH values for the NaCl particles of this study were slightly higher than those known in the literature (75–80% for DRH and 40–50% for ERH) (CitationGao et al. 2007). Calibration curves for water content (Mwater
, μg) in the impacted NaCl particles were obtained by relating FTIR absorbance (Abs
and Abs
of the water peak (3350 cm–1 and 1650 cm–1) at different RH and the water mass fraction predicted from an inorganic thermodynamic model (CitationNenes et al. 1998). M′
water
(μg/μg) represents the particle-phase water mass [Mwater
(μg)] normalized to the dry-particle mass (M
dry−particle
) that is estimated from the difference between the total particle mass and the water mass at a given laboratory RH and a given aerosol particle sample:
FIG. 7 The M′ water (Equations (Equation5) and (6), μg/μg) of NaCl, saline, Oxone, and saline-Oxone aerosols as a function of decreasing humidity. Error bars were estimated from uncertainties in the FTIR absorbance at O-H band and balance.
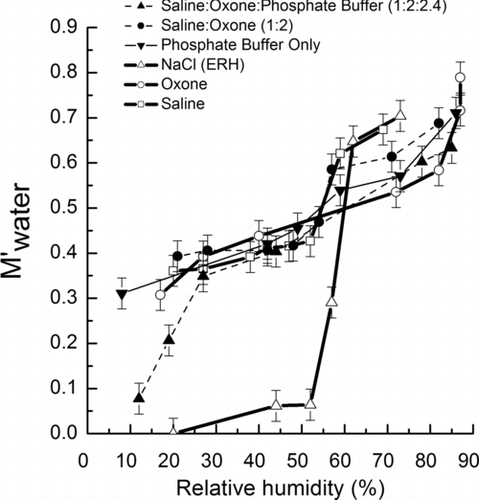
and show the Mwater (μg) and M′ water (μg/μg) obtained for the various particle compositions. In this study, M′ water for saline and NaCl particles was estimated from Equation (Equation5) and also compared to that obtained using Equation (Equation6) to ensure of the use of FTIR peaks at 1650 cm–1, which give relatively weaker peak intensity than one at 3350 cm–1. When the aerosol includes inorganic species (e.g., HOSO– 3, HOSO– 4, and HOPO–2 3) that contain an OH stretching band other than water at 3350 cm–1, Equation (Equation6) was employed to estimate M′ water since the FTIR peak at 1650 cm–1 is unique for water (OH bending motion). In , both impacted NaCl particles and impacted saline particles show step changes in the water mass fraction indicating a phase transition (ERH ∼ 55%). However, no dry aerosol was observed for the saline particles which showed a large water mass fraction (M′ water ∼ 0.35) in the particles even at 20% RH compared with NaCl particles which showed an insignificant water mass fraction. This difference is mainly caused by MgCl2 and MgSO4 present in saline particles (CitationTang et al. 1997; CitationZhao et al. 2006). MgSO4 promotes gel formation in concentrated liquid solutions at low RH preventing crystallization of the particles. The saline-Oxone aerosol appeared to be similar to saline aerosol with weak ERH (∼55%). In general, NaCl can react with H2SO4 forming NaHSO4, while no evidence is observed for the reaction of MHSO4 (e.g., M: Na+ or K+) with NaCl (CitationZangmeister and Permberton 2000). The calculated mole ratio of Cl– to HSO− 5 in the internally mixed saline-Oxone aerosol is 4.8 at a given initial composition indicating that the major aerosol constituent is still saline, although potassium, sodium sulfates and bisulfate as constituents of Oxone are present in aerosol and the sodium ion as a byproduct after evolution of acidic gas (e.g., HOCl) () can titrate bisulfate. It is also reasonable to conclude that the aerosol is not crystallized due to gel-like saline constituents.
Either phosphate buffer salt as a mixture of NaH2PO4 and Na2HPO4 or Oxone as triple salts consisting of 2KHSO5, KHSO4, and K2SO4, are hygroscopic and show no apparent phase transition as RH was reduced to 20% (). Saline-Oxone particles with phosphate buffer also show no ERH at 55% RH. The saline cannot be a major aerosol constituent in saline-Oxone particles with phosphate buffer due to the presence of the high fraction of phosphate salts. Instead, its water mass fraction significantly drops at 19% as an ERH. The aerosol phase reaction combining with evolution of an acidic gas can produce a variety of additional salts (e.g., M2HPO4, M2SO4, and MgHPO4) in saline-Oxone particles with phosphate buffer and would change the hygroscopic property of the aerosol. It is known that the MgHPO4 is slightly soluble in water and often used for production of less hygroscopic fertilizer (CitationKarmyshov et al. 1974). Thus the amount of hydrated MgHPO4 (commonly tri-hydrated) (CitationBensalem and Iyer 1995) would be limited compared to MgSO4 (commonly hepta-hydrated) in saline, and this would modify the hygroscopic property of gel-like saline constituent in the presence of phosphate buffer salt.
In fact, all impacted oxidant particles have significant water mass fractions suggesting that oxidant aerosols are liquid and active chlorine species can form in saline-Oxone aerosol over the humidity range (20–85%) relevant to ambient conditions. summarizes possible major aerosol constituents from the saline-Oxone aerosol with and without buffer.
3.4. Dynamics of Aerosol Acidity
Acidity influences the distribution of aqueous-phase chemical species (e.g., SO−2
5 vs. HSO−
5 and OCl− vs. HOCl) which can affect the oxidizing capability of the resulting aerosol. The proton concentration, [H+] (M), of saline-Oxone aerosol was determined from proton mass (, ng/m3) measured by UV-Vis spectroscopy (CitationJang et al. 2008) and Mwater
measured by FTIR. To estimate the
in saline-Oxone aerosol, thymol blue (TB) dye was selected as a pH indicator (CitationSaikia and Dutta 2006) since it is insensitive to active chlorine oxidants. A color change from yellow (λ= 450 nm) to red (λ= 550 nm) occurs near pH 1.2–2.8 due to protonation of the TB dye (HTB+). The
of the aerosol filter sample was calculated using the sample air volume (Vair
), UV-Visible absorbance [
] at 550 nm for the protonated TB, and a calibration curve for aerosol acidity. The calibration curve (Equation (Equation7)) was obtained by relating the known
of inorganic acidic aerosol particles (NH4HSO4) (CitationJang et al. 2008) with A
550,aerosol
corrected for spectral overlap from the absorbance of unprotonated TB.
calculated from Equation (Equation7) for the saline-Oxone aerosol (1:2 weight ratio), saline-Oxone-phosphate buffer (1:2:2 weight ratio) aerosol and Oxone only aerosol are shown in . The [H+] of the saline-Oxone, saline-Oxone with phosphate buffer and the Oxone only aerosols were obtained from
(Equation (Equation7)) and M
water (Equation (6)):


4. CONCLUSIONS
Our results from the study of the oxidizing capability of aerosol-phase active chlorine species towards the organic dye show that the aerosolized saline-Oxone is a feasible decontaminant for surfaces. The production rate of active chlorine species varies with formulation of the oxidizing aerosol (i.e., presence and type of buffer) and aerosol mixing types (e.g., internally or externally mixed aerosol). For example, when the fast oxidation is required in the application such as examples shown by CitationDelcomyn et al. (2006), the externally mixed saline and Oxone particles without phosphate buffer would be most profitable () among oxidant aerosols formulations investigated here. The externally mixed saline-Oxone with phosphate buffer was found to slowly form active chlorine in aerosol, but an insignificant difference was observed in oxidation capability with and without buffer systems.
Overall, one significant problem with this approach is the partitioning of the aerosol-originated oxidant to the gas phase. The low pH observed in the particles favors the formation of HOCl and Cl2 which evaporate from the particles. Future research needs to quantify the gas−particle partitioning of active chlorine and investigate formulations that result in neutral pH.
This work was supported by the Defense Threat Reduction Agency and the Air Force Research Laboratory (Project: 080623: FA8650-08-1-5916) and the National Science Foundation (ATM-0757443 and ATM 0852747).
REFERENCES
- Adewuyi , Y. G. and Owusu , S. O. 2003 . Aqueous Absorption and Oxidation of Nitric Oxide with Oxone for the Treatment of Tail Gases: Process Feasibility, Stoichiometry, Reaction Pathways, and Absorption Rate. . Ind. Eng. Chem. Res. , 42 : 4084 – 4100 .
- Bensalem , A. and Iyer , G. 1995 . Ambient Pressure and Temperature Synthesis of New Layered Magnesium Phosphate: MgHPO4. 0. 78H2O. . J. Solid State Chem. , 114 : 598 – 600 .
- Cziczo , D. J. and Abbatt , J. P. D. 2000 . Infrared Observations of the Response of NaCl, MgCl2, NH4HSO4, and NH4NO3 Aerosols to Changes in Relative Humidity from 298 to 238 K. . J. Phys. Chem. A , 104 : 2038 – 2047 .
- Delcomyn , C. A. , Bushway , K. E. and Henley , M. V. 2006 . Inactivation of Biological Agents using Neutral Oxone–Chloride Solutions. . Environ. Sci. Technol. , 40 : 2759 – 2764 .
- Eckhardt , C. , Hefti , H. , Meyer , H. R. and Weber , K. 1989 . Stable Detergents Containing Optical Brighteners and Bleaching Agents. . Eur. Pat. Appl. , : 7
- Eoga Anthony , B. J. 1985 . Monopersulfate-containing Denture Cleansers . Eur. Pat. Appl. , : 20
- Feng , Y. , Smith , D. W. and Bolton , J. R. 2007 . Photolysis of Aqueous Free Chlorine Species (HOCl and OCl–) with 254 nm Ultraviolet Light. . J. Environ. Eng. Sci. , 6 : 277 – 284 .
- Gao , Y. , Chen , S. B. and Yu , L. E. 2007 . Efflorescence Relative Humidity of Airborne Sodium Chloride Particles: A Theoretical Investigation. . Atmos. Environ. , 41 : 2019 – 2023 .
- Gay , W. A. 1994 . Sanitizer for Swimming Pools, Spas, and Hot Tubs . Cont. –in–part of U. S. 5,258,409 , : 9
- Gershenzon , M. , Davidovits , P. , Jayne , J. T. , Kolb , C. E. and Worsnop , D. R. 2002 . Rate Constant for the Reaction of Cl2(aq) with OH–. . J. Phys. Chem. A , 106 : 7748 – 7754 .
- Jang , M. , Cao , G. and Paul , J. 2008 . Colorimetric Particle Acidity Analysis of Secondary Organic Aerosol Coating on Submicron Acidic Aerosols . Aerosol Sci. Technol. , 42 : 409 – 420 .
- Jiang , Z. 1989 . New Oxidation Method for Aldehydes to Carboxylic Acids by Oxone-Acetone System. . Zhongguo Yiyao Gongye Zazhi , 20 : 529 – 530 .
- Karmyshov , V. F. , Vasileva , L. V. , Perminova , L. Ya. and Abramov , I. E. 1974 . Preparation of Granulated Ammoniated Superphosphate from Kara-Tau Phosphorites. . USSR. Khimicheskaya Promyshlennost (Moscow, Russian Federation) , 9 : 669 – 671 .
- Kennedy , R. J. and Stock , A. M. 1960 . Oxidation of Organic Substances by Potassium Peroxymonosulfate. . J. Org. Chem. , 25 : 1901 – 1906 .
- Kreidenweis , S. M. , Petters , M. D. and DeMott , P. J. 2008 . Single-Parameter Estimates of Aerosol Water Content. . Environ. Res. Lett. , 3 : 7
- Martin , P. 2005 . A Composition including Potassium Monopersulfate and Halogen Sepd. by a Barrier Film for Water Treatment in Aquatic Facilities. . PCT Int. Appl. , : 16
- Nenes , A. , Pandis , S. N. and Pilinis , C. 1998 . ISOPROPIA, A New Thermodynamic Equilibrium Model for Multiphase Multicomponent Inorganic Aerosols . Aquatic Geochemistry , 4 : 123 – 152 .
- Saikia , P. M. and Dutta , R. K. 2006 . Acid-base Equilibria of Sulfonephthalein Dyes in Aqueous Polymer-surfactant Media. . J. Surfactants and Detergents , 9 : 39 – 45 .
- Schulze , A. , Pagona , G. and Giannis , A. 2006 . Oxone/Sodium Chloride. A Simple and Efficient Catalytic System for the Oxidation of Alcohols to Symmetric Esters and Ketones. . Synth. Commun. , 36 : 1147 – 1156 .
- Sleiman , M. , Vildozo , D. , Ferronato , C. and Chovelon , J. M. 2007 . Photocatalytic Degradation of Azo Dye Metanil Yellow: Optimization and Kinetic Modeling using a Chemometric Approach. . Applied Catalysis, B: Environmental , 77 : 1 – 11 .
- Tang , I. N. , Tridico , A. C. and Fung , K. H. 1997 . Thermodynamic and Optical Properties of Sea Salt Aerosols. . J. Geophys. Res. , 102 : 23269 – 23275 .
- Thomas , L. , Christofer , T. , Romano , T. K. , Ingrid , K. , Andreas , H. , Erwin , M. and Klaus , R. L. 2000 . On the Surprising Kinetic Stability of Carbonic Acid (H2CO3). . Angew. Chem. Int. Ed. , 5 : 892 – 894 .
- Travis , B. R. , Sivakumar , M. , Hollist , G. O. and Borhan , B. 2003 . Facile Oxidation of Aldehydes to Acids and Esters with Oxone. . Organic Letters , 25 : 1031 – 1034 .
- Wallace , W. H. , Bushway , K. E. , Miller , S. D. , Delcomyn , C. A. , Renard , J. J. and Henley , M. V. 2005 . Use of In Situ–Generated Dimethyldioxirane for Inactivation of Biological Agents. . Environ. Sci. Technol. , 39 : 6288 – 6292 .
- Wang , L. , Bassiri , M. , Najafi , R. , Najafi , K. , Yang , J. , Khosrovi , B. , Hwong , W. , Barati , E. , Belisle , B. , Celeri , C. and Robson , MC . 2007 . Hypochlorous Acid as a Potential Wound Care Agent. Part I. Stabilized Hypochlorous Acid: A Component of the Inorganic Armamentarium of Innate Immunity. . J. Burns & Wounds , 6 : 65 – 79 .
- Webb , K. S. and Ruszkay , S. J. 1998 . Oxidation of Aldehydes with Oxone in Aqueous Acetone. . Tetrahedron , 54 : 401 – 410 .
- Wojtowicz , J. A. , Faust , J. P. and Brigano , F. A. 1983 . Water Treatment of Swimming Pools, Spas, and Hot Tubs. . Kirk–Othmer Encycl. Chem. Technol. , 24 : 427 – 441 .
- Zangmeister , C. D. and Pemberton , J. E. 2000 . Raman Spectroscopy and Atomic Force Microscopy of the Reaction of Sulfuric Acid with Sodium Chloride. . J. Am. Chem. Soc. , 122 : 12289 – 12296 .
- Zhao , L. J. , Zhang , Y. H. , Wei , Z. F. , Cheng , H. and Li , X. H. 2006 . Magnesium Sulfate Aerosols Studied by FTIR Spectroscopy: Hygroscopic Properties, Supersaturated Structures, and Implications for Seawater Aerosols. . J. Phys. Chem. A , 110 : 951 – 958 .