Abstract
Carbon nanotubes (CNTs) are being used in many applications and filtration of airborne CNTs is very important for CNT control. Penetration of airborne multi-wall CNTs through a screen filter is studied using a numerical model and the results are compared to experiments. The flow through the screen filter is simulated using a three-dimensional model, and the particle capture due to diffusion, interception and inertial impaction are covered. The length and diameter of CNTs are determined from electron microscopic images and used in the model. In the filtration experiments, the challenging CNTs are classified by a differential mobility analyzer. Therefore we compute the drag force and diffusion coefficient for CNTs based on their mobility sizes. The effects of CNT rotation and orientation on the drag, diffusion coefficient and interception length are considered in the sense of average over a large number of particles. Both the modeling and experimental results show that the CNT penetration is less than the penetration for a sphere with the same mobility diameter, which is mainly due to the larger interception length of the CNTs. The modeling results for CNTs agree reasonably well with experiments when the mobility diameter is less than 250 nm, but significantly underestimate the penetration when the mobility diameter is larger than 250 nm. The discrepancy is attributed to possible curling and bending of the longer CNTs in the flow.
1. Introduction
Carbon nanotubes (CNTs) are being used in structural composites for sporting equipment, conductive plastics, electron field emitters, semiconductor devices, etc. A limited number of studies have been devoted to the transport and filtration of CNTs in aqueous solutions through porous media (CitationJaisi et al. 2008; CitationJaisi and Elimelech 2009; CitationLiu et al. 2009). However, studies so far on the filtration of CNTs in air where they have high mobility are scarce (CitationSeto et al. 2010).
We have experiences in aerosolizing CNTs for toxicological studies (CitationKim et al. 2010). We have developed dispersion techniques using electrospray to generate airborne CNTs with controlled degree of agglomeration. Large amount of CNTs can be generated for testing of high efficiency filters. SEM images of multi-wall CNTs from our electrospray show that these CNTs have diameters about 85 nm and lengths from 2 to 10 μm, which are similar to the dimensions of asbestos fibers. Such similarity has raised concerns that CNTs may cause negative health effects similar to asbestos fibers (CitationPoland et al. 2008).
Significant research has been performed on filtration of asbestos and fibrous aerosols (CitationFeigley 1975; CitationSpurny 1986; CitationGallily et al. 1986; CitationGentry et al. 1989; CitationGradoń et al. 1988, Citation1991; CitationGradoń and Podgórski 1990; CitationFeigley and Chen 1992; CitationMyojo 1999; CitationCheng et al. 2006; CitationWebber et al. 2007; CitationVallero et al. 2009). CitationFeigley (1975) modified the classical filtration theory for spherical particles to develop a model for deposition of fibrous particles in a fibrous filter. The orientation of fibrous particles during motion was assumed to be random. Then the average angle ψ between the fiber axis and its direction of motion can be obtained. An aerodynamic radius Rae was defined for fibrous particles based on their sizes and the angle ψ. This radius Rae is then used as an effective radius to compute the diffusion coefficient, Peclet number and Stokes number for fibrous particles. Finally the deposition efficiencies due to diffusion, interception and impaction were computed following the classical filtration for spherical particles. CitationSpurny (1986) performed experiments to measure collection efficiencies of asbestos fibers in Nuclepore and Millipore filters. CitationGallily and Eisner (1979) and Galliy and Cohen (1979) studied deposition of elongated particles on different obstacles in laminar flows. CitationGallily et al. (1986) calculated the trajectory of cylindrical particles past a long circular cylinder or a Nuclepore hole, and compared their results to experiments. Both the translational and rotational motions of the cylindrical particles were considered. They found pronounced rotation and a tendency of alignment on a time basis along the flow lines for the cylindrical particles. Their experiments were performed in a silicon oil using particles of the order of millimeters. CitationGentry et al. (1989) focused on collection efficiencies of asbestos fibers in Nuclepore filters and considered the collection of fibers in the straight pore theoretically. The interception efficiency was computed under the assumption that the fibers rotated freely in the pores. They showed that the collection efficiencies in experiments were lower than would be expected for the fibers rotating freely in the pores, which they attributed to preferable orientation and alignment when the fibers entered the pores. Gradoń et al. (1988) analyzed the transverse and rotary motions of an ellipsoid in the flow near a cylinder and computed the deposition efficiency for the effect of interception, inertia and sedimentation. CitationGradoń and Podgórski (1990) extended the analysis to perfectly flexible fibrous particles. They found that the deposition efficiency of stiff elongated particles is greater than spherical (compact) ones with the same mass, because the elongated particles are more likely to be intercepted. However, they stated that flexible elongated particles are difficult to be removed by the filter element, because they deform and align with the streamline and can pass by the filter element. CitationMyojo (1999) performed Monte Carlo simulation for fibers penetrating mesh screens with several simplifications, including neglecting the flow disturbance by the screen wires and inertia of the fibers. He also performed experiments and developed a method to determine the length distribution of the fibers based on the penetration results. CitationFeigley and Chen (1992), CitationCheng et al. (2006), CitationWebber et al. (2007), and CitationVallero et al. (2009) focused on measurement of asbestos collection on different filters and occupational safety aspect. These studies demonstrated that the fiber dimension and filter pore size were important for the filtration efficiency.
Another related topic is the filtration of nanoparticle agglomerates, since chain-type agglomerates have similar morphology with CNTs. CitationFu et al. (1990) studied the filtration of iron oxide chain aggregate aerosols using a screen type filter, which is the same diffusion battery screen as used in the present study. Fu et al. compared their results with a model for the chain agglomerates adapted from the classical filtration theory for spherical particles. The interception parameter in the model for chain agglomerates was unknown, and was determined by matching the model results with experimental ones. Fu et al. used the interception parameter to estimate the orientation of agglomerates in the flow. They found that interception was the dominant deposition mode and that the chain agglomerates were preferentially aligned with the flow at the higher flow rates. CitationLange et al. (1999) measured the filtration efficiency of fibrous filters for carbon agglomerates and for spherical particles as a function of the mobility diameter. They derived a filtration model based on the interception equivalent diameter of agglomerates, which is defined as the diameter of a spherical particle diameter having the same penetration as that of the agglomerate. Kim, S.C. et al. (2009) studied filtration of silver nanoparticle agglomerates by fibrous filters. They found that agglomerate particles showed lower penetration due to their larger interception length compared with spherical particles with the same mobility diameters.
We carried out experiments to study CNT penetration through a screen filter, which was a diffusion battery screen. We performed numerical simulations of the experiments to better understand the flow field in the filter and the capture mechanisms of CNTs including diffusion, interception and inertial impaction. The length and diameter of CNTs were measured using electron microscopy and directly used in the numerical model, and we compared the experimental and numerical results without using fitting parameters.
2. Experimental Results
The experimental setup and results for CNT penetration are described here to facilitate the comparison with numerical modeling. The filtration system consisted of a CNT generation system, a size classification system and a penetration measurement system. Multi-wall CNTs (MWCNTs) manufactured by Bayer Material Sciences were used in the experiments. The CNTs were dispersed into airborne form using a high through-put electrospray (CitationKim et al. 2010). The CNTs were then classified by a Differential Mobility Analyzer (DMA, TSI 3081). The selected CNTs with the same electrical mobility size then went through a Po-210 neutralizer and were used to challenge the filter. The concentrations upstream and downstream of the filter were measured by a Condensation Particle Counter (CPC). Then the fractional penetration through the filter was obtained.
The electrosprayed MWCNTs can be characterized by different methods. CitationKim et al. (2010) measured the electrosprayed MWCNTs using a Scanning Mobility Particle Sizer (SMPS) and showed that the size distribution had peaks around 150 nm and 400 nm, even though the geometric lengths were 2 to 10 μm. Similar results have been observed by CitationSeto et al. (2010). We utilized the DMA to select CNTs of a certain mobility diameter, deposited them on a grid, then analyzed them using Scanning Electron Microscopy (SEM, ). A large number of CNTs were measured and the length distribution was fitted into a lognormal distribution. The mean length of the CNTs was then obtained for the corresponding mobility diameter. shows the CNT number count versus the geometric length for mobility diameters of 200, 300 and 400 nm. Our results showed that the mean CNT length was 2300 nm, 4300 nm, and 6100 nm when the CNT mobility diameter was 200 nm, 300 nm, and 400 nm, respectively. The diameter of the CNTs was dCNT = 85 nm in all cases. CitationSeto et al. (2010) showed that the fractions of multiply charged particles were 29% and 20% for 200 and 300 nm CNTs, respectively. Our CNT length distributions () had distinctively single modes, thus we believe the fractions of multiply charged particles in our study were relatively low.
FIG. 2 The CNT number count versus the geometric length measured from SEM images for mobility diameters of 200, 300, and 400 nm. The curves represent lognormal fitting for the data of each mobility size.
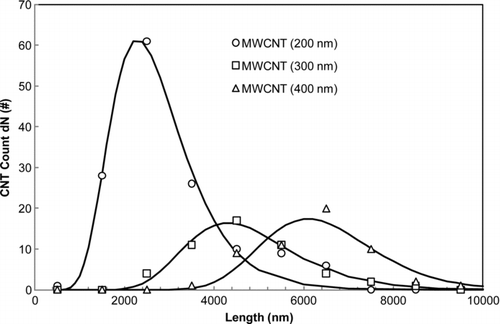
The testing filters were screens made of 635-mesh type 304 stainless steel, which were used in the diffusion battery (TSI, model 3040). The screen wire was 20 μm in diameter and the opening dimension was also 20 μm (). The main reason we chose the wire mesh screen was that it had a regular structure with well-defined wire diameter and opening dimension. We avoided complications caused by inhomogeneity, variable pore sizes, etc, which are common in fibrous filters. CitationSinclair et al. (1979) measured penetration through the screen-type diffusion battery using monodisperse NaCl and DOP particles of 0.015 to 0.11 μm in diameter. CitationCheng and Yeh (1980) developed explicit equations for penetration through the screen-type diffusion battery and showed good agreement with the experiments. Cheng and Yeh measured the screen solidity and obtained α = 0.345, where the solidity α is defined as
FIG. 3 SEM images of the stainless steel screen used in the TSI diffusion battery, (a) front view, (b) slightly tilted view.
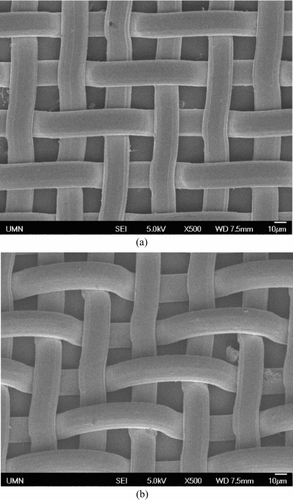
Penetration of CNTs and Polystyrene Latex (PSL) particles was measured through 20 layers of the diffusion screens. Since the filtration efficiency of a single screen was very low, 20 layers were used to improve the measurement statistics. PSL particles were used to provide the control data set. shows the measured penetration values of CNTs and PSL spheres as functions of the mobility size. When the mobility size was above 100 nm, the penetration of CNTs was considerably lower than that of PSL spheres. Interception was the main mechanism that gave this difference, which we will discuss in detail in Section 4. Since penetration is an exponential function of the number of layers of screens (CitationCheng and Yeh 1980), we compute the penetration through a single layer of the screen P 1 as
3. Numerical Methods
We have developed a numerical model for fibrous filtration using the computational fluid dynamics code FLUENT. The air flow inside the filter is first simulated, then the particle motion is considered and the filtration efficiency is obtained. We have used the model to study filtration by elliptical fibers and nanofiber filters (CitationWang et al. 2008a, Citationb; CitationWang and Pui 2009). The basic numerical method is extended here to simulated CNT penetration in the screen filter.
The screen is composed of repeating sections which are cross-shaped. A three-dimensional model including two cylinders with 20 μm diameter and 40 μm height is created to simulate the cross-shaped section (). The two cylinders are placed perpendicularly and the gap between them is 5 μm. The gap is chosen so that the solidity of the model is 0.349, close to the value 0.345 measured by CitationCheng and Yeh (1980). The simulation domain is a long box with a 40 μm by 40 μm cross-section and the two cylinders are placed at the center of the domain. The inlet of the flow is 200 μm upstream to the first cylinder and a uniform velocity profile is prescribed so that
FIG. 5 The three-dimension model for simulation of the cross-shaped section in the screen filter. The two cylinders have 20 μm diameter and 40 μm height; they two cylinders are placed perpendicularly.
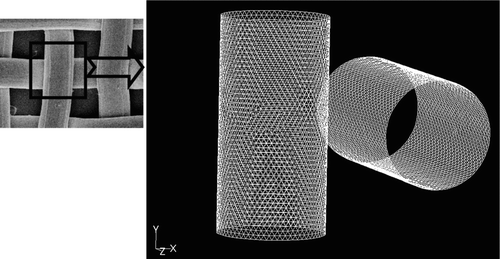
The flow field is obtained by solving the steady-state incompressible Navier-Stokes equations. Vector plots for the air flow passing the two cylinders are shown in . The arrows represent the local flow velocities and the lengths of the arrows are proportional to the velocity magnitude. It can be seen that the velocities are close to zero near the cylinder surfaces; the velocity magnitude is largest at the symmetric planes. Contour plots for the pressure in the simulation domain are shown in . It can be seen that the pressure drop occurs mainly near the two cylinders. The pressure drop Δp, computed as the difference between the average pressure values at the inlet and outlet, is 4.65 Pa from the simulation. We can calculate the pressure drop using the expression given by CitationDavies (1973)
FIG. 6 Vector plots for the air flow passing the two cylinders. The arrows represent the local flow velocities and the lengths of the arrows are proportional to the velocity magnitude.
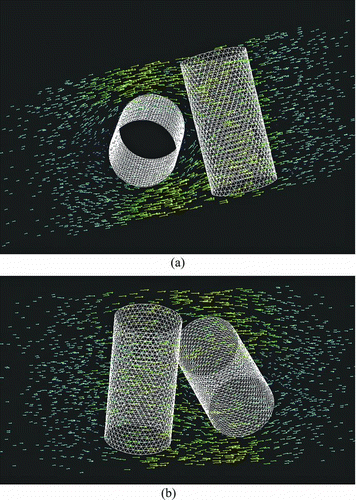
Mechanical mechanisms for particle capture include diffusion, interception and inertial impaction. Numerical study of the particle capture due to diffusion may be carried out by solving the convective diffusion equation
FIG. 8 Contour plot for the concentration of 50 nm particles from the calculation for the diffusion effect.
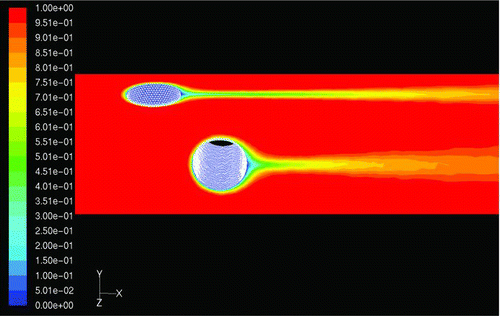
To simulate particle capture due to interception and inertial impaction, we add particles to the flow using the Discrete Phase Model of FLUENT. This model calculates the trajectories of particles using a Lagrangian formulation that includes the particle inertia and hydrodynamic drag. The governing equation of the particle motion is
4. Numerical Results for CNT Penetration through a Screen Filter
The length, diameter, and mass of CNTs need to be determined for the filtration simulation. The CNTs lengths were determined from SEM images as described in Section 2. The mean CNT length was 2300 nm, 4300 nm, and 6100 nm when the CNT mobility diameter was 200 nm, 300 nm, and 400 nm, respectively. CitationKim et al. (2007) considered the relation between the geometric length and electrical mobility diameter of nanowires and nanotubes. They derived an expression to show
FIG. 9 Linear fitting for the CNT length and the CNT mobility diameter divided by the slip correction factor.
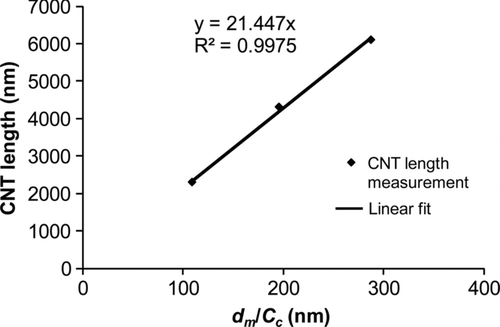
where e is the elementary charge and n is the number of electrical charges on the particle. If we assume that the CNT and a spherical particle pass through the DMA with the same electrical charges on them, then the drag on the CNT in the DMA is the same as that on a sphere with equivalent mobility size. The drag can be computed as


The diffusion coefficient D of CNTs is needed when solving the convective diffusion Equation (Equation7). The value of D is tied to the drag force
It is assumed that contact between any point on a CNT and a wire in the screen filter leads to interception. We need to consider the effect of CNT length and orientation on interception. The orientations of CNTs are random before they approach the wires. Following Gradoñ et al. (1988), we consider rotation of the CNT near the wire surface using the results of CitationJeffery (1922) for an ellipsoid in a field with a constant velocity gradient G. Jeffery's results show that the particle rotates periodically with a rotation period Tr ,
We consider the interception under the assumption that orientations of CNTs are random before they approach the wires and the effect of rotation of the CNTs near the wires is negligible. The problem is simplified as the relative position between a randomly oriented CNT and a plane. Let θ be the angle between the CNT and the plane and ϕ be the azimuth. If the distance from the CNT center to the plane is less than LCNT /2 × sinθ, the CNT is intercepted by the plane. Since θ can be any arbitrary angle between 0 and π/2, the average interception length for a CNT with random orientation is
CitationSeto et al. (2010) performed experiments to study filtration of MWCNTs by fibrous filters at several filtration velocities. They observed that the CNTs with 300 nm mobility diameter had considerably lower penetration than spherical particles of the same mobility size, which agreed with our results. Seto et al. compared their experimental results with the single-fiber efficiency from classical filtration theories and concluded that an orientation angle, θ, was needed to match the experimental and modeling results. By fitting the experimental data, they obtained the angle between 40 and 60 degrees and it decreased with increasing particle size and increasing filtration velocity. In our study, the orientation angle corresponding to sinθ = 0.6366 is approximately 40 degree. If the rotational effect of the CNTs near the wire is considered, the effective orientation angle is expected to increase because the orientations with large angles are more probable to contribute to interception during rotation. Thus we believe the orientation angle obtained here is in reasonable agreement with those in CitationSeto et al. (2010).
We perform simulation for CNT penetration through one layer of the diffusion screen and the results are compared to the experimental ones as shown in . Simulation is also carried out for spherical PSL particles and the agreement between the model and experiments is excellent. This demonstrates that our numerical methods described in Section 3 are sound. Our numerical model does not involve interaction between diffusion and interception. We used the same approach to simulate penetration through a composite filter of nanometer and micrometer fibers (CitationWang et al. 2008a), where we observed very good agreement between the modeling and experimental results for compact particles from 20 to 780 nm. The results demonstrate that in the above cases, the contribution of the interaction between diffusion and interception to the total filtration efficiency is rather small. For the CNTs, the filtration efficiency due to interaction between Brownian diffusion and interception can be larger, at the same time the filtration efficiency due to interception is also larger, thus the contribution due to the interaction compared to the overall filtration efficiency may not necessarily be higher when CNTs are compared to compact particle. Further studies are needed to accurately assess the effect of omitting the interaction term.
FIG. 10 Penetrations of PSL particles and CNTs through one layer of the diffusion screen as functions of the mobility size. Modeling and experimental results are compared.
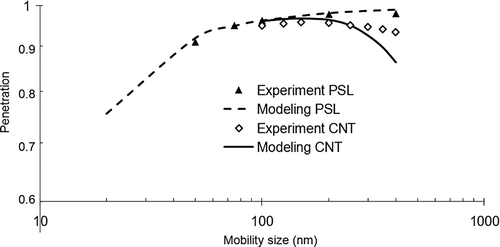
For the CNTs, the modeling results agree reasonably well with experiments when dm < 250 nm, but significantly underestimate the penetration when dm > 250 nm. Both the modeling and experimental results show that the CNT penetration is less than the penetration for a sphere with the same mobility diameter, which is mainly due to the larger interception length of the CNTs. One reason for underestimation of the penetration of longer CNTs (dm > 250 nm) may be that they curl or bend in the flow, which makes their effective interception length smaller and easier to penetrate the filter. shows examples of SEM images of CNTs. Both straight and curled CNTs exist. Gradoñ and Podgórski (1990) showed that perfectly flexible elongated particles can deform and align with the streamline and pass by the filter element. Our CNTs are not perfectly flexible but the longer CNTs may bend in the flow and the results agree qualitatively with Gradoñ and Podgórski (1990).
5. Conclusions
We develop a three-dimensional numerical model to simulate the penetration experiments of multi-wall CNTs through a screen filter. Detailed data of the flow field passing by an element in the screen filter are obtained. Capture mechanisms including diffusion, interception and inertial impaction are simulated and the results for spherical particles agree very well with experiments using PSL particles. We compute the penetration for CNTs with specified mobility sizes since the challenging CNTs in the experiments are classified by a DMA. The length, diameter and mass of the CNTs are determined as functions of the mobility size based on experimental data. The drag force and diffusion coefficient for CNTs are computed based on their mobility sizes. Since the orientations of long CNTs (dm > 213 nm) in the DMA and in the filter are different, the drag force on the long CNTs in the filter is larger than that in the DMA, and the diffusion coefficient in the filter is smaller than that in the DMA. The interception length is determined based on the CNT length and the random angle between the CNT and the filter wire surface. Simulation results show that the CNT penetration is less than that for a sphere with the same mobility diameter, in agreement with experiments. Our simulation does not take the flexibility of CNTs into account and considers them as stiff and straight particles. This seems to be valid for short CNTs (dm < 250 nm) as the modeling results agree reasonably well with the experiments in this range. However, long CNTs (dm > 250 nm) may curl and bend in the flow, which can explain the higher penetration in the experiments than predicted by the model.
Acknowledgments
The work was partially supported by the NSF grant (Award ID: 0646236) on “Experimental and Numerical Simulation of the Fate of Airborne Nanoparticles from a Leak in a Manufacturing Process to Assess Worker Exposure.” The authors thank the support of members of the Center for Filtration Research: 3M Corporation, Boeing Company, Cummins Filtration Inc., Donaldson Company, Inc., Entegris Inc, Hollingsworth & Vose Company, Samsung Semiconductor Inc., Shigematsu Works CO., LTD, TSI Inc., and W. L. Gore & Associates and the affiliate member National Institute for Occupational Safety and Health (NIOSH).
References
- Cheng , Y. S. , Holmes , T. D. and Fan , B. 2006 . Evaluation of Respirator Filters for Asbestos Fibers. . J. Occup. Environ. Hyg. , 3 ( 1 ) : 26 – 35 .
- Cheng , Y. S. and Yeh , H. C. 1980 . Theory of a Screen-Type Diffusion Dattery. . J. Aerosol Sci. , 11 : 313 – 320 .
- Davies , C. N. 1973 . Air Filtration , 36 London : Academic Press .
- Feigley , C. E. and Chen , H. C. 1992 . Penetration of Several Filter Materials by Asbestos as a Function of Fiber Dimensions. . Am. Ind. Hyg. Assoc. J. , 53 ( 12 ) : 767 – 772 .
- Feigley , C. E. 1975 . A Mathematical Model for Deposition of Asbestos in Fibrous Filter. . Clean Air. , 9 ( 4 ) : 67 – 71 .
- Fu , T. H. , Cheng , M. T. and Shaw , D. T. 1990 . Filtration of Chain Agglomerate Aerosols by Model Screen Filter. . Aerosol Sci. Technol. , 13 : 151 – 161 .
- Fuchs , N. A. 1964 . The Mechanics of Aerosols. , 40 New York : Pergamon Press .
- Gallily , I. and Cohen , A. 1979 . On the Orderly Nature of the Motion of Nonspherical Aerosol Particles II. Inertial Collision between Spherical Large Droplet and an Axially Symmetrical Elongated Particle. . J. Colloid Interface Sci. , 68 : 338 – 356 .
- Gallily , I. and Eisner , A. D. 1979 . On the Orderly Nature of theMotion of Nonspherical Aerosol Particles I. Deposition froma Laminar Flow. . J. Colloid Interface Sci. , 68 : 320 – 337 .
- Gallily , I. , Schiby , D. , Cohen , A. H. , Hollander , W. and Schless , D. 1986 . On the Inertial Separation of Nonspherical Aerosol Particles from Laminar Flows. I. The Cylindrical Case. . Aerosol Sci. Tech. , 5 : 267 – 286 .
- Gentry , G. W. , Spurny , K. and Schörmann , D. 1989 . Collection Efficiency of Ultrafine Asbestos Fibers Experimental and Theory. . Aerosol Sci. Technol. , 9 : 184 – 195 .
- Gradoń , L. and Podgórski , A. 1990 . Flexible Fibrous Particle Behaviour in the Carrier Gas Flow around Cylindrical Obstacle. . Chem. Eng. Sci. , 45 ( 12 ) : 3435 – 3441 .
- Gradoń , L. , Podgórski , A. and Grzybowski , P. 1991 . Deposition of Flexible and Stiff Fibrous Particles. . J. Aerosol Sci. , 20 ( 8 ) : 971 – 974 .
- Gradoń , L. , Grzybowski , P. and Piłaciński , W. 1988 . Analysis of Motion and Deposition of Fibrous Particles on a Single Filter Element. . Chem. Eng. Sci. , 43 ( 6 ) : 1253 – 1259 .
- Jaisi , D. P. and Elimelech , M. 2009 . Single-Walled Carbon Nanotubes Exhibit Limited Transport in Soil Columns. . Environ. Sci. Technol. , 43 ( 24 ) : 9161 – 9166 .
- Jaisi , D. P. , Saleh , N. B. , Blake , R. E. and Elimelech , M. 2008 . Transport of Single-Walled Carbon Nanotubes in Porous Media: Filtration Mechanisms and Reversibility. . Environ. Sci. Technol. , 42 ( 22 ) : 8317 – 8323 .
- Jeffery , G. B. 1922 . The Motion of Ellipsoidal Particles Immersed in a Viscous Fluid. . Proc. R. Soc. A. , 102 ( 715 ) : 161 – 179 .
- Kim , S. C. , Chen , D. R. , Qi , C. , Gelein , R. M. , Finkelstein , J. N. , Elder , A. , Bentley , K. , Oberdorster , G. and Pui , D. Y. H. 2010 . A Nanoparticle Dispersion Method for in Vitro and in Vivo Nanotoxicity Study. . Nanotoxicology. , 4 ( 1 ) : 42 – 51 .
- Kim , S. C. , Wang , J. , Emery , M. , Shin , W-G. , Mullholand , G. and Pui , D. Y. H. 2009 . Structural Property Effect of Nanoparticle Agglomerates on Particle Penetration through Fibrous Filter. . Aerosol Sci. Technol. , 43 ( 4 ) : 344 – 355 .
- Kim , S. H. , Mulholland , G. W. and Zachariah , M. R. 2009 . Density Measurement of Size Selected Multiwalled Carbon Nanotubes by Mobility-Mass Characterization . Carbon. , 47 ( 5 ) : 1297 – 1302 .
- Kim , S. H. , Mulholland , G. W. and Zachariah , M. R. 2007 . Understanding Ion-Mobility and Transport Properties of Aerosol Nanowires. . J. Aerosol Sci. , 38 ( 8 ) : 823 – 842 .
- Lange , R. , Fissan , H. and Schmidt-Ott , A. 1999 . Predicting the Collection Efficiency of Agglomerates in Fibrous Filter. . Particle Particle Systems Characterization. , 16 ( 2 ) : 60 – 65 .
- Liu , X. , O'Carroll , D. M. , Petersen , E. J. , Huang , Q. and Anderson , C. L. 2009 . Mobility of Multiwalled Carbon Nanotubes in Porous Media. . Environ. Sci. Technol. , 43 ( 21 ) : 8153 – 8158 .
- Myojo , T. 1999 . A Simple Method to Determine the Length Distribution of Fibrous Aerosols. . Aerosol Sci. Technol. , 30 ( 1 ) : 30 – 39 .
- Poland , C. A. , Duffin , R. , Kinloch , I. , Maynard , A. , Wallace , W. A. H. , Seaton , A. , Stone , V. , Brown , S. , MacNee , W. and Donaldson , K. 2008 . Carbon Nanotubes Introduced into the Abdominal Cavity of Mice Show Asbestos-like Pathogenicity in a Pilot Study. . Nature Nanotechnol. , 3 : 423 – 428 .
- Seto , T. , Furukawa , T. , Otani , Y. , Uchida , K. and Endo , S. 2010 . Filtration of Multi-Walled Carbon Nanotube Aerosol by Fibrous Filters. . Aerosol Sci. Technol. , 44 ( 9 ) : 734 – 740 .
- Sinclair , D. , Countess , R. J. , Liu , B. Y. H. and Pui , D. Y. H. 1979 . Aerosol Measurement , Edited by: Lundgren , D. A. , Lippmann , M. , Harris , F. S. Jr. , Clark , W. E. , Marlow , W. H. and Durham , M. D. 544 Gainesville : University Presses of Florida .
- Spurny , K. R. 1986 . On the Filtration of Fibrous Aerosols. . Sci. Total Environ. , 52 : 189 – 199 .
- Vallero , D. A. , Kominsky , J. R. , Beard , M. E. and Crankshaw , O. S. 2009 . Efficiency of Sampling and Analysis of Asbestos Fibers on Filter Media: Implications for Exposure Assessment. . J. Occup. Environ. Hyg. , 6 ( 1 ) : 62 – 72 .
- Wang , J. and Pui , D. Y. H. 2009 . Numerical Investigation of Filtration by Fibers with Elliptical Cross-sections. . J. Nanoparticle Res. , 11 ( 1 ) : 185 – 196 .
- Wang , J. , Kim , S. K. and Pui , D. Y. H. 2008a . Investigation of the Figure of Merit for Filters with a Single Nanofiber Layer on a Substrate. . J. Aerosol Sci. , 39 : 323 – 34 .
- Wang , J. , Kim , S. K. and Pui , D. Y. H. 2008b . Figure of Merit of Composite Filters with Micrometer and Nanometer Fibers. . Aerosol Sci. Technol. , 42 ( 9 ) : 722 – 728 .
- Webber , J. S. , Czuhanich , A. G. and Carhart , L. J. 2007 . Performance of Membrane Filters Used for TEM Analysis of Asbestos. . J. Occup. Environ. Hyg. , 4 ( 10 ) : 780 – 789 .