Abstract
Single particle mass spectrometers have traditionally been deployed to measure the size and composition of individual particles. The relatively slow sampling rates of these instruments are determined by the rate at which the ionization lasers can fire and/or mass spectra can be recorded. Under most conditions, our single particle mass spectrometer, SPLAT, can detect and size particles at much higher rates than it can record mass spectra. We therefore developed a dual data acquisition mode, in which particle number concentrations, size distributions, and asphericity are measured at a rate determined by particle concentration and the particle detection efficiency, all while the instrument generates and records individual particle sizes and mass spectra at an operator-set rate. Particle number concentrations are calculated from the particle detection rate at the first optical stage and the measured sampling flow rate. We show that SPLAT measured particle number concentrations are in very good agreement with independent measurements by the passive cavity aerosol spectrometer probe (PCASP). Particle asphericity is based on the ratio of the particle detection rates at the first and second optical stages. Particle size is based on the measurement of particle time of flight between the two detection stages. We illustrate the artifact in the measured size distributions that can be introduced by high particle concentrations and present a method to remove it and correct the size distributions. Particle number concentration and asphericity are measured with 1 s resolution and particle vacuum aerodynamic size distributions are measured with 4 to 60 s resolution.
INTRODUCTION
Atmospheric aerosols affect climate, air quality, and health. Properly representing aerosols in models requires knowledge of their physical and chemical properties. Field measurements are designed to characterize particle concentrations, size distributions, chemical compositions, optical properties, shapes, densities, and activities as cloud condensation nuclei (CCN). These field data are an essential element required to guide model development and test their performance. However, to measure each of these properties typically necessitates a large number of instruments that require significant resources. The final product of this traditional approach is a multitude of independent measurements by different instruments that need to be put together to yield a cohesive coherent picture of the atmospheric aerosol life cycle.
It would clearly be advantageous to develop analytical tools that simultaneously measure several aerosol attributes for the same aerosol population or even individual particles, thereby also directly mapping the relationships between particle properties. To this end, most Single Particle Mass Spectrometers (SPMS) measure the size and the chemical composition of individual aerosol particles, which are directly related to aerosol optical properties and CCN and ice nucleation (IN) activity. SPMS are now an essential component of many field campaigns, including those conducted on aircraft (McFarquhar et al. 2010; Pratt et al. Citation2009; Thomson et al. Citation2000; Zelenyuk et al. Citation2009a). Recent developments of SPMS aim to extend the capabilities of SPMS beyond size and composition to measure density, hygroscopicity, optical properties, shape, fractal dimension, and number concentrations (Buzorius et al. 2002; Herich et al. 2009; Moffet and Prather Citation2005; Murphy et al. Citation2004; Zelenyuk et al. Citation2006; Zelenyuk and Imre Citation2009; Zelenyuk et al. Citation2008a; Zelenyuk et al. Citation2008b). Moffet and Prather developed the procedure for the determination of particle index of refraction and density from the measurements of the intensity of light scattered by particles as they are detected in their SPMS (ATOFMS) (Moffet and Prather Citation2005). In another SPMS (PALMS) the light scattering intensity is used to size the particles and deduce its density using additional, simultaneous measurement of particle vacuum aerodynamic diameter (dva ) (Murphy et al. Citation2004). Increase in SPMS sensitivity made it possible to combine SPMS with a Differential Mobility Analyzer (DMA) or a Tandem DMA (TDMA) to measure individual particle size, composition, density (Zelenyuk et al. Citation2008c), effective density (Spencer et al. Citation2007; Zelenyuk et al. Citation2006; Zelenyuk and Imre Citation2007; Zelenyuk et al. Citation2007, Citation2008a, Citation2008b), dynamic shape factors (Zelenyuk et al. Citation2006, Citation2008b), and provide information on particle asphericity (Zelenyuk et al. Citation2008c), asymmetry (Zelenyuk and Imre Citation2007), morphology (Carson et al. Citation1997; Vaden et al. Citation2010; Woods et al. Citation2002; Zelenyuk et al. Citation2008b), and hygroscopic growth factor (Buzorius et al. 2002; Herich et al. 2009; Zelenyuk et al. Citation2007, Citation2008a). The majority of the above listed examples of multidimensional characterization were accomplished using our single particle mass spectrometer, SPLAT II (for simplicity, just SPLAT (Zelenyuk and Imre Citation2005; Zelenyuk et al. Citation2009a)) and are described in detail elsewhere (Zelenyuk and Imre Citation2009) and references therein.
This article is the first in a two-part series that present recent developments of SPLAT that make it possible to run this instrument in two simultaneous modes and collect information on particle number concentrations and asphericity with 1 s resolution, and density and size distributions with 10 to 60 s resolution. Moreover, all of this is accomplished without having to interrupt SPLAT's routine measurements of single particle size and chemical composition, and without the need to combine SPLAT with other instruments, e.g., a DMA. The high temporal resolution this approach yields is especially valuable on mobile platforms like aircraft, where high temporal resolution and low payload are critical. In this article, we describe how particle number concentrations, size distributions, and asphericity are measured with high temporal resolution in real-time, and in the second article, we present the method to determine aerosol density based on measurements of the size distribution. The approaches described here are applicable to all single particle mass spectrometers that use an aerodynamic lens inlet and two stages of optical light scattering for particle detection and sizing. The specific performance parameters will depend on the size dependent detection probabilities of individual instruments.
EXPERIMENTAL
Particle Generation
The laboratory-generated particles presented in this paper were generated with an atomizer (TSI Inc., Model 3076), by aerosolizing the corresponding suspensions, neat compounds, and solutions. To avoid effects of surfactants and agglomeration, polystyrene latex (PSL) beads (Duke Scientific) were generated from water suspensions, whose volume fractions were lower than 10–5. Arizona Test Dust (ATD) particles were also generated by aerosolizing ATD water suspensions. NaCl (Sigma-Aldrich, >99.5% purity) and NaNO3 (SN) (Fisher Scientific, >99.5% purity) were dissolved in ultrapure deionized water and pyrene (Sigma-Aldrich, >98% purity) was dissolved in methanol (Sigma-Aldrich, 99.8% purity). These solutions were then aerosolized and all particle types were dried using two diffusion driers (TSI Inc., Model 3062) connected in series. Dioctyl phthalate (DOP) (Sigma-Aldrich, 99% purity) was aerosolized neat. Secondary organic aerosol (SOA) particles were formed by ozonolysis of α-pinene in a ∼100 L Teflon bag at low relative humidity (RH < 1%). The reaction mixture included α-pinene, ozone, and cyclohexane at concentrations of 200 ppb, 500 ppb, and 250 ppm respectively. Soot particles were directly sampled from a 1.9 L single-cylinder direct-injection spark ignition gasoline engine (General Motors). In some laboratory experiments presented here a DMA (TSI Inc., Model 3081) was used to classify particles with narrow mobility size distributions prior to their characterization by SPLAT.
Field data presented here were obtained by SPLAT during two field campaigns: the Indirect and Semi-Direct Aerosol Campaign (ISDAC) and the Carbonaceous Aerosols and Radiative Effects Study (CARES). During ISDAC, SPLAT was deployed onboard the Canadian National Research Council Convair 580 aircraft on the North Slope of Alaska (McFarquhar et al. 2010; Zelenyuk et al. Citation2009a) and in CARES, it was deployed in a ground sampling site in Sacramento, CA during June 2010.
Single Particle Mass Spectrometer
SPLAT has been described in detail elsewhere (Zelenyuk and Imre Citation2009; Zelenyuk et al. Citation2009a). Particles enter the aerodynamic lens inlet through a 100 μm flow-calibrated critical orifice. The aerodynamic lens forms a low divergence particle beam (Liu et al. Citation1995a, Citation1995b; Wang and McMurry Citation2006) and allows the transport of particles from the ambient air into the vacuum system with extremely high efficiencies (Zelenyuk et al. 2009a). It is important to keep in mind that beam divergence depends on particle shape and particle size. As we show below, it is possible to use this fact to characterize particle asphericity in real-time, with 1 s resolution. For each particle, transport through the lens imparts a velocity that is a narrow function of its vacuum aerodynamic diameter (dva ). After particles pass through the aerodynamic lens inlet and the two differentially pumped sections, they enter the main, high-vacuum section, where they are detected by light scattering in two optical detection stages, marked as ODS1 and ODS2 in . Each optical detection stage consists of a 300 mW, frequency-doubled diode-pumped continuous wave (cw) Nd:YAG laser (CrystaLaser, Model GCL-300-L, ∼330 μm focal spot), a large ellipsoidal reflector (Opti-Forms, Model E102-1, F# 0.91) and a photomultiplier counting head (PMT) (Hamamatsu, Model H6180-01). The PMT signals are continuously analyzed by counting the number of photons that are detected over a time window, which is set by the operator in the present work to 2 μs. The number of detected photons per 2 μs is compared to an operator-determined threshold. When the number of photons exceeds the threshold, the particle is detected and a clock is started. The same particle then proceeds to the 2nd optical detection stage located 10.5 cm downstream from the 1st stage, where the process is repeated. If the threshold condition is met in the 2nd stage as well, the particle time of flight (PTOF) between the two detection stages is calculated and recorded. The PTOF is used to calculate particle velocity and determine the particle dva with greater than 0.5% precision (Zelenyuk and Imre Citation2005; Zelenyuk et al. Citation2009a). The PTOF is also used to generate the trigger pulses for lasers (GAM Lasers, Model EX5), used to characterize the particle compositions. The trigger pulses are timed to fire both lasers precisely when the particle arrives in their focal spots situated at the ion source of the angular reflectron time-of-flight mass spectrometer (TOF-MS, R. M. Jordan, Inc., Model D-850) The TOF-MS is located 10.5 cm downstream from the 2nd optical detection stage (not shown). An infrared (CO2 laser) is used to evaporate the particle, and a time-delayed UV excimer laser pulse is used to ionize the evaporating plume and ablate the non-volatile aerosol fraction (Zelenyuk and Imre Citation2005; Zelenyuk et al. Citation2009a). Individual particle chemical composition is determined from the acquired single particle mass spectra (Zelenyuk et al. Citation2009a, Citation2009b).
FIG. 1 Schematic diagram of the inlet and particle detection region of SPLAT. The aerodynamic lens, on the left, forms a particle beam whose divergence depends on particle shape. Spherical particles form low-divergent beams (shown in blue), while aspherical particles form a slightly diverging beams (shown in red). The optical detection stages ODS1 and ODS2 are used to detect the particles and measure their time of flight. Note that the second laser beam interacts with the complete blue particle beam but misses a fraction of the red beam.
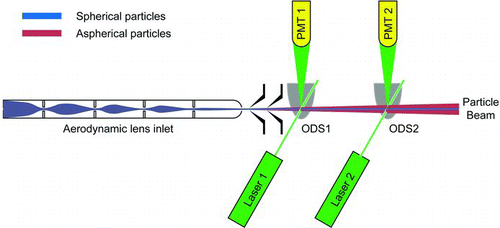
RESULTS AND DISCUSSION
Measuring Particle Number Concentration
The number concentration of aerosol particles is one of the most fundamental aerosol properties and is of prime importance in quantifying the effects that aerosols exert in different areas, especially aerosol effects on health and climate. The observed particle number concentration strongly depends on location and sources, and can significantly vary as function of time. This is particularly true for airborne measurements that require high temporal resolution.
The rate at which all SPMS can characterize both particle size and composition is limited by the rate at which desorption/ionization lasers can operate and the time it takes to acquire and record particle sizes and mass spectra (instrument busy time) (Allen et al. Citation2006; Pratt et al. Citation2009; Zelenyuk et al. Citation2009a). This effectively restricts most SPMS to characterizing less than 10 particles s–1. The sampling rate of SPLAT was recently significantly increased, making it possible to characterize the size and composition of up to 100 particles s–1 (Zelenyuk et al. Citation2009a). However, considering the typical ambient aerosol concentrations and SPLAT's high transmission and detection efficiencies, it is clear that the rate with which particles could be detected considerably exceeds this limit. In fact, during instrument alignment, using atomized sodium nitrate particles, particle detection rates in both ODS1 and ODS2 approach 100,000 particles s–1, which represent SPLAT's maximum detection rate.
To take advantage of SPLAT's high sensitivity and detection rate, the instrument control board (ICB), which is described in detail elsewhere (Zelenyuk et al. Citation2009a; 2009c), continuously records the particle time of flight between ODS1 and ODS2 and the detection rates in ODS1 and ODS2. As we show below, these recorded values can accurately be converted into particle number concentrations, size distributions, and can even be used to gain information about aerosol asphericity.
The instrument transmission and detection efficiencies of particles with diameters larger than 125 nm and smaller than 600 nm are 100%. Under most circumstances the number concentrations of atmospheric particles larger than 600 nm are very small, and thus their contribution to the overall particle number concentrations is negligible. Since SPLAT's transmission and detection efficiencies of small particles decrease extremely rapidly with particle size below ∼100 nm, the detection rate at ODS1 is directly proportional to the concentrations of particles larger than 100 nm and the instrument sampling flow rate, which is continuously measured and recorded. It is important to note that because particle beam divergence depends on particle shape (Huffman et al. Citation2005; Salcedo et al. Citation2007; Zelenyuk et al. Citation2009a) it might affect the ODS1 detection efficiency of aspherical particles. The fraction of particles missed by ODS1 depends on the instrument geometry, which includes particle beam diameter and divergence, and the laser beam diameter. To minimize the fraction of particles missed by ODS1 due to beam divergence we positioned ODS1 in SPLAT only 10 cm downstream from the nozzle of the aerodynamic lens and use detection lasers with relatively large focal spots (335 μm full width at half maximum (FWHM)). Indeed, measurements conducted during instrument characterization show that 100% of mildly aspherical particles are detected by ODS1. For example, using aspherical 200 nm NaCl particles with average dynamic shape factor of χ= 1.08 and number concentration, as determined by a condensation particle counter (CPC), of 12 particles cm–3 we measured a particle detection rate of 14.6 ± 4 particles s–1 at ODS1. Taking into account the measured instrument sampling flow rate of 1.3 cm3 s–1 and assuming 100% detection efficiency we calculate a particle concentration of 11.2 ± 3 particles cm–3, which is in good agreement with the CPC measured particle concentrations. In addition, we note that routine alignment and calibration procedures of SPLAT use spherical and aspherical particles with known sizes, shapes, and number concentrations.
We conclude that the rate of particle detection by PMT1 is directly proportional to the number concentration of particles larger than 100 nm and the proportionality constant is determined by the sampling flow of the instrument inlet. Under normal circumstances, the sampling flow rate is constant and determined by the critical orifice at the entrance into the aerodynamic lens inlet, but when the instrument is deployed on an aircraft, as was the case during ISDAC, the pressure change gives rise to changes in flow rate. This is handled by recording the ambient pressure as well as the pressure in the aerodynamic lens, both of which are used to calculate the exact inlet flow rate.
As we noted above, the use of measured detection rate and sampling flow rate to determine particle number concentrations is not limited to SPLAT. To adopt this approach and utilize it in any other instrument that uses particle optical detection requires characterization of the instrument's size-dependent detection efficiency, knowledge of the sampling flow rate, evaluation of a shape bias, and measurement of the particle detection rate.
shows as an example the measurements of the particle number concentrations observed during a ∼100 min long flight segment of the ISDAC field campaign. The figure displays two superimposed traces showing in black the number concentration of particles as measured by the passive cavity aerosol spectrometer probe (PCASP, PMS PCASP-100X) and in red the particle number concentrations as measured by SPLAT. Both measurements are displayed with 1 sec temporal resolution, and neither was adjusted or scaled. PCASP is an optical particle counter commonly used for measuring the concentration and size distribution of particles in the size range of 100 nm to 3 μm that are assumed to have a spherical shape, and an index of refraction between 1.52 and 1.59. A statistical comparison between the two traces yields an average difference between the two of 0.5%.
FIG. 2 (a) Particle number concentrations (particles cm–3) measured by SPLAT on a flight during the ISDAC campaign. The red trace shows concentrations derived from the particles detected by SPLAT at the first PMT while the black trace shows concentrations measured independently and simultaneously with a PCASP instrument. Both traces display data with 1 s resolution. (b) Expanded region of the traces in (a), exhibiting the excellent sensitivity and time-resolution of the measurement.
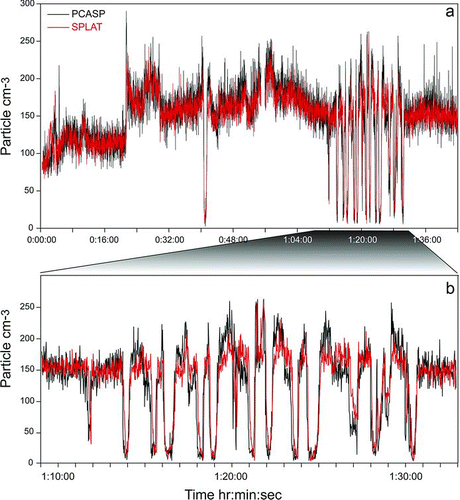
The quantitative agreement between SPLAT and PCASP provide conclusive evidence that the particle concentrations as determined by SPLAT with temporal resolution of 1 s are accurate. illustrates in an expanded scale a 24 min segment, during which the observed particle number concentrations change very rapidly, highlighting the requirement of fast data acquisition. This flight segment was designed to sample above, in, and below the cloud a number of times. When the aircraft is either above or below the cloud, the particle number concentration is ∼150 particles cm–3. The dips in the particle number concentrations correspond to in-cloud sampling and are due to the fact that the cloud droplets are not sampled and that in this cloud the number of particles that did not get activated as CCN is very small. Note that the transition from in-cloud to above or below cloud is extremely rapid and requires high temporal resolution to be properly quantified.
clearly demonstrates that in SPLAT the particle detection rate at ODS1 can be used to accurately quantify the ambient particle number concentrations with high temporal resolution. It offers an excellent method to measure ambient aerosol number concentrations without the need for external calibration or scaling factors, while single particle sizes and chemical compositions are simultaneously being collected.
Measuring Particle Size Distributions with High Temporal Resolution
The size distribution of atmospheric aerosols is an important attribute that determine aerosol behavior (Baron and Willeke 2001; McMurry Citation2000). Particle optical properties, CCN activity, lung deposition, and settling timescales in the atmosphere are all directly related to particle size. Moreover, as we show in the following paper (Vaden et al. Citation2011), particle size distributions measured in an instrument like SPLAT can even be used to estimate particle densities, which are useful for converting particle volumes into mass loads, identifying and quantifying particle internal compositions, and quantifying optical properties such as light absorption and scattering.
Allen et al. (Citation2006) developed a scheme that allows the operator to alternate the operation of their SPMS (ATOFMS) between two different data acquisition modes, normal and fast scatter. In both modes, individual particles were detected as light scattering events at two PMTs, and particle acquisition time and PTOF were recorded. In normal mode a timing circuit that monitors the PMT signals was used to trigger the ablation/ionization laser, and individual particle mass spectra were then processed and saved. In fast scatter mode, mass spectra were not generated and the acquisition rate was not limited to the maximum firing rate of the ablation/ionization laser of 10 Hz. The most recent version of this SPMS, in its airborne version (A-ATOFMS) can size at a rate of ∼35 particles s–1 and generate mass spectra at a rate of ∼4 particles s–1 (Pratt et al. Citation2009).
In SPLAT, particle size distributions are measured at a rate determined by the particle number concentrations and their detection probabilities, while in parallel the instrument records the size and composition of some of these particles at an operator set rate of up to 100 particles s–1. The temporal resolution with which particle dva distributions can be obtained by SPLAT clearly depends on the ambient particle number concentrations and size distributions. When aerosol number concentrations are low, obtaining statistically robust particle dva distributions with good temporal resolution requires high instrument sensitivity. Our experience shows that measuring size distributions of typical atmospheric polydisperse aerosol with reasonable signal to noise requires sizing ∼1000–2000 particles. If the particle size distribution is narrow, fewer particles are required.
In we provide a few examples that illustrate the use of SPLAT to measure dva distributions encountered during a few atmospheric events in ISDAC. The orange curve was measured during a high altitude flight segment, in which biomass-burning particles represented a significant fraction of the particles. These data were acquired in 60 s, the average particle number concentration was 150 particles cm–3 and the total number of particles sized was 9695. The blue curve represents the size distribution of 8079 particles that served as cloud condensation nuclei, whose average concentration was 63 particles cm–3, and were sampled for 60 s. The black curve shows the size distribution of 3130 interstitial particles whose concentration was 15 particles cm–3 and was obtained by sampling for 136 s. These three examples illustrate that it is possible to obtain high-quality particle size-distributions in ∼1 min even at relatively low particle number concentrations. The black size distribution of interstitial aerosols, obtained by sampling for 136 s, represents the entirety of data on interstitial particles during this flight. As mentioned above, the particle number concentrations are calculated based on the particle detection rate at ODS1 and the measured altitude-dependent sampling flow rates.
FIG. 3 dva distributions of particles that served as CCN, interstitial particles, and particles characterized at high altitude all measured during ISDAC campaign. The dva distribution of the CCN, interstitial, and high altitude particles were acquired in 60, 60, and 136 s, and contain 8,079, 9,695, and 3,130 sized particles, while the ambient particle concentrations were 63, 151, and 15 particles cm–3, respectively, illustrating the high temporal resolution of these measurements.
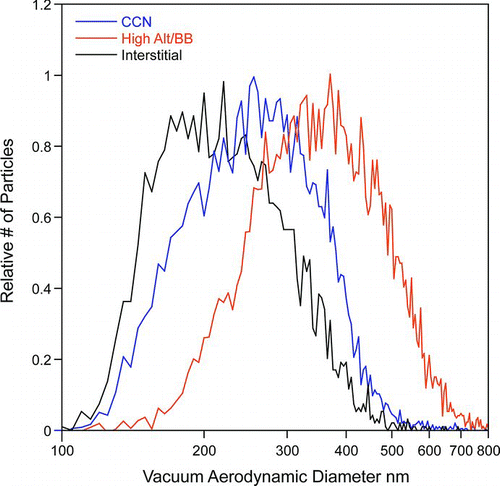
As discussed in the sections above, in SPLAT the vacuum aerodynamic diameter of individual particles (dva ) is calculated from the measured PTOF between the two optical detection stages, using an empirically derived PTOF-to-dva calibration that is obtained using either NIST-certified size standards or DMA-classified spherical particles of known density. In principle, dva distributions, such as those presented in , can be quickly measured for any ambient aerosol population, but a complication arises for aerosol samples with high particle number concentrations. At high concentrations, more than one particle may be found between the two optical detection stages at any time. In this case, a “false coincidence” between the two detectors will occur because PMT2 will detect the particle that was between the two PMTs, slightly after another particle was detected by PMT1. These false coincidences lead to erroneous PTOFs that are always shorter than the true PTOF, which translate into erroneously small dva values. Note that this does not affect the absolute particle number concentrations, which are determined exclusively by the rate of detection events at the first PMT. Furthermore, false coincidences do not negatively affect the sizes of particles whose mass spectra were recorded, because the laser timings that are based on false coincidences result in the lasers missing the particles and generating no mass spectra. For this reason, the size distributions of chemically characterized particles are free of false coincidences. However, since false coincidences reduce particle hit-rate, it is advantageous to keep the rate of particle detection below a few thousands per second.
shows three dva distributions measured by SPLAT during ISDAC, when the aircraft flew through an air parcel that contained high number concentration of biomass burning particles. The red size distribution was acquired in 60 s, during which 7,160 particles were sized and the ambient particle number concentrations were 250 particles cm–3. Unlike the size distributions in , this one shows an increasing number of particles smaller than 200 nm all the way down to 30 nm. This feature of the SPLAT measured size distribution is a clear indication that high number concentration of detectable particles produces false coincidences. Given that the SPLAT detection efficiency is known to drop-off very sharply as particle diameter decreases below 100 nm, this feature in is an unmistakable signature of false coincidences. It is therefore not surprising to find that when the ambient particle concentrations increase, as illustrated with the blue and green traces, the fraction of particles that belong to the false coincidences category increases. The blue trace was acquired in 60 s during which 15,134 particles were sized and the ambient particle number concentration was 500 particles cm–3. The green trace was acquired in 10 s sizing 5,365 particles with an ambient particle number concentration of 1,000 particles cm–3.
FIG. 4 (a) SPLAT-measured size distributions of ambient particles at relatively high concentrations during ISDAC, illustrating the effect of false coincidences. The three traces (red, blue, and green) were acquired in 60, 60, and 10 s, and contain 7,160, 15,134, and 5,365 sized particles while the ambient particle concentrations were 250, 500, and 1,000 particles cm–3, respectively. The dashed lines are exponential fits to the false coincidences (FC). (b) The corrected size distributions calculated by subtracting the false coincidences fraction.
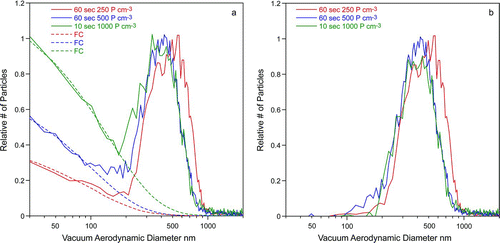
The three traces in clearly illustrate that it is possible to measure particles size distributions in a very short time and that, in addition to the properly sized particles, these dva distributions contain a fraction of data points with unrealistic values. The contributions of the false coincidences into dva distributions can be fit with simple empirically derived exponential functions, as shown by the dashed lines in . Once fit, the corrected size distributions are calculated simply by subtracting the false coincidence fractions to yield the dva distributions shown in .
Thus far, we presented examples of dva distributions measured by SPLAT under a range of conditions and acquisitions times. Because the quality of the measured size distributions depends on the particle size, the breadth of the distribution, and sampling time, it not easy to unequivocally state the maximum and minimum particle number concentrations that can be measured with SPLAT. presents, as an example, the SPLAT measured dva distributions for two cases, in which the encountered particle concentrations were approaching the high and low limits of the practical range of aerosol concentration.
FIG. 5 (a) Mobility size distribution of ambient particles measured during late afternoon on June 7 in Sacramento, CA. (b) Vacuum aerodynamic size distribution of 8,000 particles measured in 4 s. The figure shows the raw data (red), the exponential fit to the false coincidences (blue), and the corrected size distribution (green). (c) Vacuum aerodynamic size distribution of 110 DMA-classified SOA particles present at a number concentration of 0.6 particles cm–3, and sized in 2 min. Singly and doubly charged particles are observed.
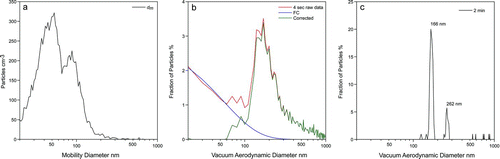
shows the mobility size distribution of ambient particles sampled during late afternoon on June 7 in Sacramento, CA. The particle size distribution shows two peaks at 53 and 88 nm, with a total number concentration of ∼10,000 particles cm–3, the diameter of 2,800 of which were larger than 82 nm and thus detectable with SPLAT equipped with a new aerodynamic lens inlet (Vaden et al. Citation2011). shows the observed dva distribution of 8,000 particles (red trace), which was obtained in just 4 s, during which time SPLAT detected 3,850 particles s–1 in ODS1, with a sampling flow rate of 1.3 cm3 s–1, yielding aerosol number concentration of 2,960 particles cm–3, in good agreement with the DMA measured number concentrations. The dva distribution in displays the clear indication of false coincidences, which was illustrated in . As before, the contribution of false coincidences was fit with an empirically derived exponential function (blue trace) and was then subtracted from the raw data to yield the corrected dva distribution (green trace). The corrected dva distribution in shows the main peak at 160 nm and an additional, smaller peak at 90 nm. Increasing the averaging time yields improved signal to noise, but does not change the overall shape of the dva distribution. Note that the fraction of data points classified as false coincidences in , with nearly 4,000 particles detected each second, happens to be smaller than that observed when measuring 1,000 particles cm–3 with detection rate of ∼500 particles s–1, shown in (green trace). The difference between these two cases relates to the number of particles detected in both ODSs. Nevertheless, the key here is that at high aerosol number concentrations false coincidences leave a clear signature that are easily removed to yield reliable size distributions.
To illustrate the low particle concentration limit we present an example, in which SPLAT was used to characterize DMA-classified secondary organic aerosol (SOA) particles at a concentration of 0.6 particles cm–3. The dva distribution of 110 SOA particles obtained in 2 minutes of sampling is shown in . It has 2 peaks at 166 nm and 261 nm that correspond to singly and doubly charged particles, respectively. While a few background counts, seen here above 500 nm, start to appear at these low concentrations, this example clearly shows that it is possible to acquired dva distributions even for aerosol concentrations below 0.6 particles cm–3. It is important to note, however, that in this case, as few as 110 DMA-classified particles were sufficient to yield dva distribution with good signal to noise ratio. To size ∼1,000 particles, as would be required for a polydisperse sample, at this concentration would take 20 min, which in many cases might still be practical.
demonstrates that SPLAT can be used to measure particle size distributions when the detectable particle concentrations are between 0.5 to ∼3,000 particles cm–3, with temporal resolution of a few seconds to a few minutes. The high temporal resolution is achieved by operating the instrument in the dual data acquisition mode that can be used by all single particle mass spectrometers.
Measuring Particle Beam Divergence to Infer Particle Asphericity
Particle shape is an important attribute that can play a central role in determining aerosol properties and behavior. Particles are routinely assumed to be spherical because of the difficulty of experimentally determining particle shape. However, it is well known that significant fraction of aerosol particles can be aspherical. Often, simply knowing the fraction of particles that are spherical/aspherical can greatly enhance our understanding of aerosol systems and aid in data interpretation. Particle asphericity has commonly been deduced using light scattering (Dick et al. Citation1998; Perry et al. Citation1978). In remote sensing, high laser depolarization ratios are used to indicate the presence of aspherical particles, and angle-resolved light scattering has been successfully used to determine particle sphericity during in-situ sampling. Recently we developed a new method for real-time identification of particle asphericity (Zelenyuk et al. Citation2008c), in which the combined SPLAT/DMA system is used to determine if particles are spherical or aspherical based on the line-shape of the vacuum aerodynamic diameter distribution of DMA-classified particles. In particular we demonstrated that asphericity causes broadening of the dva distributions for particles with a narrow distribution of mobility diameters (Zelenyuk et al. Citation2008c). We showed that increase in line-width is a reflection of the fact that the vacuum aerodynamic diameter of aspherical particles depends on the particle orientation.
The use of aerodynamic lenses as inlets by SPLAT and other aerosol mass spectrometers have opened up the opportunity to use particle beam divergence to characterize particle asphericity. A number of studies have shown that the particle beam divergence depends on particle shape, with spherical particles forming narrower and significantly lower divergence particle beams than those formed by aspherical particles (Katrib et al. Citation2005; Liu et al. 1995; Citation1995b; Murphy Citation2007; Schreiner et al. 1999; Zelenyuk and Imre Citation2005, Citation2007; Zelenyuk et al. Citation2009a). Hence, on a qualitative level particle beam divergence can be directly related to particle shape. The utility of this approach was first demonstrated with the aerosol mass spectrometer (AMS) (Huffman et al. Citation2005; Salcedo et al. Citation2007). The AMS was equipped with a thin translatable wire with which the particle beam diameter is measured by blocking a portion of the particle beam with the wire and translating it across the beam while quantifying the measured aerosol mass as a function of the wire position. The measured beam diameter was then used to deduce particle asphericity. This approach is somewhat limited by the fact that the instrument must be dedicated to making the beam profile measurements and cannot be simultaneously used to quantify other particle properties.
Here we demonstrate that in SPLAT the particle beam divergence can be quantified using the ratio of the number of particles detected by PMT1 to PMT2. The schematic, shown in , illustrates how particle beam divergence can be quantified in-real time by comparing the number of particles detected at each of the two ODSs. Since the diameters of the light-scattering laser beams (335 μm FWHM) are slightly larger than the aerosol beam for spherical particles, 100% of spherical particles are detected by both PMTs and the ratio PMT1/PMT2 is 1. When it comes to aspherical particles, the situation is different. Because the particle beams of aspherical particles have higher divergence, by the time aspherical particles reach ODS2, the particle beam diameter is larger than the 2nd light scattering-laser beam, fewer particles are detected by PMT2 than by PMT1, and the PMT1/PMT2 ratio is greater than 1, with more divergent beams having larger PMT1/PMT2 ratios. We term the PMT1/PMT2 ratio the particle beam divergence parameter (BDP), and note that particle asphericities can be monitored in real-time simply by measuring the ratio PMT1/PMT2.
In SPLAT the BDP is measured with 1 s resolution, while routine measurements of particles sizes and compositions are continuously carried out. Note, that when sampling ambient particles, each BDP data point is a measure of the average divergence of the particle beam, which most likely contains a mixture of particles of different sizes and compositions. This fact alone can make the interpretation of measured BDP of a complex aerosol mixture rather challenging. It is, therefore, useful to use the measured BDPs and their temporal variation in conjunction with other aerosol properties that are simultaneously measured by SPLAT, like particle compositions and size distributions. In most cases, it is possible to correlate the changes in BDP with observed temporal variations in particle compositions and/or size distributions.
Before we proceed to use measured BDP to characterize aspherical particles, we note that the particle beam divergence is not only a function of particle shape, but that it also depends on particle size (Liu et al. Citation1995a, Citation1995b; Wang and McMurry Citation2006). We therefore start by exploring the relationship between measured BDP and particle size for spherical particles, which is presented in in a plot of measured PMT1/PMT2 ratios versus particle size, for several types of laboratory-generated spherical particles. The figure shows that BDP is nearly 1.0 for all spherical particles larger than ∼150 nm, but increases to ∼1.5 as particle diameter decreases to 70 nm.
FIG. 6 Beam divergence parameters, or PMT (PMT1/PMT2) ratios, measured for several different sizes and types of laboratory-generated spherical particles. The 73 nm PSL data points (labeled 1 and 2) illustrate that for the very small particles the PMT ratios are sensitive to differences in the operator-set particle detection thresholds at the two ODSs (see text for more detail). Included also are the PMT ratios calculated based on an aerodynamic lens calculator and Equation (Equation1) (dashed line).
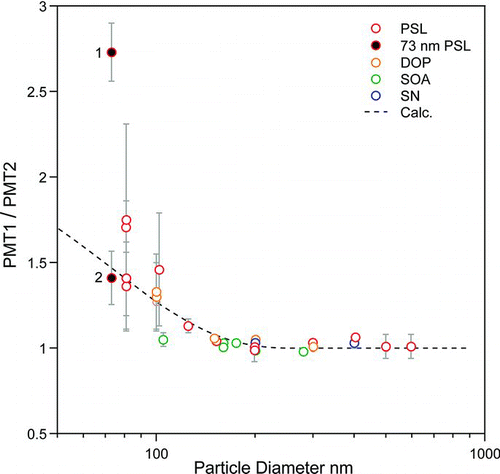
For spherical particles the BDP can be calculated by using the aerodynamic lens calculator (Wang and McMurry Citation2006). This program uses the aerodynamic lens configuration as input, and simulates, for spherical particles, the transmission through the individual lenses and the beam divergence for specified conditions like sizes, densities, flow conditions, etc. First, the aerosol beam diameters at ODS1 and ODS2 are calculated using the aerodynamic lens calculator, after which the BDP is calculated as the ratio of the overlaps between particle beam, assumed to be Gaussian, and the scattering laser beams at ODS1 and ODS2, according to Equation (Equation1):
The calculated BDP as a function of particle size for spherical particles is shown in (dashed line) together with the experimentally measured PMT1/PMT2 ratios. Particles larger than ∼150 nm form essentially non-divergent beams and are detected with 100% probability in both ODS1 and ODS2. Smaller particles form more divergent beams, and as a result have larger calculated and measured BDPs. Clearly, the model fits the observed value for all but the smallest particles.
Most notable is the scatter in measured BDPs for the 73 nm PSL particles, for which we show two data points, one with a BDP much larger than the calculated value and the other in good agreement with calculation. The larger discrepancy between measured and calculated BDP values for the 73 nm particles, labeled (Equation1) in , is a result of the peculiarity in the way particles are detected in SPLAT. Because of differences in light-scattering background, it is typical to set the threshold for the number photons that indicate particle detection at ODS2 at 1–2 photons higher than in ODS1. While, this does not affect the BDP of larger particles that produce signals much larger than threshold (Zelenyuk et al. Citation2009c), it does effect the detection probability of smaller particles. For 73 nm particles, 1 or 2 photons make a significant difference in detection probability, which result in fewer particles being detected at ODS2 and thus a larger observed BDP.
To test whether the discrepancy between calculated and observed BDP is indeed reflection of the difference between the operator set particle detection thresholds at ODS1 and ODS2, we increased the threshold at ODS1 to equalize the two and repeated the measurements. This measurement yielded the second BDP data point for 73 nm particles, labeled (2) in , which is in good agreement with calculated value. While intentional increase in particle detection threshold at ODS1 improves the BDP measurements of the very small particles, it also lowers the instrument's small particle detection efficiency, which is clearly undesirable. Instead, SPLAT is typically operated with two different thresholds set to the lowest values while assuring that with no particles present, the particle detection rates in both ODSs are zero.
Under most circumstances, given that the SPLAT detection efficiencies for particles smaller than 100 nm drop rather rapidly, we expect to find that for spherical ambient particles BDPs are between 1.0 and 1.1, and that BDP values above 1.1, indicate the presence of larger fraction of aspherical particles. However, when interpreting the observed BDPs it is important to use the measured particle size distributions to distinguish between the effect of size and shape, both of which influence particle beam divergence and thus the BDP.
We now proceed to test this approach by measuring the BDPs of laboratory-generated particles of known compositions, sizes, and shapes. shows the BDPs measured for 6 different DMA-classified particle types with temporal resolution of 1 s. NaNO3 (dp = 400 nm) and SOA (dp = 160 nm) particles are spherical (Zelenyuk et al. Citation2005, Citation2008c), and yield BDP of unity with very low scatter. The rest of the particles in are aspherical, (Zelenyuk et al. Citation2006; Zelenyuk and Imre Citation2009) and yield BDPs ranging from ∼1.5 to over 6.0. The spreads in the measured BDP as well as their values are useful for understanding particle shapes. For example, NaCl particles (dp = 300 nm) have BDP around 1.75, with a relatively narrow distribution of values. This is consistent with the fact that NaCl particles are cubic or nearly cubic with a relatively narrow distribution of shapes. The pyrene particles (dp = 200 nm) show larger BDP, but with a significantly narrower spread of values. This most likely indicates that the aspherical pyrene particles exist in very similar shapes. The Arizona test dust (dp = 200 nm) and fractal soot particles (dp = 250 nm) demonstrate another extreme, for which very broad distributions of BDPs are observed. These last two cases represent particles well known for having a wide range of shapes. The idea that there is a relationship between particle distributions of shapes and spread in BDPs is consistent with the line-shapes of the SPLAT measured dva distributions for these DMA-classified particles (Zelenyuk et al. Citation2008c).
FIG. 7 Beam divergence parameters, or PMT ratios, measured for different populations of aerosol particles with 1 s resolution. In each case, the particles were monitored during a specific time window, and thus, the data show the PMT ratios as a function of time. For each particle type, the average PMT ratio and the spread in the distribution of values are both useful for obtaining information on the particle asphericity. (Figure provided in color online.)
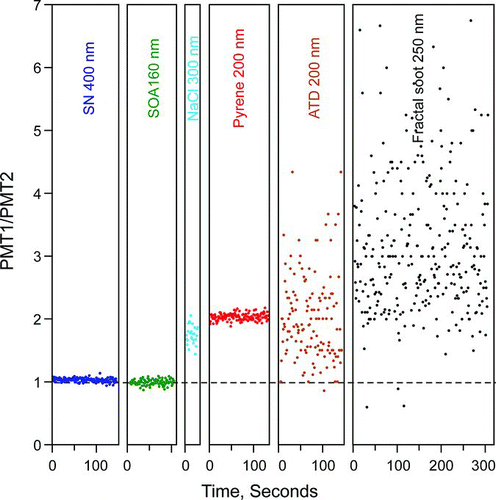
For example, the fractal soot data in spans a wide spectrum of BDPs. Since analysis of particle compositions, effective densities, and fractal dimension for these particles show that 100% of them are composed of fractal soot coated with thin layer of organics, the wide range of BDPs must reflect large variability in particle shapes. This conclusion is also consistent with our observation that the dva size distributions of these DMA-classified soot particles have a line-shape with 50% FWHM, which is ∼10 times larger than spherical particles and ∼5 times larger than widths of most other aspherical particle types that we have studied.
shows measured BDPs recorded for ambient aerosol particles characterized during two short flight segments in ISDAC field campaign. shows that from 2:20 to 2:30 (UTC) most of the particles were spherical. At 2:30 (UTC), a large spike in BDP is observed, which according to the particle mass spectra, is the result of the airplane flying through a narrow layer of soot particles. Following the encounter with the soot plume the BDP values vary between 1.1 and 1.5 and then, at 2:50 (UTC), the BDP values increase slowly to 2, and remain relatively elevated till 3:10 (UTC). The particle mass spectra show that from ∼2:50 (UTC) onwards, the airplane flew through a region, in which a majority of the characterized particles were composed of sulfates internally mixed with very small amount of organics. The higher BDP values combined with measured dva distributions indicate that these particles are aspherical.
FIG. 8 Examples of beam divergence parameters, or PMT ratios, continuously measured in ISDAC with 1 s resolution. (a) The large variations in BDPs observed at ∼2:30 UTC corresponds to the aircraft flying through a plume of soot. The latter third of this flight segment, which exhibits large BDPs corresponds to sampling of aspherical particles composed of sulfate and a very small amount of organics. (b) BDPs measured as the aircraft porpoised through a cloud sampling in the cloud, interstitial particles, and background particles above and below the cloud. The diagram above shows, on the same timescale, the aircraft altitude path, and its relation to the cloud. The BDP values indicate spherical particles throughout, except when interstitial particles were sampled. An examination of the mass spectra and particle size distributions shows that in this case high BDP values are due to the presence of a large fraction of small particles. (Figure provided in color online.)
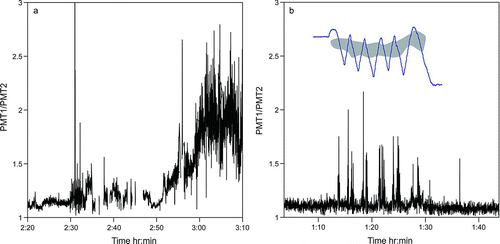
shows data acquired during the segment shown in . The inset at the top shows the flight altitude path through the cloud, drawn on the same timescale as the BDP data below. When the aircraft is either above or below the cloud, the data indicate that the vast majority of the detected particles are spherical and BDP is ∼1, but when the airplane flies in the cloud (where only interstitial particles are sampled) the average BDP value significantly increases. The measurements of particle compositions for this flight, which are presented in a separate publication (Zelenyuk et al. 2010), indicate that a larger fraction of interstitial particles are composed of dust as compared with background particles. However, dust particles represent much less than 1% of the particle population and do not explain the observed increase in BDP. The rest of the interstitial particles are composed of organics mixed with sulfate and have compositions nearly identical to those of particles above and below the cloud (Zelenyuk et al. 2010).
Since in this case the observed changes in BDP are not accompanied with changes in particle compositions, particle size is most likely the reason for the observed high BDP values. Earlier in the paper (), we presented a comparison between the size distribution of interstitial aerosols and that of CCN showing that interstitial particles are smaller. In addition, comparison between the SPLAT and CPC measured particle number concentrations shows that above and below the cloud the CPC measured number concentration is ∼15% higher than SPLAT. In other words, only 15% of background aerosol particles were smaller than 100 nm. In contrast, when measuring interstitial particles the CPC number concentration is 80% larger than SPLAT indicating that 80% of the interstitial particles are smaller than 100 nm. We conclude that the large BDPs in this case are mostly due to the presence of a large fraction of very small particles and not due to shape.
So far we emphasized how measured BDP can be used to obtain information that relates to particle shape. It is important to keep in mind that BDP is also a direct measure of the particle detection and characterization efficiency. Larger BDP indicate that a smaller fraction of particles that enter the instrument are sized and chemically characterized. Since, as we showed above, it is most often possible to correlate higher BDP values with specific particle compositions, this information can be used, in the analysis stage, to correct the contributions of different particles types to account for their losses due to particle beam divergence.
In addition, measured BDP values are routinely used in SPLAT during instrument alignment to assure that the numbers of spherical particles detected in the two ODSs are the same.
The approach described here to measure BDP in real-time can be adopted by all SPMS that use an aerodynamic lens inlet and two stages of particle optical detection. Many existing instruments already record data that can be used to calculate BDP, but did not take advantage of this information yet.
CONCLUSIONS
Single particle mass spectrometers have traditionally been deployed to measure the size and composition of individual particles at relatively slow sampling rates that are determined by the rate at which the ionization lasers can fire and/or mass spectra can be recorded. In this article, we showed that it is possible to extend the capabilities of SPMS by simultaneously operating them in two parallel modes, in which particle number concentrations, size distributions, and asphericities are measured with high temporal resolution and individual particle size and compositions are measured at a reduced rate. In this operational mode, particles are continuously detected and sized, but the evaporation/ionization lasers and mass-spectral acquisition are triggered at a rate that does not exceed that specified by the operator. It is important to understand that while the mass spectra and particle sizes represent individual particle characteristics, the additional information gathered at higher rate relates to the aerosol population ensemble.
We showed that in SPLAT the particle detection rate at ODS1 is directly related to the particle number concentration in the ambient atmosphere. Taking typical atmospheric particle densities, the SPLAT-derived particle concentration include particles larger than ∼100 nm and smaller than ∼600 nm. We demonstrated that it is possible to obtain information on particle dva distributions with temporal resolution of ∼1 min or less, depending on ambient aerosol number concentrations and size. We illustrated that for high particle number concentrations (300–3,000 particles cm–3) particle dva distributions contain some erroneously-sized particles, which we term false coincidences. We showed that false coincidences appear as particles with unreasonably small vacuum aerodynamic diameters and that they have a simple, exponential functional form whose influence on the measured dva distributions can easily be identified and corrected. As we will show in the second paper of this series, the measurements of dva distributions can be further used to determine average aerosol particle density and the densities of chemically resolved particle classes.
The particle beam divergence is dependent on particle size and shape. Since the particle beam divergence does not affect particle detection in ODS1, but decreases the number of particles detected at ODS2, the ratio of particle detection rates PMT1/PMT2 provides a direct measure of beam divergence, which needs to be analyzed together with the concurrently measured particle size distributions to identify particle asphericity.
The new methods described here were developed and demonstrated with SPLAT, but are easily adoptable to all SPMS that use an aerodynamic lens inlet and two stages of optical detection.
Acknowledgments
This work was supported by the U.S. Department of Energy (DOE) Office of Basic Energy Sciences, Division of Chemical Sciences, Geosciences, and Biosciences and Office of Biological and Environmental Research (OBER). Part of this research was performed in the Environmental Molecular Sciences Laboratory, a national scientific user facility sponsored by the DOE's OBER at Pacific Northwest National Laboratory (PNNL). PNNL is operated by the U.S. DOE by Battelle Memorial Institute under contract No. DE-AC06-76RL0 1830.
We thank the ISDAC and CARES teams for their incredible help during these field campaigns. ISDAC and CARES were supported by the U.S. DOE Atmospheric Radiation Measurement (ARM) Program Climate Research Facility, the DOE Atmospheric Sciences Program, the National Research Council of Canada and Environment Canada. Some of the data were obtained from the ARM program archive, sponsored by DOE OBER Environmental Science Division.
REFERENCES
- Allen , J. O. , Bhave , P. V. , Whiteaker , J. R. and Prather , K. A. 2006 . Instrument Busy Time and Mass Measurement Using Aerosol Time-of-Flight Mass Spectrometry . Aerosol Sci. Technol. , 40 : 615 – 626 .
- Baron , P. A. and Willeke , K. 2001 . Aerosol Measurement: Principles, Techniques, and Applications. , New York : John Wiley & Sons .
- Buzorius , G. , Zelenyuk , A. , Brechtel , F. and Imre , D. 2002 . Simultaneous Determination of Individual Ambient Particle Size, Hygroscopicity, and Composition . Geophys. Res. Lett. , 29 ( Artn ) : 1974
- Carson , P. G. , Johnston , M. V. and Wexler , A. S. 1997 . Real-Time Monitoring of the Surface and Total Composition of Aerosol Particles . Aerosol Sci. Technol. , 26 : 291 – 300 .
- Dick , W. D. , Ziemann , P. J. , Huang , P. F. and McMurry , P. H. 1998 . Optical Shape Fraction Measurements of Submicrometre Laboratory and Atmospheric Aerosols . Measurement Sci. & Technol. , 9 : 183 – 196 .
- Herich , H. , Kammermann , L. , Friedman , B. , Gross , D. S. , Weingartner , E. , Lohmann , U. , Spichtinger , P. , Gysel , M. , Baltensperger , U. and Cziczo , D. J. 2009 . Subarctic Atmospheric Aerosol Composition: Hygroscopic Growth Properties . J. Geophys. Res.—Atmos. , 114 ( Artn ) : D13204
- Huffman , J. A. , Jayne , J. T. , Drewnick , F. , Aiken , A. C. , Onasch , T. , Worsnop , D. R. and Jimenez , J. L. 2005 . Design, Modeling, Optimization, and Experimental Tests of a Particle Beam Width Probe for the Aerodyne Aerosol Mass Spectrometer . Aerosol Sci. Technol. , 39 : 1143 – 1163 .
- Katrib , Y. , Martin , S. T. , Rudich , Y. , Davidovits , P. , Jayne , J. T. and Worsnop , D. R. 2005 . Density Changes of Aerosol Particles as a Result of Chemical Reaction . Atmos. Chem. Phys. , 5 : 275 – 291 .
- Liu , P. , Ziemann , P. J. , Kittelson , D. B. and Mcmurry , P. H. 1995a . Generating Particle Beams of Controlled Dimensions and Divergence: Theory of Particle Motion in Aerodynamic Lenses and Nozzle Expansions . Aerosol Sci. Technol. , 22 : 293 – 313 .
- Liu , P. , Ziemann , P. J. , Kittelson , D. B. and Mcmurry , P. H. 1995b . Generating Particle Beams of Controlled Dimensions and Divergence: Experimental Evaluation of Particle Motion in Aerodynamic Lenses and Nozzle Expansions . Aerosol Sci. Technol. , 22 : 314 – 324 .
- McFarquhar , G. M. , Ghan , S. , Verlinde , J. , Alexei Korolev , J. , Strapp , W. , Schmid , B. , Tomlinson , J. M. , Wolde , M. , Brooks , S. D. , Cziczo , D. , Dubey , M. K. , Fan , J. , Flynn , C. , Gultepe , I. , Hubbe , J. , Gilles , M. K. , Laskin , A. , Lawson , P. , Leaitch , R. , Liu , P. , Liu , X. , Lubin , D. , Mazzoleni , C. , Macdonald , A.-M. , Moffet , R. C. , Morrison , H. , Ovchinnikov , M. , Shupe , M. D. , Turner , D. D. , Xie , S. , Zelenyuk , A. , Bae , K. , Freer , M. and Glen , A. 2010 . Indirect and Semi-Direct Aerosol Campaign (ISDAC): The Impact of Arctic Aerosols on Clouds . Bulletin of the American Meteorological Society. , doi: 10.1175/2010BAMS2935.1
- McMurry , P. H. 2000 . A Review of Atmospheric Aerosol Mmeasurements . Atmos. Environ. , 34 : 1959 – 1999 .
- Moffet , R. C. and Prather , K. A. 2005 . Extending ATOFMS Measurements to Include Refractive Index and Density . Anal. Chem. , 77 : 6535 – 6541 .
- Murphy , D. M. 2007 . The Design of Single Particle Laser Mass Spectrometers . Mass Spectrom. Rev. , 26 : 150 – 165 .
- Murphy , D. M. , Cziczo , D. J. , Hudson , P. K. , Schein , M. E. and Thomson , D. S. 2004 . Particle Density Inferred from Simultaneous Optical and Aerodynamic Diameters Sorted by Composition . J. Aerosol Sci. , 35 : 135 – 139 .
- Perry , R. J. , Hunt , A. J. and Huffman , D. R. 1978 . Experimental Determinations of Mueller Scattering Matrices for Nonspherical Particles . Appl. Opt. , 17 : 2700 – 2710 .
- Pratt , K. A. , Mayer , J. E. , Holecek , J. C. , Moffet , R. C. , Sanchez , R. O. , Rebotier , T. P. , Furutani , H. , Gonin , M. , Fuhrer , K. , Su , Y. X. , Guazzotti , S. and Prather , K. A. 2009 . Development and Characterization of an Aircraft Aerosol Time-of-Flight Mass Spectrometer . Anal. Chem. , 81 : 1792 – 1800 .
- Salcedo , D. , Onasch , T. B. , Canagaratna , M. R. , Dzepina , K. , Huffman , J. A. , Jayne , J. T. , Worsnop , D. R. , Kolb , C. E. , Weimer , S. , Drewnick , F. , Allan , J. D. , Delia , A. E. and Jimenez , J. L. 2007 . Technical Note: Use of a Beam Width Probe in an Aerosol Mass Spectrometer to Monitor Particle Collection Efficiency in the Field . Atmos. Chem. Phys. , 7 : 549 – 556 .
- Schreiner , J. , Schild , U. , Voigt , C. and Mauersberger , K. 1999 . Focusing of Aerosols into a Particle Beam at Pressures from 10 to 150 Torr . Aerosol Sci. Technol. , 31 : 373 – 382 .
- Spencer , M. T. , Shields , L. G. and Prather , K. A. 2007 . Simultaneous Measurement of the Effective Density and Chemical Composition of Ambient Aerosol Particles . Environ. Sci. Technol. , 41 : 1303 – 1309 .
- Thomson , D. S. , Schein , M. E. and Murphy , D. M. 2000 . Particle Analysis by Laser Mass Spectrometry WB-57F Instrument Overview . Aerosol Sci. Technol. , 33 : 153 – 169 .
- Vaden , T. D. , Imre , D. , Beranek , J. and Zelenyuk , A. 2011 . Extending the Capabilities of Single Particle Mass Spectrometry: Measurements of Aerosol Particle Density without DMA . Aerosol Sci. Technol. , 45 : 125 – 135 .
- Vaden , T. D. , Song , C. , Zaveri , R. A. , Imre , D. and Zelenyuk , A. 2010 . Morphology of Mixed Primary and Secondary Organic Particles and the Adsorption of Spectator Organic Gases During Aerosol Formation . Proceedings of the National Academy of Sciences. , 107 : 6658 – 6663 .
- Wang , X. L. and McMurry , P. H. 2006 . A Design Tool for Aerodynamic Lens Systems . Aerosol Sci. Technol. , 40 : 320 – 334 .
- Woods , E. , Smith , G. D. , Miller , R. E. and Baer , T. 2002 . Depth Profiling of Heterogeneously Mixed Aerosol Particles Using Single-Particle Mass Spectrometry . Anal. Chem. , 74 : 1642 – 1649 .
- Zelenyuk , A. , Cai , Y. , Chieffo , L. and Imre , D. 2005 . High Precision Density Measurements of Single Particles: The Density of Metastable Phases . Aerosol Sci. Technol. , 39 : 972 – 986 .
- Zelenyuk , A. , Cai , Y. and Imre , D. 2006 . From Agglomerates of Spheres to Irregularly Shaped Particles: Determination of Dynamic Shape Factors from Measurements of Mobility and Vacuum Aerodynamic Diameters . Aerosol Sci. Technol. , 40 : 197 – 217 .
- Zelenyuk , A. and Imre , D. 2005 . Single Particle Laser Ablation Time-of-Flight Mass Spectrometer: An Introduction to SPLAT . Aerosol Sci. Technol. , 39 : 554 – 568 .
- Zelenyuk , A. and Imre , D. 2007 . On the Effect of Particle Alignment in the DMA . Aerosol Sci. Technol. , 41 : 112 – 124 .
- Zelenyuk , A. and Imre , D. 2009 . Beyond Single Particle Mass Spectrometry: Multidimensional Characterisation of Individual Aerosol Particles . Intl Rev. in Phys. Chem. , 28 : 309 – 358 .
- Zelenyuk , A. , Imre , D. , Cuadra-Rodriguez , L. A. and Ellison , B. 2007 . Measurements and Interpretation of the Effect of a Soluble Organic Surfactant on the Density, Shape, and Water Uptake of Hygroscopic Particles . J. Aerosol Sci. , 38 : 903 – 923 .
- Zelenyuk , A. , Imre , D. , Earle , M. , Easter , R. , Korolev , A. , Leaitch , R. , Liu , P. , Macdonald , A. M. , Ovchinnikov , M. and Strapp , W. 2010 . In-Situ Characterization of Cloud Condensation Nuclei, Interstitial, and Background Particles using Single Particle Mass Spectrometer, SPLAT II . Anal. Chem. , 82 ( 19 ) : 7943 – 7981 .
- Zelenyuk , A. , Imre , D. , Han , J. H. and Oatis , S. 2008a . Simultaneous Measurements of Individual Ambient Particle Size, Composition, Effective density, and Hygroscopicity . Anal. Chem. , 80 : 1401 – 1407 .
- Zelenyuk , A. , Yang , J. , Choi , E. and Imre , D. 2009a . SPLAT II: An Aircraft Compatible, Ultra-Sensitive, High Precision Instrument for In-Situ Characterization of the Size and Composition of Fine and Ultrafine Particles . Aerosol Sci. Technol. , 43 : 411 – 424 .
- Zelenyuk , A. , Yang , J. and Imre , D. 2009b . Comparison between Mass Spectra of Individual Organic Particles Generated by UV Laser Ablation and in the IR/UV Two-Step Mode . Intl J. Mass Spectrom. , 282 : 6 – 12 .
- Zelenyuk , A. , Yang , J. , Imre , D. and Choi , E. 2009c . Achieving Size Independent Hit-Rate in Single Particle Mass Spectrometry . Aerosol Sci. Technol. , 43 : 305 – 310 .
- Zelenyuk , A. , Yang , J. , Song , C. , Zaveri , R. A. and Imre , D. 2008b . “Depth-Profiling” and Quantitative Characterization of the Size, Composition, Shape, Density, and Morphology of Fine Particles with SPLAT, a Single-Particle Mass Spectrometer . J. Phys. Chem. A. , 112 : 669 – 677 .
- Zelenyuk , A. , Yang , J. , Song , C. , Zaveri , R. A. and Imre , D. 2008c . A New Real-Time Method for Determining Particles' Sphericity and Density: Application to Secondary Organic Aerosol Formed by Ozonolysis of Alpha-Pinene . Environ. Sci. Technol. , 42 : 8033 – 8038 .