Abstract
Particle density is an important and useful property that is difficult to measure because it usually requires two separate instruments to measure two particle attributes. As density measurements are often performed on size-classified particles, they are hampered by low particle numbers, and hence poor temporal resolution. We present here a new method for measuring particle densities using our single particle mass spectrometer, SPLAT. This method takes advantage of the fact that the detection efficiency in our single particle mass spectrometer drops off very rapidly as the particle size decreases below 100 nm creating a distinct sharp feature on the small particle side of the vacuum aerodynamic size distribution. Thus, the two quantities needed to determine particle density, the particle diameter and vacuum aerodynamic diameter, are known. We first test this method on particles of known compositions and densities to find that the densities it yields are accurate. We then apply the method to obtain the densities of particles that were characterized during instrument field deployments. We illustrate how the method can also be used to measure the density of chemically resolved particles. In addition, we present a new method to characterize the instrument detection efficiency as a function of particle size that relies on measuring the mobility and vacuum aerodynamic size distributions of polydisperse spherical particles of known density. We show that a new aerodynamic lens used in SPLAT II improves instrument performance, making it possible to detect 83 nm particles with 50% efficiency.
INTRODUCTION
One of the most informative physical properties of atmospheric particles is the particle density. Knowledge of particle densities is needed to calculate aerosol mass loading and yields of secondary organic aerosol (SOA) formation from measured particle volumes. The density is related to the particle composition and in the absence of composition measurements can even be used, in field measurements, to estimate an average aerosol composition (CitationMcMurry et al. 2002). Monitoring changes in aerosol densities due to changes in their composition can be used to follow heterogeneous chemical reactions (CitationKatrib et al. 2005; CitationZelenyuk et al. 2010). Particle densities are also correlated with their optical properties (CitationMoffet and Prather 2005; CitationTang and Munkelwitz 1994) and hygroscopic growth factors (CitationZelenyuk et al. 2008a).
With the advent of single particle mass spectrometry simultaneous measurements of particle compositions and densities have become possible (CitationMurphy et al. 2004; CitationSpencer et al. 2007; CitationZelenyuk and Imre 2009; CitationZelenyuk et al. 2008a, Citation2008c, Citation2008d). The simultaneous measurements of densities and internal composition for the same individual particles make it possible to obtain quantitative information on particle composition (CitationZelenyuk et al. 2008a; CitationZelenyuk et al. 2008c) and aid in particle identification (CitationHering and Stolzenburg 1995; CitationMcMurry et al. 2002; CitationZelenyuk et al. 2008a). Furthermore, particle densities were shown to be particularly useful for quantifying water retention by particles composed of inorganic salts coated with organic surfactants (CitationZelenyuk et al. 2007) and SOA (CitationZelenyuk et al. 2010). Monitoring density changes of coated particles makes it possible to detect organic coatings less than a monolayer (CitationZelenyuk et al. 2010, Citation2007).
Particle densities, or effective densities for aspherical particles (CitationDeCarlo et al. 2004), are typically determined from measurements of two particle attributes using two different instruments (CitationAlfarra et al. 2006; CitationBahreini et al. 2005; CitationDeCarlo et al. 2004; CitationHand and Kreidenweis 2002; CitationHering and Stolzenburg 1995; CitationKelly and McMurry 1992; CitationMcMurry et al. 2002; CitationMorawska et al. 1999; CitationMurphy et al. 2004; CitationPitz et al. 2003; CitationSchleicher et al. 1995; CitationSpencer et al. 2007; CitationStein et al. 1994; CitationVirtanen et al. 2004; CitationZelenyuk et al. 2005, Citation2008a, Citation2008d). These measurements are carried out either in series on the same particle, or in parallel on the same aerosol population. McMurry et al. (2002) have used a differential mobility analyzer (DMA) to classify particles of a single mobility diameter, dm , and then measured their mass with an aerosol particle mass analyzer (APM). To reduce sampling time and achieve higher transmission CitationMalloy et al. (2009) have reversed the order of the traditional DMA-APM system, by selecting particles of a given mass with the APM and analyzing them with a DMA. CitationMurphy et al. (2004) have used their single particle mass spectrometer to measure density of individual particle on the basis of the ratio of particle vacuum aerodynamic diameter and particle diameter obtained using light scattering intensity.
The measurements of particle vacuum aerodynamic diameter, , and mobility diameter, dm
, are also commonly combined to obtain particle density (CitationAlfarra et al. 2006; CitationBahreini et al. 2005; CitationDeCarlo et al. 2004; CitationKatrib et al. 2005; CitationSpencer et al. 2007; CitationZelenyuk et al. 2005, Citation2008d). When dm
and
distributions are measured separately, the transmission functions of both instruments must be considered. Moreover, the average density is calculated under the assumption that particle density is size-independent. Size-resolved density can only be obtained by measuring vacuum aerodynamic diameters of DMA-classified particles (CitationSpencer et al. 2007; CitationZelenyuk et al. 2005, Citation2006a, Citation2008a, Citation2008d). This approach reveals that ambient particles are often composed of rich and complex mixtures, with size-dependent compositions and, hence, densities (CitationSpencer et al. 2007; CitationZelenyuk et al. 2008a). This method also yields more precise particle densities (CitationZelenyuk et al. 2005, Citation2008d) and in some cases the measurements that yield density can be used to obtain particle shape, phase, and dynamic shape factors (CitationVaden et al. 2010; CitationZelenyuk and Imre 2007; CitationZelenyuk et al. 2008c).
The particle shape itself may play a role in measurements of densities. It is common to treat asphericity by defining a quantity called effective density, , identical to density in the way it is calculated, except that it denotes that particles could be aspherical (CitationDeCarlo et al. 2004; CitationZelenyuk et al. 2006a). Since the majority of atmospheric particles are either spherical or only slightly aspherical (CitationSalcedo et al. 2006) the differences between density and effective density are relatively small making the assumption of particle sphericity reasonable. Cases of dust and soot particles, in which shape plays an important role, can easily be identified based on the particle mass spectra and need to be treated separately. When the method presented here is used to determine the average density of an ensemble of internally and externally mixed particles, we rely on a newly developed approach to characterize the asphericity of ensembles of particles in real-time with high temporal resolution (CitationVaden et al. 2011) to assess whether shape plays an important role in determining the calculated density.
We begin by introducing a new method to obtain particle density directly from the vacuum aerodynamic size distributions measured by our single particle mass spectrometer, SPLAT II (for simplicity, just SPLAT (CitationZelenyuk et al. 2009a)). This method requires no DMA and yields particle density as a byproduct of routine measurements of polydisperse particle size and composition, enabling facile real-time monitoring of particle densities during field measurements with minimal assumptions or approximations about the ambient particle population. We test the approach on laboratory-generated particles and then apply it to ambient aerosol population composed of a mixture of internally and externally mixed particles and to particles of specific compositions.
We present data obtained with a new aerodynamic lens, whose improved performance significantly increases detection efficiency of small particles. In addition, we illustrate a new, fast, and simple method to measure the instrument size-dependent detection efficiency. We show how a detailed analysis of measured vacuum aerodynamic size distributions for polydisperse spherical particles of known size distributions and densities can be used to characterize the instrument detection efficiency as a function of particle size. We use this approach to obtain the detection efficiency curve of SPLAT equipped with the new aerodynamic lens inlet and show that with this lens SPLAT's 50% detection point was improved from 109 nm to 83 nm.
Experimental
Sodium nitrate particles were generated from a 0.1 M aqueous solution of NaNO3 (Fisher Scientific, >99.5% purity) with an atomizer (TSI Inc., Model 3076), and dried using two diffusion driers (TSI Inc., Model 3062) in series before being loaded into a 100 L Teflon bag. These particles were further dried by mixing/diluting with dry (Relative Humidity (RH) less than 0.5%) particle-free air using dilution ratio of ∼100, which resulted in particle number concentration of particle/cm3. Number concentrations and size distributions of NaNO3 particles as well as particles sampled directly from the ambient air were measured using a condensational particle counter (CPC, TSI Inc., Model 3786) and a scanning mobility particle sizer (SMPS, TSI Inc., Model 3936), respectively. Particle
distributions for the NaNO3 particles, as well as for ambient laboratory particles, were measured with SPLAT, as has been described in detail elsewhere (CitationZelenyuk and Imre 2009; CitationZelenyuk et al. 2009a). Briefly, particles enter into the instrument through an aerodynamic lens inlet used to transport the particles from the ambient air into the vacuum system with extremely high efficiencies (CitationZelenyuk et al. 2009a). The aerodynamic lens forms a low divergence particle beam and imparts on each particle a velocity that is a narrow function of the
(CitationZelenyuk et al. 2009a). Individual particles are detected by light scattering at two optical detection stages located 10.5 cm apart. The particle time of flight (PTOF) between the two stages is used to calculate particle velocity and determine the
with 0.5% precision (CitationZelenyuk and Imre 2005; CitationZelenyuk et al. 2009a). The particle detection event and the PTOF are then used to generate triggers to fire the IR and UV lasers for particle evaporation and ionization, respectively (CitationZelenyuk et al. 2009c). Individual particle chemical compositions are determined from the acquired mass spectra using an angular reflectron time-of-flight mass spectrometer (CitationZelenyuk et al. 2009a, Citation2009b). High-precision density measurements of NaNO3 and ambient laboratory particles, by size-selecting particles with the DMA and measuring their
with SPLAT as described in detail elsewhere (CitationZelenyuk et al. 2005; CitationZelenyuk and Imre 2009; CitationZelenyuk et al. 2008d).
Here, we present an approach that takes advantage of the distinctive feature of the SPLAT measured particle vacuum aerodynamic distributions that is a reflection of the rapid drop-off in instrument detection efficiency on the small-particle side. To avoid confusion we use to denote the true particle vacuum aerodynamic size distribution and define
, to denote the SPLAT measured vacuum aerodynamic size distribution that depends on the
distribution and the SPLAT detection efficiency curve.
The ability to use the left-edge of the distribution to estimate particle densities necessitates knowledge of the detection efficiency curve for particles with diameters between ∼50 and ∼150 nm. The data presented in this article were obtained with two different aerodynamic lens inlets. SPLAT equipped with the older lens inlet configuration (CitationZelenyuk and Imre 2005) was carefully characterized by measuring the SPLAT detection efficiency of NIST-certified size standards (spherical polystyrene latex spheres) of known diameters, whose concentrations were determined with a condensation particle counter (CPC) (CitationZelenyuk et al. 2009a); this measurement yielded a 50% detection efficiency at dp
=109 nm.
A new aerodynamic lens system was used for some of the experiments presented here. Like the old lens, it has outer diameter of 0.500 inches, and contains six apertures with the same diameters ranging from 3 mm to 5 mm that are placed at the same positions as before. What is different is that the inner diameter (ID) of the new lens is 0.3735 inches as compared with the old lens whose ID was 0.402 inches, and the apertures in the new lens are designed to assure their precise, reproducible positioning during lens assembly. This is accomplished by machining each aperture to be an integral part of a sleeve that is only 0.0015 inches smaller than the lens ID, as compared with the old design, in which the apertures were 0.01 inches thick flat disks and 0.007 inches smaller in diameter than the tube ID, leaving some room for misalignment. The combined effect of tight tolerances and precise machining assures exact, reproducible alignment of the aerodynamic beam.
Below we demonstrate the application of a new, much simpler approach to obtain the instrument's detection efficiency vs. d
p
curve by measuring the distribution of polydisperse aerosol population composed of spherical particles of known density, for which the particle size distribution is measured with a DMA. We apply this procedure to the new lens using dry NaNO3 particles and find that the instrument's new detection probability drops to 50% at dp
= 83 nm.
Field measurements of aerosol particles presented here were conducted during the Indirect and Semi-Direct Aerosol Campaign (ISDAC), in which SPLAT was deployed onboard the Canadian National Research Council Convair 580 aircraft (CitationMcFarquhar et al. 2010; CitationZelenyuk et al. 2009a) and during the recent Carbonaceous Aerosols and Radiative Effects Study (CARES) field campaign that took place in Sacramento, CA in June 2010.
Simulations of the
Distribution and Its Use in Determining Particle Density
We begin by pointing out that the vacuum aerodynamic size distributions of polydisperse aerosol, as measured by SPLAT, , have a distinctive line-shape (CitationMcFarquhar et al. 2010; CitationZelenyuk and Imre 2005). For the vast majority of atmospheric particles the large-particle side of
is determined by the particle vacuum aerodynamic size distribution while in contrast, the sharp drop-off on the small-particle side reflects the fact that the instrument detection efficiency decreases rapidly as particle true size, dp
, decreases below ∼100 nm (CitationZelenyuk et al. 2009a). The instrument's detection sensitivity is a function of the particle true diameter and SPLAT measures vacuum aerodynamic diameter,
. Therefore, the sharp drop-off on the left edge of the
curve is almost entirely determined by the instrument detection efficiency, which is a known function of dp
. The combined knowledge of dp
and
can be used to calculate particle density, ρ
p
, or for aspherical particles effective density, ρ
(CitationDeCarlo et al. 2004; CitationZelenyuk et al. 2005; Citation2006a; CitationZelenyuk and Imre 2009) using Equation (Equation1):

The instrument (SPLAT) detection efficiency as a function of particle diameter can be fit using an approximate form given in Equation (Equation2):
FIG. 1 (a) A fit of the experimental small-particle detection efficiency curve (CitationZelenyuk et al. 2009b) using Equation (Equation1); (b) A typical log-normal size distribution (red) and the corresponding SPLAT detected vacuum aerodynamic size distribution (


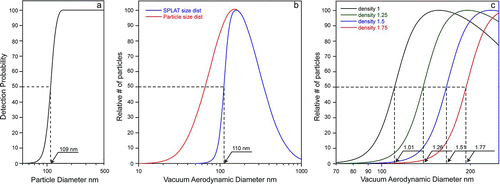
The effect of this sharp decrease in detection efficiency for small particles on a typical log-normal particle size distribution is shown in . The curve is a product of the particle sizedistribution and the particle detection efficiency curve, i.e.,


It is possible to obtain a relatively accurate particle density from by noting that the at the 50% point (on the left-edge) is 110 nm and knowing that the instrument detection efficiency drops to 50% at 109 nm to calculate a particle density of 1.01 g cm−3, which is only 1% different from the true value, ρ
p
.
Simulated distributions for particles with different densities are shown in . These curves are generated using Equation (Equation3) with the same parameters as in , but with increasing values of ρ
p
as indicated in the legend of . The shape of the left-edge does not change with density, because only the position of the
distribution is affected by density under constant davg
p
and ln σ. The density values estimated from the 50% point on the left-edges are shown at the bottom of the figure, and are all within 1% of the exact particle density.
demonstrates that once the halfway point of the detection efficiency curve is determined (for a given instrument configuration), accurate estimates of particle densities can be obtained from the halfway point of the left-edge of the distribution. One reason for the high accuracy of the estimated densities shown in is the fact that in this case davg
p
= 100 nm, which is very close to the halfway point of the detection efficiency curve (109 nm). For particles with davg
p
much larger or much smaller than 109 nm, the shape of the left-edge of
will be slightly impacted by davg
p
and the density estimate would be expected to be less accurate. This is illustrated in , in which simulated
curves with ρ
p
= 1.0 g cm−3 and ln σ=0.7, and increasing davg
p
values as indicated in the legend are shown. Note that while davg
p
changes by a factor of 5, from 50 nm to 250 nm, the densities estimated from the left-edge of all curves changes only from 0.93 g cm3 to 1.13 g cm−3. These densities, shown at the bottom of , are all reasonably close to the exact density (1.0 g cm−3).

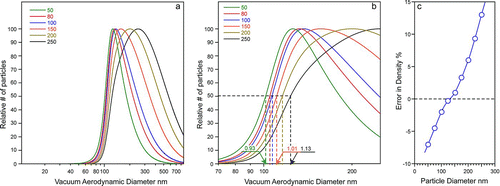
Nevertheless, a number of ways exist by which the accuracy of the estimated density can be improved. The most obvious is to take the effect of davg
p
and σ on the shape of the observed into account by fitting the observed
distribution with Equation (Equation3). This approach will be illustrated in the next section below. An alternative approach is to generate a correction curve in which the errors in estimated densities as a function of davg
p
and σ are quantified. The error curve generated for the estimated densities in is shown in . For davg
p
below 109 nm, the errors are negative, i.e., the estimated densities based on the 50% point are underestimated, and for davg
p
above 109 nm, the errors are positive, i.e., the densities based on the 50% point are overestimated, and the further davg
p
is from 109 nm, the larger the required corrections. Note, however, that even for particles with davg
p
as large as 250 nm, when nearly the entire aerosol population (98% and 96.5% for ln σ=0.7 and 0.5, respectively) are detected by SPLAT, the uncorrected density estimates are still less than 15% too high. Most importantly, the density obtained based on the 50% point can be used to calculate a fairly precise value for davg
p
using the observed
and the 50% point estimated density. For example, consider a
curve with a maximum at
nm and a 50% point at 131 nm, which yields an estimated density of 131/109 = 1.2 g cm−3. This approximate density is used to calculatedavg
p
∼200/1.2=167 nm. davg
p
= 167 is then used to obtain, from a 2.5% correction factor that needs to be applied to the density estimate of 1.2 g cm−3, yielding an improved density estimate of 1.17 g cm−3.
Improved Detection Probability of Small Particles and a New Method for Establishing the Instrument Detection Efficiency Curve
We now proceed to apply the above methodology to estimate particle densities from laboratory aerosol measurements. However, as we have mentioned above the instrument in its new configuration has a new aerodynamic lens inlet, for which a detection efficiency curve has not been established yet. To apply the method described above we must first establish the new SPLAT detection efficiency vs. d p curve. We start with dry, spherical NaNO3 particles, but because the exact density of these particles depends on the drying process (CitationZelenyuk et al. 2005, Citation2010) we first establish their density under present drying conditions with a high-precision method using a DMA and SPLAT connected in series. The measurements performed for DMA-classified particles with diameters 100 and 200 nm (not shown) yield a density of 2.07 ± 0.02 g cm−3, which is consistent with our previous measurements (CitationCai et al. 2006; CitationZelenyuk et al. 2005, Citation2010, Citation2007).
The mobility size distribution of the polydisperse NaNO3 aerosol is shown in (blue curve). The curve of the NaNO3 aerosol shown in (blue curve) clearly has a similar shape to that simulated in . In principle, the high-precision density and the log-normal distribution of the aerosol mobility size distribution could be used to fit the data in with Equation (Equation3) to obtain parameters a and b. We choose instead to test whether it is possible to use
alone to obtain the detection efficiency curve, particle density, and even the mobility size distribution. The results are shown as the red curves in Figures and and the derived parameters are listed in .
FIG. 3 (a) Mobility size distribution of polydisperse NaNO3 particles obtained with a SMPS (blue) and a fit using parameters listed in (red). See text for details; (b) Experimentally observed
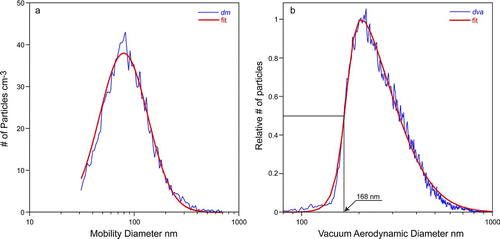
TABLE 1 Parameters obtained by fitting the NaNO
distribution with Equation (Equation3)
The excellent fits shown in indicate that the line-shapes determined by Equation (Equation3) are good representations of which include the new SPLAT detection efficiency curve and the particle size distributions. The davg
p
of 79 nm obtained from the SPLAT data alone compares very well with the 80 nm value from the SMPS measurement. Similarly, this procedure yields a particle density of 2.06 g cm−3, which is in excellent agreement with the high-precision measurement of NaNO3 density. The same fit also yields the new 50% detection efficiency point of 83 nm, for SPLAT with the new aerodynamic lens configuration, which is 24% lower than the 109 nm value obtained with the older lens. This indicates a significant increase in SPLAT's detection efficiency of smaller particles with the new configuration.
With this example, we have outlined a new simple method for establishing a detection probability curve for small particles. This method uses a polydisperse aerosol, composed of particles of known density and with known particle size distribution that peaks near the halfway point of the detection probability. Measurement of the vacuum aerodynamic size distribution with good signal-to-noise allows for an accurate fit, which is carried out assuming that can be described with a sharp drop-off detection efficiency function multiplied by the measured mobility size distribution and shifted by the known density. As we show below, once a new curve is obtained it can be tested on another polydisperse aerosol sample of known density.
The Densities of NaNO3 and Ambient Particles
From , the estimated density for the NaNO3 particles based on the simulated curve is 2.06 g cm−3. Alternatively, we can use the newly established halfway point of dp
= 83 nm together with the observed halfway point of
which is 168 nm (shown in ) to yield an estimated density (before correction) of 2.02 g cm−3, which differs by only 2% from the exact value. Considering the relevant error correction curve, we find that the estimated density needs to be increased by 1% to yield a density of 2.04 g cm−3, which is only 1% off the true particle density.
With the new detection curve established based on the NaNO3 particles, the next step is to test how well the analysis works for different particles. The curve measured for ambient laboratory air particles is shown in (black trace), with a fit to the data (red curve). These data were fit with Equation (Equation3), in which the 50% detection point (a in Equation (Equation2)) was fixed at dp
= 83 nm to yield a particle density of 1.38 g cm−3. As with the NaNO3 particles, we measured for these particles their precise density using DMA-classified particles and SPLAT. The results of these measurements for 100 nm, 151 nm, and 200 nm DMA-classified particles are shown in , and yield an effective density of 1.4 g cm−3, in excellent agreement with the density of 1.38 g cm−3 from
. We note that the observed dva
distributions of the monodisperse ambient laboratory particles are rather broad and asymmetric, which is especially true for the larger particles with mobility diameter of 200 nm. This line-shape indicates either that these particles are aspherical (CitationZelenyuk et al. 2008d), in which case the density measurements yield effective particle densities, or that the particles have a range of compositions and hence densities. The asphericity parameters measured for these particles (CitationVaden et al. 2011) indicate that a significant fraction of these particles are aspherical, which is consistent with the line-shape of the smaller particles. The broader line-shapes of larger particles indicates the presence of a mixture of particles with a range of compositions and hence densities.
FIG. 4 (a) Experimentally observed

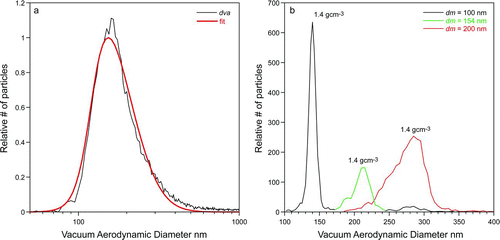
Notable in the case of the polydisperse room-air particles is that on the small-particle side the shape of the mobility size distribution was found to be very different from a log-normal distribution. Yet the fit, used to extract a relatively accurate density, works extremely well despite the fact that it assumes log-normal distribution. The reason is that since the small particle side of is strongly dominated by the SPLAT detection efficiency, the exact shape of the dp
distribution on the small particle side is not that important.
Before we precede to present field data measurements, we apply the simple method of locating the halfway point on the left-edge to the ambient particle data (as opposed to fitting the data with Equation (Equation3)). The observed 50% point is dva = 118 nm yielding an estimated effective density of 1.42 g cm−3. Since the particle size distribution peaks at 110 nm the error correction is −1%, yielding again a density estimate with 1% accuracy.
Application to Field Measurements and the Densities of Composition-Resolved Particles
The density measurements for the DMA-classified ambient laboratory particles presented in the section above indicate that their density was nearly size-independent, making the interpretation of the density calculated from rather straightforward. However, ambient particles can present more complex scenarios, in which the particle compositions, and hence densities, are size-dependent. In these cases, the interpretation of the density calculated from the sharp, small particle
edge is not so obvious.
In , we present data obtained on June 25, 2010 at 10 am during CARES field campaign, when the number concentration of particles larger than 14 nm was ∼5,000 particles cm−3, and the number concentration of particles detectable by SPLAT was 700 particles cm−3. shows that the mobility size distribution peaks at 37 nm and has a second smaller mode at ∼220 nm, providing the first indication that more than one type of particles is present. shows the results of the density measurements of DMA-classified particles with diameters 80, 100, 119, 151, and 200 nm. The figure reveals a simple pattern, in which particle densities increase from 1.3 to 1.6 g cm−3 as the particle size increase from 80 to 200 nm. The line-shapes of the distributions of the monodisperse particles in are also informative. They show that the majority of smallest particles have a density of 1.3 g cm−3, and as the particle size increases the line-shape broadens, indicating that a mix of particles with densities between 1.3 and 1.6 g cm−3 are present, and that the density of most of the 200 nm particles is 1.6 g cm−3.
FIG. 5 (a) Mobility size distribution of particles measured in Sacramento, CA during the CARES field campaign; (b) Density measurements of DMA-classified particles with diameters of 80, 100, 119, 150, and 200 nm; (c) The
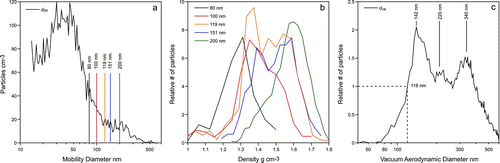
The analysis of individual particle mass spectra and sizes reveals that vast majority of the particles are composed of organics mixed with sulfate and that particles with higher density have larger fraction of sulfate.
is a plot of the measured , showing the characteristic sharp drop-off at the small particle side and three peaks at 142, 220, and 340 nm. The first is most likely due to the drop in detection efficiency, and the third is consistent with the ∼220 nm, minor peak in the mobility size distribution and the measured density of 1.6 g cm−3. The presence of three distinct peaks in
provides an indication that the particle compositions are most likely size-dependent. It is clear that the average particle density calculated from the sharp detection drop-off for this
distribution cannot be representative of all the particles.
shows also that the 50% point is at dva
= 118 nm, which translates to a density of 118/83 = 1.42 g cm−3. For a density correction, we note that the size distribution shows multiple peaks with the most intense peak at 142 nm, which yields a particle size of 142/1.42 = 100 nm, which with the new lens translates to no correction. It is important to point out that despite the fact that the instrument was transported from the laboratory to the field, where it was reassembled, realignment, and recalibrated, the 50% detection point remained at dp
= 83 nm.
Looking at the size-resolved particle densities, shown in , we find that a density of 1.42 g cm−3 is a reasonable representation of the densities of particles that are between 80 and 120 nm—the range of sizes that affect the position and shape of the sharp drop-off we used to calculate the particle density.
This example illustrates the fact that even in complex cases, where the particle densities are size-dependent and the mobility and distributions exhibit multiple peaks, it is possible to obtain useful and relatively accurate average density. It is important to keep in mind that the calculated values represent an average density of particles that are between 80 nm and 120 nm in diameter. When the data provide indication that particle compositions vary with particle size, it is best to apply this method to the
distributions of the chemically resolved data. Below we demonstrate an application of this approach to data collected on one of 27 ISDAC flights.
We apply the data analysis methodology presented above to aerosol populations measured during the ISDAC, in which SPLAT was deployed onboard of an aircraft to continuously sample atmospheric particles. shows the curve of a representative aerosol measurement taken over a few minutes of flight, from which we calculate an average particle density of 1.39 g cm−3, using the sharp drop-off on the left side of the
curve. We can test this calculated density by comparing
to the size distribution measured by the Passive Cavity Aerosol Spectrometer Probe (PCASP) that was also used to monitor the aerosol size distribution during the ISDAC. Because the PCASP cannot detect particles smaller than 100 nm, the density is calculated by comparing the large particle side of the SPLAT and PCASP size distributions to yield 1.4 g cm−3, which is clearly in excellent agreement with the density obtained based on the small particle size estimate.

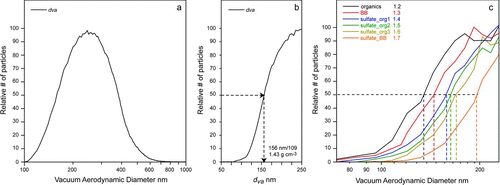
One of the most important features of SPLAT and all other single particle mass spectrometers is their ability to measure simultaneously the vacuum aerodynamic diameter and mass spectrum of each detected particle. This enables the separate and simultaneous characterization of distributions for different types of particles by classifying their mass spectra into a number of classes (CitationZelenyuk et al. 2006b; Citation2008b) and separately obtaining the
distributions of particles that belong to each of these classes. Classification of the mass spectra (not shown) recorded during this flight segment shows that the majority of the particles belong to the following six classes: organics, biomass burning (BB) aerosol, three types of sulfates mixed with organics, and sulfates mixed with BB.
shows the left-hand sides of the distributions obtained for six classes listed above. The density values calculated from each composition-resolved
curves is shown in the figure legend. The density of the organic particles that are mostly composed of the oxygenated organics is found to be 1.2 g cm−3, which is in good agreement with typical SOA particle densities (CitationZelenyuk et al. 2008d) and the density of the oxygenated organics component of atmospheric particles previously measured using high-precision methods (CitationZelenyuk et al. 2008a). The calculated density of BB particles of 1.3 g cm−3 also falls within the 1.2–1.4 g cm−3 range of densities reported for dry biomass burning particles (CitationReid et al. 2005).
The estimated densities of 1.4, 1.5, and 1.6 g cm−3 of the particles composed of organics mixed with sulfates can be used to calculate their corresponding sulfates and organics weight fractions (CitationZelenyuk et al. 2008a). Using densities of 1.8 and 1.2 g cm−3 for sulfate and organics, respectively, we calculate the weight fractions of organics in these three classes to be 56%, 38%, and 22%, respectively. Similarly, the density of particles composed of sulfate mixed with BB whose estimated density is 1.7 g cm−3 indicates that these particles have only 10% BB by mass.
In addition to the six classes discussed above, there were a few dust particles, and small number of fresh and process sea-salt particles. However, the number of particles in these classes was significantly smaller than the ∼1000 particles required to obtain distributions that can be used to estimate particle density.
Conclusions
Typical density measurements require measurement of two different particle properties, which is usually accomplished by two separate instruments. We have shown here that in SPLAT the sharp decrease in detection efficiency of small particles gives rise to a reproducible, distinctive line-shape of the curve. While the sharp drop-off is determined by the particle true diameter, the
curve is a measure of particle vacuum aerodynamic diameter, making it possible to deduce, based on SPLAT data alone, the aerosol density or effective density. The only requirement is that the particle size distribution is sufficiently broad and that it has significant population with diameters close to the sharp detection probability drop-off point.
We have illustrated two different practical methods to take advantage of this feature and to estimate particle density with good precision. In one, the measured SPLAT detection efficiency curve is simulated with a simple function and the overall particle population is assumed to have a log-normal distribution. The measured is then fit as the product of these two functions using the density as one of the fitting parameters. The other, somewhat simpler, approach uses the ratio of particle diameter at the 50% detection efficiency point and the vacuum aerodynamic diameter on the small-particle side where the observed
distribution drops to half of its maximum value, to estimate the particle density. We showed that it is possible to generate an error correction curve to improve the accuracy with which the density is estimated. Both methods were tested with particles of known densities.
The method presented in this article can be utilized by any single particle mass spectrometer that uses particle optical detection. It is suitable for field studies of atmospheric aerosols, which often require high temporal resolution and real-time monitoring. We illustrated the application of this analytical methodology to field data acquired by SPLAT during ground and aircraft-based deployments. The overall density derived for an aerosol sample was found to be in good agreement with the density obtained by other methods. The density analysis of the composition-resolved particles using their distributions alone reveals density values consistent with known values for typical atmospheric particles and was used to quantify the relative fraction of constituents of internally mixed particles. This method is therefore highly useful in complex data analyses that are typically required for interpretation of field measurements.
This article also presents the first data obtained with a new aerodynamic lens inlet that improves the detection efficiency of small particle, making it possible to detect 83 nm particles with 50% efficiency. The improved lens was designed and machined to assure that the positioning of the apertures is reproducible and precise. We have also shown that it is possible to reverse the approach used to measure particle density and deploy polydisperse spherical particles of known density and size distribution to determine the instrument detection efficiency. The application of this method to SPLAT, equipped with the newly designed aerodynamic lens inlet, shows a significantly improved detection efficiency of small particles, as the 50% detection point of the new lens is 83 nm as compared with 109 nm for the older lens.
Acknowledgments
This work was supported by the U.S. Department of Energy (DOE) Office of Basic Energy Sciences, Division of Chemical Sciences, Geosciences, and Biosciences and Office of Biological and Environmental Research (OBER). Part of this research was performed in the Environmental Molecular Sciences Laboratory, a national scientific user facility sponsored by the DOE's OBER at Pacific Northwest National Laboratory (PNNL). PNNL is operated by the U.S. DOE by Battelle Memorial Institute under contract No. DE-AC06-76RL0 1830.
We thank the ISDAC and CARES teams for their incredible help during these field campaigns. ISDAC and CARES were supported by the U.S. DOE Atmospheric Radiation Measurement (ARM) Program Climate Research Facility, the DOE Atmospheric Sciences Program, the National Research Council of Canada and Environment Canada. Some of the data were obtained from the ARM program archive, sponsored by DOE OBER Environmental Science Division.
REFERENCES
- Alfarra , M. R. , Paulsen , D. , Gysel , M. , Garforth , A. A. , Dommen , J. , Prevot , A. S.H. , Worsnop , D. R. , Baltensperger , U. and Coe , H. 2006 . A Mass Spectrometric Study of Secondary Organic Aerosols Formed from the Photooxidation of Anthropogenic and Biogenic Precursors in a Reaction Chamber . Atmos. Chem. Phys. , 6 : 5279 – 5293 .
- Bahreini , R. , Keywood , M. D. , Ng , N. L. , Varutbangkul , V. , Gao , S. , Flagan , R. C. , Seinfeld , J. H. , Worsnop , D. R. and Jimenez , J. L. 2005 . Measurements of Secondary Organic Aerosol from Ooxidation of Cycloalkenes, Terpenes, and M-Xylene using an Aerodyne Aerosol Mass Spectrometer . Environ. Sci. Technol. , 39 : 5674 – 5688 .
- Cai , Y. , Zelenyuk , A. and Imre , D. 2006 . A High Resolution Sstudy of the Effect of Morphology on the Mass Spectra of Single PSL Particles with Na-Containing Layers and Nodules . Aerosol Sci. Technol. , 40 : 1111 – 1122 .
- DeCarlo , P. F. , Slowik , J. G. , Worsnop , D. R. , Davidovits , P. and Jimenez , J. L. 2004 . Particle Morphology and Density Characterization by Combined Mobility and Aerodynamic Diameter Measurements. Part 1: Theory . Aerosol Sci. Technol. , 38 : 1185 – 1205 .
- Hand , J. L. and Kreidenweis , S. M. 2002 . A New Method for Retrieving Particle Refractive Index and Effective Density from Aerosol Size Distribution Data . Aerosol Sci. Technol. , 36 : 1012 – 1026 .
- Hering , S. V. and Stolzenburg , M. R. 1995 . Online Determination of Particle-Size and Density in the Nanometer-Size Range . Aerosol Sci. Technol. , 23 : 155 – 173 .
- Katrib , Y. , Martin , S. T. , Rudich , Y. , Davidovits , P. , Jayne , J. T. and Worsnop , D. R. 2005 . Density Changes of Aerosol Particles as a Result of Chemical Reaction . Atmos. Chem. Phys. , 5 : 275 – 291 .
- Kelly , W. P. and McMurry , P. H. 1992 . Measurement of Particle Density by Inertial Classification of Differential Mobility Analyzer Generated Monodisperse Aerosols . Aerosol Sci. Technol. , 17 : 199 – 212 .
- Malloy , Q. G.J. , Nakao , S. , Qi , L. , Austin , R. , Stothers , C. , Hagino , H. and Cocker , D. R. 2009 . Real-Time Aerosol Density Determination Utilizing a Modified Scanning Mobility Particle SizerAerosol Particle Mass Analyzer System . Aerosol Sci. Technol. , 43 : 673 – 678 .
- McFarquhar , G. M. , Ghan , S. , Verlinde , J. , Alexei , Korolev , J. , Strapp , W. , Schmid , B. , Tomlinson , J. M. , Wolde , M. , Brooks , S. D. , Cziczo , D. , Dubey , M. K. , Fan , J. , Flynn , C. , Gultepe , I. , Hubbe , J. , Gilles , M. K. , Laskin , A. , Lawson , P. , Leaitch , R. , Liu , P. , Liu , X. , Lubin , D. , Mazzoleni , C. , Macdonald , A. M. , Moffet , R. C. , Morrison , H. , Ovchinnikov , M. , Shupe , M. D. , Turner , D. D. , Xie , S. , Zelenyuk , A. , Bae , K. , Freer , M. and Glen , A. 2010 . Indirect and Semi-Direct Aerosol Campaign (ISDAC): The Impact of Arctic Aerosols on Clouds . Bulletin of the American Meteorological Society , doi: doi:10.1175/2010BAMS2935.1
- McMurry , P. H. , Wang , X. , Park , K. and Ehara , K. 2002 . The Relationship between Mass and Mobility for Atmospheric Particles: A New Technique for Measuring Particle Density . Aerosol Sci. Technol. , 36 : 227 – 238 .
- Moffet , R. C. and Prather , K. A. 2005 . Extending ATOFMS Measurements to Include Refractive Index and Density . Anal. Chem. , 77 : 6535 – 6541 .
- Morawska , L. , Johnson , G. , Ristovski , Z. D. and Agranovski , V. 1999 . Relation between Particle Mass and Number for Submicrometer Airborne Particles . Atmos. Environ. , 33 : 1983 – 1990 .
- Murphy , D. M. , Cziczo , D. J. , Hudson , P. K. , Schein , M. E. and Thomson , D. S. 2004 . Particle Ddensity Inferred from Simultaneous Optical and Aerodynamic Diameters Sorted by Composition . J. Aerosol Sci. , 35 : 135 – 139 .
- Pitz , M. , Cyrys , J. , Karg , E. , Wiedensohler , A. , Wichmann , H. E. and Heinrich , J. 2003 . Variability of Apparent Particle Density of an Urban Aerosol . Environ. Sci. Technol. , 37 : 4336 – 4342 .
- Reid , J. S. , Koppmann , R. , Eck , T. F. and Eleuterio , D. P. 2005 . A Review of Biomass Burning Emissions. Part II: Pntensive Physical Properties of Biomass Burning Particles . Atmos. Chem. Phys. , 5 : 799 – 825 .
- Salcedo , D. , Onasch , T. B. , Dzepina , K. , Canagaratna , M. R. , Zhang , Q. , Huffman , J. A. , DeCarlo , P. F. , Jayne , J. T. , Mortimer , P. , Worsnop , D. R. , Kolb , C. E. , Johnson , K. S. , Zuberi , B. , Marr , L. C. , Volkamer , R. , Molina , L. T. , Molina , M. J. , Cardenas , B. , Bernabe , R. M. , Marquez , C. , Gaffney , J. S. , Marley , N. A. , Laskin , A. , Shutthanandan , V. , Xie , Y. , Brune , W. , Lesher , R. , Shirley , T. and Jimenez , J. L. 2006 . Characterization of Ambient Aerosols in Mexico City during the MCMA-2003 Campaign with Aerosol Mass Spectrometry: Results from the CENICA Supersite . Atmos. Chem. Phys. , 6 : 925 – 946 .
- Schleicher , B. , Kunzel , S. and Burtscher , H. 1995 . In-Situ Measurement of Size and Density of Submicron Aerosol-Particles . J. Appl. Phys. , 78 : 4416 – 4422 .
- Spencer , M. T. , Shields , L. G. and Prather , K. A. 2007 . Simultaneous Measurement of the Effective Density and Chemical Composition of Ambient Aerosol Particles . Environ. Sci. Technol. , 41 : 1303 – 1309 .
- Stein , S. W. , Turpin , B. J. , Cai , X. P. , Huang , C. P.F. and Mcmurry , P. H. 1994 . Measurements of Relative Humidity-Dependent Bounce and Density for Atmospheric Particles Using the Dma Impactor Technique . Atmos. Environ. , 28 : 1739 – 1746 .
- Tang , I. N. and Munkelwitz , H. R. 1994 . Water Activities, Densities, and Refractive Indexes of Aqueous Sulfates and Sodium-Nitrate Droplets of Atmospheric Importance . J. Geophys. Res., [Atmos.]. , 99 : 18801 – 18808 .
- Vaden , T. D. , Imre , D. , Beranek , J. and Zelenyuk , A. 2011 . Extending the Capabilities of Single Particle Mass Spectrometry. Part I: Measurements of Aerosol Number Concentration, Size Distribution, and Asphericity . Aerosol Sci. Technol. , 45 : 113 – 124 .
- Vaden , T. D. , Song , C. , Zaveri , R. A. , Imre , D. and Zelenyuk , A. 2010 . Morphology of Mixed Primary and Secondary Organic Particles and the Adsorption of Spectator Organic Gases during Aerosol Formation . Proc. Natl Acad. Sci. , 107 : 6658 – 6663 .
- Virtanen , A. , Ristimaki , J. and Keskinen , J. 2004 . Method for Measuring Effective Density and Fractal Dimension of Aerosol Agglomerates . Aerosol Sci. Technol. , 38 : 437 – 446 .
- Zelenyuk , A. , Cai , Y. , Chieffo , L. and Imre , D. 2005 . High Precision Density Measurements of Single Particles: The Density of Metastable Phases . Aerosol Sci. Technol. , 39 : 972 – 986 .
- Zelenyuk , A. , Cai , Y. and Imre , D. 2006a . From Agglomerates of Spheres to Irregularly Shaped Particles: Determination of Dynamic Shape Factors from Measurements of Mobility and Vacuum Aerodynamic Diameters . Aerosol Sci. Technol. , 40 : 197 – 217 .
- Zelenyuk , A. , Ezell , M. J. , Perraud , V. , Johnson , S. N. , Bruns , E. A. , Yu , Y. , Imre , D. , Alexander , M. L. and Finlayson-Pitts , B. J. 2010 . Characterization of Organic Coatings on Hygroscopic Salt Particles and Their Atmospheric Impacts . Atmos. Environ. , 44 : 1209 – 1218 .
- Zelenyuk , A. and Imre , D. 2005 . Single Particle Laser Ablation Time-of-Flight Mass Spectrometer: An Introduction to SPLAT . Aerosol Sci. Technol. , 39 : 554 – 568 .
- Zelenyuk , A. and Imre , D. 2007 . On the Effect of Particle Alignment in the DMA . Aerosol Sci. Technol. , 41 : 112 – 124 .
- Zelenyuk , A. and Imre , D. 2009 . Beyond Single Particle Mass Spectrometry: Multidimensional Characterisation of Individual Aerosol Particles . International Reviews in Physical Chemistry. , 28 : 309 – 358 .
- Zelenyuk , A. , Imre , D. , Cai , Y. , Mueller , K. , Han , Y. P. and Imrich , P. 2006b . SpectraMiner, an Interactive Data Mining and Visualization Software for Single Particle Mass Spectroscopy: A Laboratory Test Case . Intl J. Mass Spectrom. , 258 : 58 – 73 .
- Zelenyuk , A. , Imre , D. , Cuadra-Rodriguez , L. A. and Ellison , B. 2007 . Measurements and Interpretation of the Effect of a Soluble Organic Surfactant on the Density, Shape, and Water Uptake of Hygroscopic Particles . J. Aerosol Sci. , 38 : 903 – 923 .
- Zelenyuk , A. , Imre , D. , Han , J. H. and Oatis , S. 2008a . Simultaneous Measurements of Individual Ambient Particle Size, Composition, Effective Density, and Hygroscopicity . Anal. Chem. , 80 : 1401 – 1407 .
- Zelenyuk , A. , Imre , D. , Nam , E. J. , Han , Y. P. and Mueller , K. 2008b . ClusterSculptor: Software for Expert-Steered Classification of Single Particle Mass Spectra . Intl. J. Mass Spectrom. , 275 : 1 – 10 .
- Zelenyuk , A. , Yang , J. , Choi , E. and Imre , D. 2009a . SPLAT II: An Aircraft Compatible, Ultra-Sensitive, High Precision Instrument for In-Situ Characterization of the Size and Composition of Fine and Ultrafine Particles . Aerosol Sci. Technol. , 43 : 411 – 424 .
- Zelenyuk , A. , Yang , J. and Imre , D. 2009b . Comparison between Mass Spectra of Individual Organic Particles Generated by UV Laser Ablation and in the IR/UV Two-Step Mode . Intl. J. Mass Spectrom. , 282 : 6 – 12 .
- Zelenyuk , A. , Yang , J. , Imre , D. and Choi , E. 2009c . Achieving Size Independent Hit-Rate in Single Particle Mass Spectrometry . Aerosol Sci. Technol. , 43 : 305 – 310 .
- Zelenyuk , A. , Yang , J. , Song , C. , Zaveri , R. A. and Imre , D. 2008c . “Depth-Profiling” and Quantitative Characterization of the Size, Composition, Shape, Density, and Morphology of Fine Particles with SPLAT, a Single-Particle Mass Spectrometer . J. Phys. Chem. A , 112 : 669 – 677 .
- Zelenyuk , A. , Yang , J. , Song , C. , Zaveri , R. A. and Imre , D. 2008d . A New Real-Time Method for Determining Particles’ Sphericity and Density: Application to Secondary Organic Aerosol Formed by Ozonolysis of Alpha-Pinene . Environ. Sci. Technol. , 42 : 8033 – 8038 .