Abstract
A humidity control system was operated upstream of two collocated MOUDIs (micro-orifice uniform deposit impactors) for sampling ambient aerosol particles. One MOUDI used silicone-grease-coated aluminum foils (ALs) as the impaction substrates and was considered as the reference impactor, while the other used uncoated ALs or uncoated Teflon filters (TFs) as the impaction substrates for quantifying the effect of different relative humidities (RHs) and impaction substrates on the PM0.1 concentrations and mass distributions of ambient PMs. Test results showed that decreasing RH in general increased particle bounce from uncoated substrates with the bounce from uncoated ALs being more severe than that from uncoated TFs. Particle bounce did not influence the overall mass distribution of ambient fine particles when RH ranged between 40% and 80%, whereas it led to undersampling of particles greater than 2.5 μm in aerodynamic diameter severely. Oversampling of PM0.1 occurred by as much as 95%–180% or 25%–55% when the MOUDI used uncoated ALs or TFs, respectively, as RH was reduced from 50% to 25%. Particle bounce was found to be negligible, and PM0.1 and PM2.5 could be sampled accurately with less than 5% error at the RH of 75%–80% or 65%–80% when uncoated ALs or TFs were used, respectively.
INTRODUCTION
Both ambient ultrafine particles (UFPs) and engineered nanoparticles (NPs) may pose health risks to human beings when they are inhaled or ingested. UFPs and NPs are referred to as PM0.1, or particles with diameter smaller than 0.1 μm, in this study. NPs induce lung injury because of their ability to generate reactive oxygen species. Using the RAW 264.7 phagocytic cell line to compare the cellular effects of ambient UFPs with four other engineered NPs, it was found that ambient UFPs and engineered cationic polystyrene NPs showed clear evidence of cellular toxicity as compared to the other engineered NPs. Ambient UFPs had an additional effect to induce proinflammatory responses (CitationXia et al. 2006). Therefore, it is important to understand the pollutant sources of NPs and adopt proper control measures to avoid human exposure to both UFPs and engineered NPs.
The micro-orifice uniform deposit impactor (MOUDI, MSP Corp., Shoreview, MN, USA) is one of the most commonly used devices for sampling NPs (CitationChow and Watson 2007). The mass concentration of NPs determined by gravimetric analysis and species concentration determined by subsequent chemical analysis help identify the pollutant sources (CitationCass et al. 2000). Uncoated substrates provide minimum interference to chemical analysis of collected samples. However, ambient solid particles may bounce easily when impacting the uncoated substrates or previously deposited particles (Tsai and Cheng 1995). Particles that bounce from the upper impactor stages to the lower stages with smaller cutsizes will lead to overestimation of particle mass concentrations in the lower stages. Applying a sticky substance or low viscosity oil on the substrates was recommended to reduce bounce (CitationGulijk et al. 2003; CitationPak et al., 1992; CitationTurner and Hering 1987), but interference with the chemical analysis of particulate organic carbon could occur. Besides, using a sticky substance would be ineffective in high-temperature sampling (CitationCheng and Yeh 1979). Moreover, evaporation of oil and subsequent adsorption by filters may also occur, which create errors in determining particle mass and chemical species concentrations. Thus, uncoated substrates sometimes are used in field sampling studies, and this could be the reason why the MOUDI oversampled PM0.1 severely as compared to the calculated PM0.1 concentrations from the scaning mobility particle sizer (SMPS) data (CitationKhlystov et al. 2004; CitationShen et al. 2002).
Ambient aerosol particles are known to be hygroscopic due to the presence of inorganic salts and organic acid, leading to water vapor absorption as relative humidity (RH) increases. This suggests that sampling ambient particles at a controlled and high RH condition without using coated impaction substrates is possible since liquid particles are much less bouncy than solid particles. CitationVasiliou et al. (1999) used an RH conditioner made of 16 ceramic tubes and filled with saturated sodium chloride (NaCl) solution to maintain the RH of the aerosol flow entering the uncoated ten-stage MOUDI (MSP Model 11) at about 75% ± 2% during field samplings at Las Vegas with low RHs of about 10%–30%. The ratio of the percent of sulfate mass on the after filter (or PM0.056) to the sum of all stages of the conditioned MOUDI was compared with that of a collocated unconditioned MOUDI. It was found that the bounce of fine ambient particles in the conditioned MOUDI was largely eliminated when the sampled aerosol flow was above ∼70% RH. However, there was a tendency for the sulfate loading at the after filter to increase with decreasing RH due to particle bounce. The average percentage of sulfate mass on MOUDI after filter was 2% and 4%, respectively, for the conditioned and unconditioned MOUDIs implied that the unconditioned MOUDI oversampled PM0.056 by as much as 100%. They concluded that an accurate mass distribution of ambient particles could be obtained by the MOUDI when RHs were kept at above ∼70% but below 80% for fear that flow-induced sizing errors might occur (CitationFang et al. 1991).
CitationStein et al. (1994) used a tandem differential mobility analyzer (TDMA) with an RH conditioner to study the bounce of atmospheric 0.25-μm particles in a one-stage 0.1-μm cutsize impactor in which uncoated aluminum foils (ALs) were used as the impaction substrates. They found that particle bounce increased sharply as RH decreased below 60%–70%. At the RHs of 50%, 40%, 30%, and 20%, about 10%, 20%, 40%, and 70% of incoming 0.25-μm particles bounced. Less than 3% of particles bounced when the RH was kept at 70%–80%, when the surface loading effect on bounce was also found to be negligible. On the other hand, CitationDzubay et al. (1976) studied the bounce of larger particles by using the Anderson 2000 cascade impactor, which had the size cuts of 7.0, 3.3, 2.0, and 1.1 μm. In the study, two of the impactors were compared in parallel on the roof of a 4-m high building near a freeway in downtown Durham, NC, one of which used Dow Corning high-vacuum silicone-grease-coated ALs as impaction substrates while the other used uncoated ALs. The grease-coated impactor was regarded as the reference sampler. They found that the mass median aerodynamic diameter (MMAD) of the uncoated impactors was 2–5 times smaller than the coated impactor due to particle bounce. However, the RH data were not reported and related to the bounce effect on the distortion of MMAD.
The main objective of this article is to study the influence of the RH of the inlet aerosol flow on the UFP concentration collected by the after filter of the MOUDI with different uncoated substrates. In addition, the mass distribution as well as PM2.5 and PM10 fractions were also studied. A humidity control system was operated upstream of two parallel MOUDIs, one of which used silicone-grease-coated ALs (M1, reference MOUDI) and the other used uncoated ALs (M2) or uncoated Teflon filters (TFs, M3) as the impaction substrates. The present experimental setup enabled the study of particle bounce at different conditioned RHs (10%–98%), when conditioned aerosol flow was introduced simultaneously into both MOUDIs to exclude the effect of particle loss in the conditioner on the comparison study since the same inlet was used. The goal of this study is to find out the RH value at which particles do not bounce from uncoated substrates, and measurements of mass distributions and PM0.1, PM2.5, and PM10 concentrations are not influenced by particle bounce.
METHODS
The field comparison tests were conducted 10 m high at the 3rd floor of the Environmental Engineering Building of National Chiao Tung University in Hsinchu, Taiwan. In total, more than forty 24-h samples were taken from January 2009 to March 2010.
A humidity control system consisting of a humidity conditioner (FC 200-780, Perma Pure LLC, Toms River, NJ, USA) with a proportional–integral–differential (PID) controller was used upstream of two collocated MOUDIs as shown in . Fixed RHs of 10%–98% at the inlet aerosol flow with the variation within ±2% RH were set by adjusting the flow rates of the moist and dry sheath air of the humidity conditioner through the automatic PID controller. Normally, the set RH was stabilized within 10 min.
In the MOUDIs, the nozzle plates of 3.2-μm cutsize were replaced with those of 2.5-μm cutsize, and the 56-nm cutsize nozzle plates (stage 10) were removed so that PM0.1 was collected by the after filter; that is, the cutsizes of the MOUDIs in this study were 18, 10, 5.6, 2.5, 1.8, 1.0, 0.56, 0.32, 0.18, and 0.1 μm at sampling flow rate of 30 L/min. Teflon filters (TefloR2PL047, Pall Corp., Washington, NY, USA) were used at the after-filter stage of each tested MOUDI, while three different impaction substrates were used in stages 0–9 of three different MOUDIs, respectively, including grease-coated ALs (M1, reference sampler), uncoated ALs (M2), and uncoated TFs. The impaction plates were rotated against the nozzle plates by 18° for 3 s after every 20-s pause during the operation of all MOUDIs. Conditioned aerosol flow was introduced into M1 and M2 simultaneously, or M1 and M3 simultaneously, and the effect of particle bounce on PM measurement was determined by comparing the PM mass concentration collected at every stage of the MOUDIs as
In our previous study, a MOUDI using coated ALs substrates and the Teflon after filter (similar to the reference sampler M1 in this study) was applied to obtain the chemical mass closure of PM0.1 at different atmospheric environments successfully (CitationChen, Tsai, Chou, et al. 2010; CitationChen, Tsai, Huang, et al. 2010). The PM0.1 concentration measured by the MOUDI was found to agree with that converted from the number concentration of an SMPS (Model 3936, TSI, Inc., Shoreview, MN, USA) by using the effective density obtained by CitationChen, Tsai, Chou, et al. (2010). Both PM2.5 and PM10 concentrations of the grease-coated M1 were also found to be in very good agreement with those of a collocated dichotomous sampler (Model SA-241, Andersen, Inc., GA, USA), with an average relative difference of less than 7.5% (CitationChen, Tsai, Huang, et al. 2010).
Before the particle bounce test, three MOUDIs (M1, M2, and M3), all of which used grease-coated ALs in stages 0–9 and TF in the after filter, were collocated to compare the mass distributions of atmospheric aerosols to ensure no bias between them. In total, five 24-h measurements were conducted. In addition, two MOUDIs (M1 and M2), one (M1) with a humidity conditioner and the other (M2) without a humidity conditioner at its inlet, were collocated in the comparison tests to examine particle loss in the humidity conditioner. Five 24-h measurements were conducted during the cloudy and rainy days of high RH of 80%–95% when particle bounce was not expected to occur (CitationVasiliou et al. 1999). Loss of submicron and ultrafine particles with the diameter smaller than 0.6 μm in the humidity conditioner was examined in more detail by introducing polydisperse NaCl particles into the humidity conditioner. The particles were produced by atomizing an NaCl solution using an atomizer (Model 3076, TSI, Inc., MN, USA), into the humidity conditioner. Subsequently, the loss was determined by comparing the size distributions of NaCl particles at the upstream and downstream of the humidity conditioner as measured by the TSI model 3936 SMPS with a long DMA (TSI model 3081).
In every sampling run, at least two TFs and two uncoated and silicone-grease-coated ALs were used as laboratory and field blanks, respectively, for gravimetric analysis. All filter samples were conditioned at least for 24 h in a temperature and RH controlled room (21.5°C ± 1°C, 40% ± 5% RH) before and after sampling. For coated ALs, 0.3–0.5 mg of silicone grease (KF-96-SP, Topco Technologies Corp., Taiwan) was applied uniformly on the foils (CitationChen, Tsai, Huang, et al. 2010; CitationPak et al. 1992). After coating, the foils were baked in an oven at 65°C for 90 min (CitationMarple et al. 1991). The electrostatic charge of the TFs was eliminated by an ionizing air blower (Model CSD-0911, MEISEI, Japan) before weighing (CitationTsai et al. 2002). A microbalance (Model CP2P-F, Sartorius, Goettingen, Germany) was used to weigh the filters after they were conditioned.
The possibility that the coated silicone grease might evaporate from stages 0–9 during sampling and be subsequently absorbed by the collected PM0.1 particles or the after filter was examined before the sampling campaign. The weight of the coated ALs before and after sampling clean filtered air by a HEPA (high efficiency particulate air) filter for 24 h was compared to check if evaporation occurred from the coated ALs. Results showed that the weight difference was <2 μg for each foil of stages 0–9, indicating evaporation was negligible during sampling. That is, use of silicon grease in stages 0–9 did not interfere with the PM0.1 mass concentrations in this study.
RESULTS AND DISCUSSION
QA/QC Results
The gravimetric analysis of all laboratory and field blanks showed that weight differences between presampling and postsampling were less than 3 μg, which was relatively low compared to the weight of 43 μg for the typical PM0.1 concentration of 1 μg/m3 in urban areas (CitationChen, Tsai, Huang, et al. 2010). The comparison of mass concentrations of ambient particles between three collocated MOUDIs showed very good agreement for all size intervals, especially for the last three stages (PM0.18–0.32, PM0.1–0.18, and PM0.1) as shown in . Four repeat tests, conducted at different days, showed similar results. Particles at the present site were seen to be bimodal including the accumulation and coarse modes, which enabled us to investigate the bounce of UFPs, fine and coarse particles at the same time using different impaction substrates at different RHs.
Particle Loss in the Humidity Conditioner
shows the comparison of mass distributions obtained by the MOUDI with the humidity conditioner installed but not running and the other MOUDI without the conditioner. There was no control of the RH for the MOUDI with the humidity conditioner, and both MOUDIs sampled aerosols at the RH of 80%–95%. The MMADs of the coarse and accumulation modes of the MOUDI with the conditioner were found to be 3.56 and 0.45 μm, respectively, while the corresponding MMADs of the other MOUDI without the conditioner were 3.95 and 0.45 μm, respectively. The same accumulation-mode MMADs and similar mass concentrations in each stage below the 2.5-μm cutsize stage between the two MOUDIs indicated that particles loss in the humidity conditioner was low for fine particles. However, a smaller coarse-mode MMAD (with a difference of ∼10%) and a lower mass concentration in each stage above the 2.5-μm stage of the MOUDI with the conditioner than that without conditioner indicate that a loss of coarse particles occurred in the humidity conditioner. Loss in the conditioner for PM>18 (stage 0), PM10–18 (stage 1), PM5.6–10 (stage 2), and PM2.5–5.6 (stage 3) was 72.5%, 52.3%, 20.9%, and 5.3%, respectively, leading to an underestimation of the corresponding mass concentrations of these large PM fractions. In comparison, PM0.1 concentrations of the two collocated MOUDIs, which were 0.56 and 0.53 μg/m3, respectively, were in very good agreement with a difference of only 5.4%. Four other repeated measurements showed similar results with the average difference of 5.8% ± 2.1% (average ± standard deviation) between the two MOUDIs.
For the humidity conditioner, compares the experimental data of particle loss for particles smaller than 0.6 μm in aerodynamic diameter with the theoretical values obtained from the equation of CitationGormley and Kennedy (1949). In the figure, the aerodynamic diameter of NaCl was converted from the mobility diameter of the SMPS by using an effective density of 1.9 g/cm3 for NaCl particles, which was obtained by comparing the volume concentration determined from the SMPS using the AIM software with the mass concentration from the gravimetric analysis of the collected filter samples of the generated NaCl particles. As can be seen in the figure, the experimental data for particle loss are in very good agreement with the theoretical values with a maximum difference of ∼4%. The experimental particle loss increased from 3.4% to 20.3% as the particle diameter was decreased from 100 to 15 nm. For particles larger than 100 nm, slightly higher experimental losses than the predicted values were observed, because of the fact that the effects of inertial impaction and gravity were not considered in the theory. From the particle loss data and density of NaCl particles, the loss of PM0.1 concentration was calculated to be about only ∼5%. This small loss of PM0.1 is similar to the small difference of 5.4% between the two MOUDIs (one with the conditioner and the other without the conditioner) described previously in . In summary, installing the humidity conditioner at the MOUDI inlet does not lead to the losses of fine and nanosized particles, but the losses for coarse particles are substantial.
FIG. 4 Comparison of particle loss in the humidity conditioner for particles smaller than 600 nm between the experimental data and the predicted values of CitationGormley and Kennedy (1949) equation.
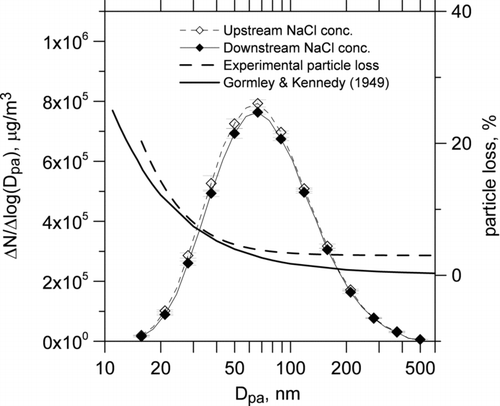
Particle Bounce Effect on Mass Distribution
compares the mass distributions and the MMADs of M1 (coated ALs) with those of M2 (uncoated ALs) or M3 (uncoated TFs) when the inlet aerosols for both collocated MOUDIs were conditioned to 25%, 50%, and 75% RHs. The RHs covered dry, moderate, and wet conditions. Both accumulation- and coarse-mode MMADs of M2 and M3 were found to be in good agreement with those of M1 at 50% and 75% RHs (). However, at RH = 25%, shifting of the mass distribution curve to the smaller diameter occurred, which led to about 9%–12% (M2) or 6%–9% (M3) smaller accumulation- and coarse-mode MMADs, respectively. Even more shifting of the curve toward the smaller size was found at RH = 10% for M2 when the reduction of accumulation- and coarse-mode MMADs was 21% and 15%, respectively.
FIG. 5 Comparison of particle mass distributions and the accumulation and coarse MMADs of M2 or M3 with those of M1 at the conditioned RH of 25%, 50%, and 75%.
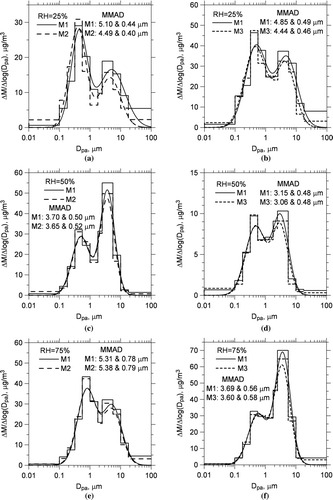
It was found that M1 sampled much more PM2.5–10 mass than M2 and M3 at both RHs of 25% and 50%. M2 and M3 undersampled PM2.5–10 by 22.5% and 14.2%, respectively, at the RH of 25%, while undersampling at 50% RH was less (11.0% and 10.2% respectively) for M2 and M3. That is, more particles bounced from uncoated ALs than from uncoated TFs at RHs <50%. Besides, M2 and M3 were also found to undersample particles at upper 0th, 1st, and 2nd stages even at an RH as high as 75% (), with 30.5%, 7.0%, and 8.4% underestimation for M2 and 26.5%, 6.4%, and 8.4% underestimation for M3, respectively. Particle bounce was found to be reduced further at RHs of 80%–98%, and undersampling was reduced to less than 3% for both M2 and M3. The observation that bounce occurred for particles larger than 2.5 μm was in agreement with that observed by CitationDzubay et al. (1976). The bouncing particles were lost on the inner wall of the MOUDI rather than bounced to the lower stages, as it was evident that the sum of PM mass at lower 4–9 stages did not increase in M2 and M3. This speculation was also observed by CitationCheng and Yeh (1979) who found that wall loss of 7, 5, 3, and 1.8-μm particles was about 58%, 54%, 40%, and 20%, respectively, in a ungreased Sierra radial slit jet cascade impactor (Model 216, Sierra Instruments, Inc., CA, USA).
Ratios of M2 and M3 PM concentrations to those of M1 at different RHs are compared in to examine the effects of RHs and different substrates on particle bounce. The linear regressions of PM0.1 and PM10 ratios are also shown in the figure. Note that the regression of PM10 ratios was obtained from pooling both M2 and M3 data since they showed a similar trend. From the figure, it is observed that the ratios of M2 and M3 to M1 are close to 1 for all PMs at high RHs of 75%–98%. Surprisingly, the ratios of M2 and M3 to M1 for PM2.5 were both found to be near 1 even when RH was decreased from 75% to 10% or 20%, respectively. However, the PM10 ratios were decreased to ∼0.75 and ∼0.8 for M2 and M3, respectively, at these low RHs. This was due to the loss of coarse particles (PM2.5–10) at the low RHs as discussed previously. Although M2 and M3 sampled PM2.5 concentrations close to those of M1 even at low RH of 10% or 20%, their mass distributions and accumulation MMADs deviated severely from those of M1, which can be seen in and , respectively. This is because fine particles bounced to the lower stages rather than being lost in the inner wall, as was the case for coarse particles.
Particle Bounce Effect on PM0.1 Concentration
The average PM0.1 determined by M1 for all forty samples was 0.8 ± 0.3 μg/m3 (average ± standard deviation), which was comparable with those at the urban areas in Los Angeles, CA (CitationCass et al. 2000). As was mentioned previously, the present M1 determined a comparable PM0.1 concentration to that converted from the SMPS data. The PM0.1 of M1 was therefore used as a reference for quantifying the influence of particle bounce on PM0.1 of M2 and M3.
It was found that both M2 and M3 collected PM0.1 concentrations very close to those of M1 at the RH of 75% (). However, bouncing particles from stages 8 and 9 to the after filter (PM0.1) led to oversampling of PM0.1 by more than 30% at RHs of 25% and 50% (). Oversampling of PM0.1 by 55% at 25% RH in M3 was very close to that found in CitationVasiliou et al. (1999). Particles could also bounce from 4 to 6 stages and be collected at lower stages (stages 7–9 and the after filter) at the RH of 25% for M2 (). Less severe bounce in M3 (TFs) than that in M2 (ALs) at the RH of 25% or 50% was found because of the softer filter surface and the additional filtration mechanism of M3.
shows bounce starts to occur at RHs below 75% and 65% in M2 and M3, respectively, which was also found by CitationStein et al. (1994) and CitationVasiliou et al. (1999). Both M2 and M3 sampled PM0.1 concentrations close to those of M1 at RHs >∼75 and >∼65%, respectively, with a difference of less than 5%. However, PM0.1 was oversampled by M2 by as much as 95% and 180% at the RH of 50% and 25%, respectively, while oversampling by M3 was less, which was 25% and 55%, respectively. On the basis of the present results, it can be concluded that particle bounce is negligible at the RH of >65% or >75% when uncoated TFs (M3) or uncoated ALs (M2) are used as the impaction substrates of the MOUDI.
In order to know the bounce effect on the distortion of the mass distribution, the ratio of the accumulation-mode MMADs of M2 and M3 to those of M1 at the RH from 10% to 98% was compared and shown in . As can be seen, the MMADs of both M2 and M3 are in good agreement with those of M1 at RH >∼40% with a difference of less than ±5%. However, the difference increased with decreasing RH, and the ratio was decreased to ∼0.75 for M2 at the RH of 10% and to ∼0.9 for M3 at the RH of 20%. That is, accumulation-mode particles can be collected accurately by the MOUDI using uncoated substrates as RH >40%.
CONCLUSION
The effects of RH of the incoming aerosols and different substrates on particle bounce in the MOUDI were examined in this study. The tested RH ranged from 10% to 98%, which was achieved by a humidity conditioner, and the tested substrates were silicone-grease-coated ALs (M1), uncoated ALs (M2), and uncoated TFs (M3). Before the bounce test, particle loss in the conditioner was examined first, and results showed that the conditioner did not lead to losses of fine and nanosized particles, but losses for coarse particles were severe.
Because of particle bounce, M2 oversampled PM0.1 by as much as 95% and 180% at the RH of 50% and 25%, respectively, while PM0.1 oversampling by M3 was 25% and 55%, respectively, at these two RHs. Less severe bounce in M3 (TFs) than in M2 (ALs) at the RH of 25% and 50% was caused possibly by the more rigid surface of ALs than TFs. It was found that the accumulation-mode particles could be collected accurately by the MOUDI using uncoated substrates when RH > 40%. Both M2 and M3 sampled PM2.5 comparably to M1 even when RH was decreased from 75% to 10% or 20%, respectively, because bouncing fine particles were collected by the lower stages of the MOUDI. In comparison, larger bouncing particles of PM>18 (stage 0), PM10–18 (stage 1), PM5.6–10 (stage 2), and PM2.5–5.6 (stage 3) were lost on the inner wall of M2 and M3 rather than bounced to the lower stages. On the basis of the present experimental results and the previous finding that flow-induced sizing errors occurred at RH > 80% (CitationFang et al. 1991), it is concluded that PM0.1 and PM2.5 can be obtained accurately with less than 5% error at RHs of 65%–80% and 75%–80%, respectively, when uncoated TFs and uncoated ALs are used in MOUDIs.
Acknowledgments
The financial support of the Taiwan EPA (EPA-97-U1U1-02-106 and EPA-98-U1U1-02-103) and Taiwan National Science Council (NSC 98-2221-E-009-020-MY3) are gratefully acknowledged.
REFERENCES
- Cass , G. R. , Hughes , L. A. , Bhave , P. , Kleeman , M. J. , Allen , J. O. and Salmon , L. G. 2000 . The Chemical Composition of Atmospheric Ultrafine Particles . Philos. Trans. R. Soc. London, Ser. A. , 358 : 2581 – 2592 .
- Chen , S. C. , Tsai , C. J. , Chou , C. K. , Roam , G. D. , Cheng , S. S. and Wang , Y. N. 2010 . Ultrafine Particles at Three Different Sampling Locations in Taiwan . Atmos. Environ. , 44 : 533 – 540 .
- Chen , S. C. , Tsai , C. J. , Huang , C. Y. , Chen , H. D. , Chen , S. J. , Lin , C. C. , Chou Charles , C.-K. , Lung , S. C. , Roam , G. D. , Wu , W. Y. , Smolik , J. and Dzumbova , L. 2010 . Chemical Mass Closure and Chemical Characteristics of Ambient PM0.1, PM2.5 and PM10 in a Highway Tunnel and at a Roadside . Aerosol Sci. Technol. , 44 : 713 – 723 .
- Cheng , Y. S. and Yeh , H. C. 1979 . Particle Bounce in Cascade Impactors . Environ. Sci. Technol. , 13 : 1392 – 1396 .
- Chow , J. C. and Watson , J. G. 2007 . Survey of Measurement and Composition of Ultrafine Particles . Aerosol Air Qual. Res. , 7 : 121 – 173 .
- Dzubay , T. G. , Hines , L. E. and Stevens , R. K. 1976 . Particle Bounce Errors in Cascade Impactors . Atmos. Environ. , 10 : 229 – 234 .
- Fang , C. P. , McMurry , P. H. , Marple , V. A. and Rubow , K. L. 1991 . Effect of Flow-Induced Relative-Humidity Changes on Size Cuts for Sulfuric-Acid Droplets in the Microorifice Uniform Deposit Impactor (MOUDI) . Aerosol Sci. Technol. , 14 : 266 – 277 .
- Gormley , P. G. and Kennedy , M. 1949 . Diffusion from a Stream Flowing through a Cylindrical Tube . Proc. Royal Irish Acad. , 52 ( A ) : 163 – 169 .
- Gulijk , C. V. , Marijnissen , J. C. M. , Makkee , M. and Moulijn , J. A. 2003 . Technical Note Oil-Soaked Sintered Impactors for the ELPI in Diesel Particulate Measurements . J. Aerosol Sci. , 34 : 635 – 640 .
- Khlystov , A. , Stanier , C. and Pandis , S. N. 2004 . An Algorithm for Combining Electrical Mobility and Aerodynamic Size Ddistributions Data When Measuring Ambient Aerosol . Aerosol Sci. Technol. , 38 ( S1 ) : 229 – 238 .
- Marple , V. A. , Robow , K. L. and Behm , S. M. 1991 . A Microorifice Uniform Deposit Impactor (MOUDI): Description, Calibration, and Use . Aerosol Sci. Technol. , 14 : 434 – 446 .
- McFarland , A. R. , Ortiz , C. A. and Bertch , R. W. Jr. 1984 . A 10 mm Cutpoint Size Selective Inlet for Hi-Vol Samplers . J. Air Pollut. Control Assoc. , 34 : 544 – 547 .
- Pak , S. S. , Liu , B. Y. H. and Rubow , K. L. 1992 . Effect of Coating Thickness on Particle Bounce in Inertial Impactors . Aerosol Sci. Technol. , 16 : 141 – 150 .
- Peters , A. , Wichmann , H. E. , Tuch , T. , Heinrich , J. and Heyder , J. 1997 . Respiratory Effects Are Associated with the Number of Ultrafine Particles . Am. J. Respir. Crit. Care Med. , 155 : 1376 – 1383 .
- Shen , S. , Jaques , P. A. , Zhu , Y. , Geller , M. D. and Sioutas , C. 2002 . Evaluation of the SMPS-APS System as a Continuous Monitor for Measuring PM2.5, PM10 and Coarse (PM2.5–10) Concentration . Atmos. Environ. , 36 : 3939 – 3950 .
- Stein , S. W. , Turpin , B. J. , Cai , X. P. , Huang , C. P. F. and McMurry , P. H. 1994 . Measurements of Relative Humidity-Dependent Bounce and Density for Atmospheric Particles Using the DMA-Impactor Technique . Atmos. Environ. , 28 : 1739 – 1746 .
- Tsai , C. J. , Chang , C. T. , Shih , B. H. , Aggarwal , S. G. , Li , S. N. , Chein , H. M. and Shih , T. S. 2002 . The Effect of Environmental Conditions and Electrical Charge on the Weighing Accuracy of Different Filter Materials . Sci. Total Environ. , 293 : 201 – 206 .
- Tsai , C. J. and Cheng , Y. H. 1995 . Solid Particle Collection Characteristics on Impaction Surfaces of Different Designs . Aerosol Sci. Technol. , 23 : 96 – 106 .
- Turner , J. R. and Hering , S. V. 1987 . Greased and Oiled Substrates as Bounce-Free Impaction Surfaces . J. Aerosol Sci. , 18 : 215 – 224 .
- Vasiliou , J. G. , Sorensen , D. and McMurry , P. H. 1999 . Sampling at Controlled Relative Humidity with a Cascade Impactor . Atmos. Environ. , 33 : 1049 – 1056 .
- Whitby , E. and Whitby , N. 1989 . DISFIT software , Minneapolis, MN : TSI Inc. .
- Xia , T. , Kovochich , M. , Brant , J. , Hotze , M. , Sempf , J. , Oberley , T. , Sioutas , C. , Yeh , J. I. , Wiesner , M. R. and Nel , A. E. 2006 . Comparison of the Abilities of Ambient and Manufactured Nanoparticles to Induce Cellular Toxicity According to an Oxidative Stress Paradigm . Nano Lett. , 6 : 1794 – 1807 .