Abstract
The nano aerosol mass spectrometer (NAMS) was deployed at a coastal site in Lewes, Delaware, to measure the composition of 21 nm mass normalized (18 nm mobility) diameter nanoparticles during new particle formation (NPF) events. NAMS provides a quantitative measure of the atomic composition of individual nanoparticles. NAMS analysis of ambient particles showed only a small change in particle composition during NPF events in Lewes compared with off-event (before and/or after the event). The N mole fraction increased 15% on-event, whereas the C mole fraction decreased 25%, suggesting an enhanced inorganic component to the aerosol during NPF. The measured changes in atomic composition constrain the possible changes in molecular composition. To explore these constraints, an apportionment algorithm was applied to the atomic composition data. This algorithm partitions the atomic composition into sulfate, nitrate, and ammonium on the basis of the atomic abundance of S, N, and O and into organic matter on the basis of C and residual O after removing contributions to inorganic species. Particles were fully neutralized both on- and off-event. The nitrate to sulfate ratio during NPF ranged from 0.7 to 1.4, suggesting that ammonium nitrate is important to particle growth in this environment. Nonetheless, nanoparticles had a significant organic fraction, and upper limits for cationic amine content were determined. The relatively small changes in total particle composition on-event versus off-event suggest that observed changes in particle hygroscopicity and volatility during NPF at other locations may be linked to subtle changes in particle composition or to shifts in the character of the organic content.
1 INTRODUCTION
Significant uncertainty exists in modeling and predicting aerosol effects on climate (CitationSolomon et al. 2007). This uncertainty arises in part due to difficulty in predicting ambient cloud condensation nuclei (CCN) levels under varying conditions (CitationSpracklen et al. 2008; CitationKuang et al. 2010). Although recent models have suggested that new particle formation (NPF) can contribute up to 45% of global CCN levels (CitationMerikanto et al. 2009), there exists considerable uncertainty in our understanding of the mechanisms of ambient particle formation and growth. Development of improved climate models requires a better understanding of these mechanisms, which in turn necessitates measurements of the chemical composition of particles <30 nm diameter.
Few online chemical composition measurements have been performed on ambient nanoparticles (CitationBzdek and Johnston 2010). The thermal desorption chemical ionization mass spectrometer (TDCIMS) has been used to determine the molecular composition of particles down to 6 nm diameter (CitationVoisin et al. 2003; CitationSmith et al. 2004; CitationHeld et al. 2009). The TDCIMS has been previously deployed to study particle composition during NPF in Atlanta, Georgia; Tecamac, Mexico; and Hyytiälä, Finland (CitationSmith et al. 2005, Citation2008, Citation2010). In Atlanta, ammonium sulfate was found to account for virtually all of the sampled nanoparticle mass (CitationSmith et al. 2005); however, in Tecamac and Hyytiälä, the predominant molecular species found in the newly nucleated aerosol were sulfate, nitrate, organic amines, and organic acids (CitationSmith et al. 2008, Citation2010). The presence of these organic species has led to the hypothesis that organic aminium salts may drive nanoparticle growth during NPF (CitationSmith et al. 2010).
The nano aerosol mass spectrometer (NAMS) is a complementary technique to the TDCIMS, as it performs quantitative atomic analysis of single particles down to 7 nm diameter (CitationWang et al. 2006; CitationWang and Johnston 2006). NAMS has been previously used to characterize ambient nanoparticles in an urban environment (CitationZordan et al. 2008). In that study, particles emitted from motor vehicles were distinguished from photochemically generated particles on the basis of atomic composition, and the molecular compositions of both types of particles were inferred on the basis of knowledge of molecular species commonly present in ultrafine aerosol. In the present study, NAMS was deployed at a field site in Lewes, Delaware, in autumn 2007 to characterize the composition of ambient nanoparticles during NPF. Changes in atomic composition that occur during NPF are described and the constraints they impose on the molecular species contributing to particle growth in this environment are discussed with the aid of an apportionment algorithm.
2 EXPERIMENTAL SECTION
The field campaign was conducted at the Hugh R. Sharp Campus of the University of Delaware in Lewes, Delaware, USA (38°47′02″;N, 75°09′39″;W) from 15 October 2007 to 12 November 2007. The campus is located on the Delaware Bay, which is at the outlet of the Delaware River to the Atlantic Ocean. The field site is situated about 800 m south of the Delaware Bay, 3 km west of the Atlantic Ocean, and is adjacent (<50 m) to a large salt marsh on the west. This site is the location of a previous campaign to study gas-phase sulfur (S) emissions and to perform a sulfur dioxide intercomparison experiment (CitationLuther and Stecher 1997; CitationStecher et al. 1997). The site is occasionally impacted by sulfur dioxide emissions from a coal-fired power plant 23 km to the south-southwest.
Ambient particle composition measurements were accomplished using NAMS, which gives a quantitative measure of the atomic composition of individual size-selected nanoparticles in the 7–30 nm size range (CitationWang et al. 2006; CitationWang and Johnston 2006). Particles were sampled through an inlet approximately 6 m above the ground. Inside NAMS, particles flow through a series of aerodynamic and electrodynamic focusing elements and then are size-selectively captured in an ion trap. Particles are irradiated with a high-energy pulsed laser beam to reach the so-called “complete ionization limit,” where a plasma is formed. Each particle is completely disintegrated into positively charged atomic ions that are mass analyzed by time-of-flight. The relative intensities of these ions give the atomic composition. For standard compounds, the atomic mole ratios obtained by NAMS are typically within 10% of their expected values (CitationZordan et al. 2010). The sampled particle size range is selected by the frequency applied to the ring electrode of the ion trap. For this study, NAMS sampled 21 nm mass normalized diameter particles, which (assuming a spherical particle and 1.7 g/mL density) corresponds to a mobility diameter of about 18 nm. For singly charged spherical particles, the mass normalized diameter equals the mobility diameter multiplied by the cube root of the density (CitationWang and Johnston 2006).
In addition to particle composition measurements, particle size distributions were monitored throughout the campaign by a scanning mobility particle sizer (SMPS; electrostatic classifier model 3080, condensation particle counter model 3025a, TSI, Inc., St. Paul, MN). A unipolar, rather than a bipolar, charger was used to charge the sampled particles entering the SMPS in order to increase the number of charged particles for NAMS analysis. (The experimental setup for this study required particles entering the SMPS and NAMS to be charged by the same device.) An empirical correction to the obtained particle size distribution was applied in order to account for the difference in charging efficiency between the chargers. Because of the possibility of particles >20 nm mobility diameter taking on multiple charges when using a unipolar charger (CitationMcMurry et al. 2009), growth rate estimates are only given to one significant figure. Note that the use of the unipolar charger has little effect on mass spectrometric measurements, as the size of particle analyzed is determined by a mass-to-charge (m/z) ratio. A doubly charged particle could be analyzed at the same m/z as a singly charged one; however, since mass is proportional to the cube of diameter, this doubly charged particle would have a mass normalized diameter only a few nm larger than the desired singly charged particle. Additionally, since analysis is performed on a particle-by-particle basis, rather than on a mass basis, the contribution of these particles to average particle composition is relatively small.
Particle molecular composition is inferred from atomic composition. The goal is to put the atomic composition into molecular context based on the major constituents that are typically found in ambient nanoparticles: sulfate, nitrate, ammonium, and carbonaceous matter. Accordingly, our molecular apportionment algorithm partitions the atomic composition into the three inorganic species based on atomic abundances of S, nitrogen (N), and oxygen (O) and into organic matter based on carbon (C) and residual O after removing contributions to inorganic species. No attempt is made to apportion the atomic composition into other inorganic species (e.g., metal oxides) because no other inorganic ions are consistently detected in Lewes. The one exception is silicon dioxide, which is apportioned owing to the occasional detection of a small silicon (Si) atomic composition in Lewes. Si is much more prevalent in urban nanoparticles (CitationZordan et al. 2008).
The apportionment algorithm used in this work is summarized in . First, S and Si are assumed to be in oxidized forms (sulfate, silicon dioxide). N is assumed to be in cationic form (the algorithm assumes ammonium) until all sulfate is neutralized. Thereafter, any remaining N is assumed to be ammonium nitrate. Finally, C and any residual O are assigned to carbonaceous matter. The apportionment scheme does not assume any oxidation state for the C in the particle. However, the O/C ratio for carbonaceous matter in a particle will permit determination of the average carbon oxidation state in the particle.
TABLE 1 Molecular apportionment of the atomic composition measured by NAMS
Organic compounds containing heteroatoms, for example, organosulfates and organonitrates, are not considered in the apportionment scheme. The “inorganic” portion of these species would be apportioned to sulfate or nitrate with the organic component being apportioned to carbonaceous matter. In terms of cationic N species, both cationic ammonium and aminium species would be partitioned to ammonium by the apportionment scheme. Whereas amine N would correctly be apportioned to the cation, the organic portion would be apportioned to carbonaceous matter.
Particle mass spectra consisting of ≥90% atomic (molar) area of a single non-C atom are deemed to represent unrealistic (or at least un-interpretable) particle compositions and are not subjected to molecular apportionment. These particles typically represent less than 0.1% of total particles in a dataset. Particles consisting of ≥90% atomic area of O are tabulated separately as particulate water. For some particles that are subjected to molecular apportionment, there is not enough O to assign all of the S, Si, and N to the corresponding molecular species. In these cases, the residual N, Si, and S signals are tabulated as “unapportioned” atomic composition. Typically, unapportioned matter represents less than about 5% of the atomic content of an ambient particle dataset. Unapportioned matter and removed particles arise primarily from pulse-to-pulse variations of the laser ablation process, which cause some particle mass spectra to deviate substantially from the true chemical composition (CitationZordan et al. 2010).
3 RESULTS AND DISCUSSION
NPF events were frequently observed at the field site. presents the SMPS-measured particle size distribution as a function of time for a 5-day period (4–9 November 2007) during which NPF was observed every day. The SMPS measurements were made on 23 days during the entire study period (15 October–12 November), and NPF events were observed on 13 of those days. Although detailed meteorological data from the field site is unavailable, some information was gained through HYSPLIT back trajectories and from meteorological data at a National Oceanic and Atmospheric Administration (NOAA) station approximately 3 km to the east of the site. Particle event days were usually sunny with no precipitation, whereas nonevent days were cloudy with occasional precipitation. These observations suggest NPF was photochemically driven. Note that 6 November had significant cloudiness and some precipitation during parts of the day, which may have truncated the event. The air masses carrying particle events examined by NAMS were tracked using HYSPLIT (CitationDraxler and Rolph 2011). These air masses were of continental origin from regions north and west of the site, having passed over the Washington, DC; Baltimore, Maryland; and/or Philadelphia, Pennsylvania, metropolitan areas. It is unlikely that emissions from the coal-fired power plant located 23 km to the south-southwest influenced these events, as air masses carrying the events uniformly arrived from the north and west of the site, and local wind directions measured 3 km from the site also were from the north and west. Wind speeds during these events ranged from 2–8 km/h. As illustrated in , NPF typically occurred in the late morning (9:00 a.m.–12:00 p.m. local time) with the abrupt appearance of particles <10 nm mobility diameter followed by growth to a final mobility diameter ∼40 nm. Growth rates from 10 to 25 nm mobility diameter were estimated by plotting the most intense (mode) particle diameter against local time. These data were fit linearly, and the growth rate for the event was estimated by solving the first derivative of the fit. NAMS was operational on 7 of the 13 event days observed by SMPS. The results we present herein focus on those specific days (20, 21, 28 October and 4, 5, 8, 11 November). Growth rates (from 10 to 25 nm mobility diameter) for these days ranged from 2 to 6 nm/h (median: 3 nm/h) and are provided in .
TABLE 2 Particle growth rates from 10 to 25 nm mobility diameter during the analyzed NPF events
FIG. 1 SMPS-measured contour plot of the particle size distribution for 4–9 November 2007. The dotted lines indicate the range of mobility diameters for particles analyzed by NAMS.
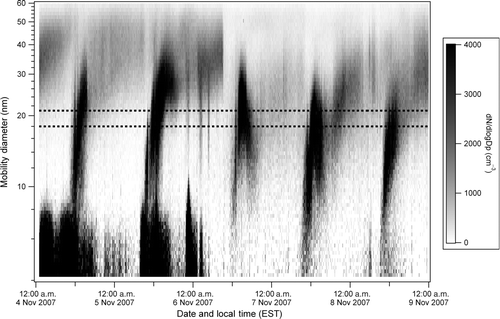
The growth rates observed during this study are similar to those observed during many other studies of NPF (CitationKulmala et al. 2004). Notably, our range of growth rates (2–6 nm/h) is within the range typically observed in Hyytiälä, Finland, a remote boreal environment that has been extensively studied (CitationDal Maso et al. 2002, Citation2005). However, the growth rates are much lower than those reported at a coastal site in Mace Head, Ireland (15–180 nm/h; CitationDal Maso et al. 2002; CitationO’Dowd et al. 2007), and an urban site in Tecamac, Mexico (15–40 nm/h; CitationIida et al. 2008). One potential explanation for the difference in growth rates is that different mechanisms are involved in NPF in these environments; however, this hypothesis would require much further exploration.
provides an average mass spectrum of particles analyzed by NAMS during a particle event (11:00 a.m.–3:30 p.m. local time) on 8 November, which is representative of the particle composition during NPF in Lewes. As discussed previously, the high irradiance of the laser pulse forms a plasma that disintegrates the particle into multiply charged atomic ions. The relative signal intensities of these atomic ions give a quantitative measure of the particle atomic composition. The “limited” mass resolving power of NAMS results in some complications in interpretation of the mass spectrum, as isobaric ion signals are present at 4 m/z (C+3 and O+4) and 8 m/z (O+2 and S+4). We have developed a method to deconvolute these isobaric ion signals (CitationZordan et al. 2010). also provides a pie chart that gives the relative atomic mole fractions of all particles during this event.
FIG. 2 Average mass spectrum of particles analyzed by NAMS during the NPF event on 8 November 2007 (11:00 a.m.–3:30 p.m. local time). The pie chart gives the atomic mole fractions during this period.
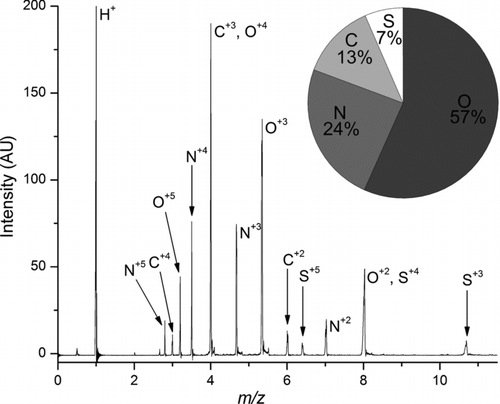
NAMS analysis of event days showed only small changes in average particle composition on-event compared to off-event (i.e., particle composition during the same day but before and/or after the event). illustrates how the atomic mole fractions of different elements increased or decreased in intensity during NPF events compared to off-event. All studied events are included in this figure, with the exception of 4 November, as no particles were analyzed off-event on this day. Median values are given by the bar, whereas the range over all studied days is given by the whiskers. We relate these changes by the percent change in the atomic mole fraction on-event versus off-event. The most obvious compositional changes are a decrease in the C mole fraction and an increase in the N mole fraction. The median decrease in the C mole fraction during events was ∼25%, whereas the N mole fraction increased by ∼16%. These changes are of a magnitude greater than the uncertainty associated with atomic composition analysis (indicated by the dotted lines in ), as NAMS typically measures atomic mole ratios to within ±10%, which corresponds to an uncertainty of ∼7% for individual atomic mole fractions (CitationZordan et al. 2010). Changes to O and S mole fractions were much smaller, and the changes may be within the uncertainty assigned to these values, with a median increase of 3% for O and 8% for S during NPF. Note that the S mole fraction in particles was always very low (≤7% of the total atomic composition, see ). As a result, small changes in the measured atomic S mole fraction in the particles on-event relative to off-event (i.e., from 7% to 5% of the particle) sometimes translated to large percent changes in the S mole fraction. This was not a complicating factor for the other elements, as their mole fractions were always much larger. The increase in N mole fraction coupled with the decrease in C mole fraction suggests an enhanced inorganic component to the particles during NPF. provides particle composition on- and off-event for all studied events. A 10% uncertainty is assigned to the molar ratios (CitationZordan et al. 2010).
TABLE 2 Average on- and off-event atomic mole fractions on NPF event days. Molar N/S, NO3 −/SO4 −2, C/Ncation, and O/C ratios indicate changes in average particle composition during events. The final column provides the number of particles analyzed in each data set. Median values exclude 4 November, where particle composition was not measured off-event a
FIG. 3 (a) Comparison of the percent change in atomic mole fraction on- versus off-event. (b) Comparison of the change in the molar ratios N/S, NO3 −/SO4 −2, C/Ncation, and O/C ratios on- versus off-event. The dotted line separates atomic ratios determined directly by NAMS (to the left) from molecular ratios inferred from the apportionment scheme (to the right). For both (a) and (b), median values for event days analyzed by NAMS are given by the bar. Whiskers indicate the range of values for individual days. The event on 4 November is excluded from these plots, as particle composition was not measured off-event on this day. In (a), the dotted lines represent the uncertainty in atomic mole fraction measured by NAMS.
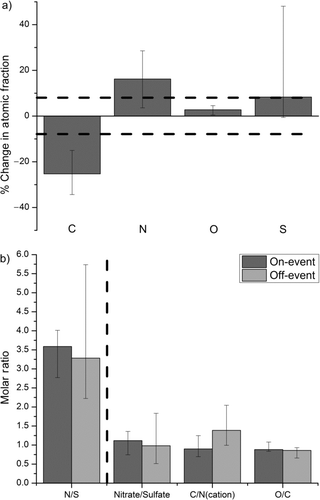
Comparison of changes in specific atomic ratios can provide some insight into changes in particle composition during NPF. For instance, the ratio of the number of moles of N to the number of moles of S (N/S) permits one to infer particle acidity, as two moles of N (as ammonium) would be required to fully neutralize one mole of S (as sulfate). Therefore, a molar N/S ratio <2 indicates that not all sulfate is neutralized (acidic aerosol), whereas a molar N/S ratio ≥2 indicates that sufficient N exists to neutralize all S. presents the median N/S ratio on-event and off-event (left of the dotted line). The event on 4 November is excluded because particle composition was not measured off-event. Except for one day (5 November), N/S always increased during the event relative to off-event, implicating N-containing species as playing an important role in the event. Additionally, these results indicate that the aerosol (both on- and off-event) is fully neutralized according to the apportionment mechanism (), as N/S is always much greater than two (2 NH4 +:1 SO4 −2). gives the molar N/S ratio for each event.
We also applied a molecular apportionment scheme () to the atomic compositional data in order to infer changes in nanoparticle molecular composition. We calculate molar NO3 −/SO4 −2, C/Ncation, and O/C ratios. NO3 −/SO4 −2 is moles of nitrate divided by moles of sulfate obtained from apportionment, C/Ncation is the moles of C (not apportioned to any molecular species in our algorithm) divided by the moles of N apportioned to ammonium, and O/C is the O to C atomic ratio for carbonaceous matter in the particle.
In our apportionment scheme, N is apportioned to ammonium or both ammonium and ammonium nitrate depending on the amount of S (assumed to be entirely sulfate) in the particle. Once all sulfate is neutralized (2 NH4 +:1 SO4 −2), ammonium and nitrate are apportioned in a 1:1 molar ratio (1 NH4 +:1 NO3 −). In this apportionment, Ncation represents a lower limit to the molar cationic N content of the particle. This value would increase if N served as cation to another acid, such as an organic acid, rather than as nitrate. If we assume that all C exists as part of N-containing species (as opposed to organic acids or oxidized C species), the C/Ncation ratio provides an upper limit for amine content in the particle. If organic acids are present, C/Ncation would decrease; if some N were incorrectly apportioned to nitrate, Ncation would increase, resulting in a decrease in C/Ncation.
summarizes NO3 −/SO4 −2, C/Ncation, and O/C (right of the dotted line) on- and off-event for six events analyzed by NAMS. gives these values for each event. Not surprisingly, NO3 −/SO4 −2 and N/S track each other. NO3 −/SO4 −2 always increased during NPF, except for the event on 5 November. NO3 −/SO4 −2 ratios indicate that both species are significant and sometimes equally important components of the aerosol on a molar basis, as NO3 −/SO4 −2 values ranged from 0.7 to 1.4 during NPF. This range of values is in qualitative agreement with TDCIMS measurements of nitrate and sulfate ion signal intensities during NPF in Tecamac, Mexico, and Hyytiälä, Finland (CitationSmith et al. 2008, Citation2010). In Lewes, C/Ncation always decreased relative to off-event composition during NPF. The increases in N/S and NO3 −/SO4 −2, coupled with the observed decrease in C/Ncation, suggest an increase of inorganic components during NPF in Lewes. In particular, these results implicate ammonium nitrate as playing an important role in the events, as the N content increases during NPF while C content decreases. To our knowledge, ammonium nitrate has not previously been discussed as a key species in particle growth for this size range.
While changes in N/S, NO3 −/SO4 −2, and C/Ncation suggest an important role of ammonium nitrate during NPF in Lewes, we cannot exclude the possibility that amines are also important components of these particles. This is evidenced by a substantial C mole fraction (). For example, the median value of C/Ncation is 0.90 during NPF events. If we assume that monomethylamine (CH3NH2; C/N = 1:1) is the only amine present, then up to 90% of the Ncation could be composed of monomethylammonium. If we assume that trimethylamine [(CH3)3N; C/N = 3:1] is the only amine present in the particle, then up to 30% of the Ncation could consist of trimethylammonium. We emphasize that these values are only upper limits for amine content. Actual amine content could be much lower but would require quantitative molecular composition measurements.
It is noteworthy that the O/C ratio only slightly increased during NPF and that it was always very high (>0.8) during NPF, which indicates a significant component of highly oxidized organics in these particles. The exact character of organic species in these nanoparticles is uncertain, as NAMS only gives the atomic composition. It is possible, for example, that much of the carbonaceous species contributing to the higher C/Ncation ratio off-event is the result of organics partitioning to the particle phase, whereas during particle events the overall character of the organic material shifts to amines and/or organic acids.
The NAMS data provide important insight into nanoparticle composition during NPF. The atomic compositions on- versus off-event are quite similar, suggesting that decreases observed in ultrafine particle volatility and hygroscopicity during NPF in other locations (CitationEhn et al. 2007; CitationRiipinen et al. 2009; CitationAsmi et al. 2010; CitationDusek et al. 2010; CitationSmith et al. 2010) are either linked to subtle changes in particle composition (e.g., a thin coating on the particle surface) or to shifts in the character of the organic content (e.g., an enhanced amine/organic acid component). In this regard, TDCIMS measurements in Tecamac and Hyytiälä show that the relative ion signals of individual molecular species can change by a factor of two or more during NPF (CitationSmith et al. 2008, Citation2010). We note that the NAMS spectra did not indicate the presence of any higher atomic weight species such as sodium, chloride, and iodine that might influence the physical properties of the particles. However, the sensitivity of our instrument to these elements has not been fully explored. The observations presented here are for several event days over the period of a month, whereas most discussions of NPF in the literature tend to focus on only one day in specific. The consistency in observations from one event to the next suggests that these results are representative of Lewes, Delaware, in autumn.
In conclusion, measured changes in atomic composition during NPF constrain the possible changes in molecular composition. Future work is needed to assess more quantitatively the specific changes in molecular composition during NPF and, in particular, to explore the role of N (ammonium, aminium, and/or nitrate) in particle growth in this environment.
Acknowledgments
The authors acknowledge Katherine M. Mullaugh for assistance during the field campaign, Elizabeth Frey of the Delaware Department of Natural Resources and Environmental Control (DNREC), and the NOAA Air Resources Laboratory (ARL) for the provision of the HYSPLIT transport and dispersion model and READY website (http://www.arl.noaa.gov/ready.php) used in this publication. Bryan R. Bzdek acknowledges a graduate fellowship from the University of Delaware Center for Critical Zone Research. This research was supported by NSF grant no. CHE-0808972.
REFERENCES
- Asmi , E. , Frey , A. , Virkkula , A. , Ehn , M. , Manninen , H. E. , Timonen , H. , Tolonen-Kivimaki , O. , Aurela , M. , Hillamo , R. and Kulmala , M. 2010 . Hygroscopicity and Chemical Composition of Antarctic Sub-Micrometre Aerosol Particles and Observations of New Particle Formation . Atmos. Chem. Phys. , 10 : 4253 – 4271 .
- Bzdek , B. R. and Johnston , M. V. 2010 . New Particle Formation and Growth in the Troposphere . Anal. Chem. , 82 : 7871 – 7878 .
- Dal Maso , M. , Kulmala , M. , Lehtinen , K. E. J. , Makela , J. M. , Aalto , P. and O’Dowd , C. D. 2002 . Condensation and Coagulation Sinks and Formation of Nucleation Mode Particles in Coastal and Boreal Forest Boundary Layers . J. Geophys. Res.—Atmos. , 107 : 8097 DOI: 8010.1029/2001jd001053
- Dal Maso , M. , Kulmala , M. , Riipinen , I. , Wagner , R. , Hussein , T. , Aalto , P. P. and Lehtinen , K. E. J. 2005 . Formation and Growth of Fresh Atmospheric Aerosols: Eight Years of Aerosol Size Distribution Data from SMEAR II, Hyytiala, Finland . Boreal Environ. Res. , 10 : 323 – 336 .
- Draxler , R. R. and Rolph , G. D. 2011 . “ HYSPLIT (HYbrid Single-Particle Lagrangian Integrated Trajectory) Model Access Via NOAA ARL Ready Website ” . (http://ready.arl.noaa.gov/HYSPLIT.php). NOAA Air Resources Laboratory, Silver Spring, MD
- Dusek , U. , Frank , G. P. , Curtius , J. , Drewnick , F. , Schneider , J. , Kurten , A. , Rose , D. , Andreae , M. O. , Borrmann , S. and Poschl , U. 2010 . Enhanced Organic Mass Fraction and Decreased Hygroscopicity of Cloud Condensation Nuclei (CCN) During New Particle Formation Events . Geophys. Res. Lett. , 37 : L03804 DOI: 03810.01029/02009gl040930
- Ehn , M. , Petaja , T. , Birmili , W. , Junninen , H. , Aalto , P. and Kulmala , M. 2007 . Non-Volatile Residuals of Newly Formed Atmospheric Particles in the Boreal Forest . Atmos. Chem. Phys. , 7 : 677 – 684 .
- Held , A. , Rathbone , G. J. and Smith , J. N. 2009 . A Thermal Desorption Chemical Ionization Ion Trap Mass Spectrometer for the Chemical Characterization of Ultrafine Aerosol Particles . Aerosol Sci. Technol. , 43 : 264 – 272 .
- Iida , K. , Stolzenburg , M. R. , McMurry , P. H. and Smith , J. N. 2008 . Estimating Nanoparticle Growth Rates from Size-Dependent Charged Fractions: Analysis of New Particle Formation Events in Mexico City . J. Geophys. Res.—Atmos. , 113 : D05207 DOI: 05210.01029/02007jd009260
- Kuang , C. , Riipinen , I. , Sihto , S. L. , Kulmala , M. , McCormick , A. V. and McMurry , P. H. 2010 . An Improved Criterion for New Particle Formation in Diverse Atmospheric Environments . Atmos. Chem. Phys. , 10 : 8469 – 8480 .
- Kulmala , M. , Vehkamaki , H. , Petaja , T. , Dal Maso , M. , Lauri , A. , Kerminen , V. M. , Birmili , W. and McMurry , P. H. 2004 . Formation and Growth Rates of Ultrafine Atmospheric Particles: A Review of Observations . J. Aerosol Sci. , 35 : 143 – 176 .
- Luther , G. W. and Stecher , H. A. 1997 . Preface: Historical Background . J. Geophys. Res.—Atmos. , 102 : 16215 – 16217 .
- McMurry , P. H. , Ghimire , A. , Ahn , H. K. , Sakurai , H. , Moore , K. , Stolzenburg , M. and Smith , J. N. 2009 . Sampling Nanoparticles for Chemical Analysis by Low Resolution Electrical Mobility Classification . Environ. Sci. Technol. , 43 : 4653 – 4658 .
- Merikanto , J. , Spracklen , D. V. , Mann , G. W. , Pickering , S. J. and Carslaw , K. S. 2009 . Impact of Nucleation on Global CCN . Atmos. Chem. Phys. , 9 : 8601 – 8616 .
- O’Dowd , C. D. , Yoon , Y. J. , Junkerman , W. , Aalto , P. , Kulmala , M. , Lihavainen , H. and Viisanen , Y. 2007 . Airborne Measurements of Nucleation Mode Particles I: Coastal Nucleation and Growth Rates . Atmos. Chem. Phys. , 7 : 1491 – 1501 .
- Riipinen , I. , Manninen , H. E. , Yli-Juuti , T. , Boy , M. , Sipila , M. , Ehn , M. , Junninen , H. , Petaja , T. and Kulmala , M. 2009 . Applying the Condensation Particle Counter Battery (CPCB) to Study the Water-Affinity of Freshly-Formed 2–9 nm Particles in Boreal Forest . Atmos. Chem. Phys. , 9 : 3317 – 3330 .
- Smith , J. N. , Barsanti , K. C. , Friedli , H. R. , Ehn , M. , Kulmala , M. , Collins , D. R. , Scheckman , J. H. , Williams , B. J. and McMurry , P. H. 2010 . Observations of Aminium Salts in Atmospheric Nanoparticles and Possible Climatic Implications . Proc. Natl. Acad. Sci. U.S.A. , 107 : 6634 – 6639 .
- Smith , J. N. , Dunn , M. J. , VanReken , T. M. , Iida , K. , Stolzenburg , M. R. , McMurry , P. H. and Huey , L. G. 2008 . Chemical Composition of Atmospheric Nanoparticles Formed from Nucleation in Tecamac, Mexico: Evidence for an Important Role for Organic Species in Nanoparticle Growth . Geophys. Res. Lett. , 35 : L04808 DOI: 04810.01029/02007gl032523
- Smith , J. N. , Moore , K. F. , Eisele , F. L. , Voisin , D. , Ghimire , A. K. , Sakurai , H. and McMurry , P. H. 2005 . Chemical Composition of Atmospheric Nanoparticles During Nucleation Events in Atlanta . J. Geophys. Res.—Atmos. , 110 : D22s03 DOI: 10.1029/2005jd005912
- Smith , J. N. , Moore , K. F. , McMurry , P. H. and Eisele , F. L. 2004 . Atmospheric Measurements of Sub-20 nm Diameter Particle Chemical Composition by Thermal Desorption Chemical Ionization Mass Spectrometry . Aerosol Sci. Technol. , 38 : 100 – 110 .
- Solomon , S. , Qin , D. , Manning , M. , Chen , Z. , Marquis , M. , Averyt , K. B. , Tignor , M. and Miller , H. L. 2007 . Climate Change 2007: The Physical Science Basis. Contribution of Working Group I to the Fourth Assessment Report of the Intergovernmental Panel on Climate Change , 996 Cambridge : Cambridge University Press .
- Spracklen , D. V. , Carslaw , K. S. , Kulmala , M. , Kerminen , V. M. , Sihto , S. L. , Riipinen , I. , Merikanto , J. , Mann , G. W. , Chipperfield , M. P. , Wiedensohler , A. , Birmili , W. and Lihavainen , H. 2008 . Contribution of Particle Formation to Global Cloud Condensation Nuclei Concentrations . Geophys. Res. Lett. , 35 : L06808 DOI: 06810.01029/02007gl033038
- Stecher , H. A. , Luther , G. W. , MacTaggart , D. L. , Farwell , S. O. , Crosley , D. R. , Dorko , W. D. , Goldan , P. D. , Beltz , N. , Krischke , U. , Luke , W. T. , Thornton , D. C. , Talbot , R. W. , Lefer , B. L. , Scheuer , E. M. , Benner , R. L. , Wu , J. G. , Saltzman , E. S. , Gallagher , M. S. and Ferek , R. J. 1997 . Results of the Gas-Phase Sulfur Intercomparison Experiment (GASIE): Overview of Experimental Setup, Results and General Conclusions . J. Geophys. Res.—Atmos. , 102 : 16219 – 16236 .
- Voisin , D. , Smith , J. N. , Sakurai , H. , McMurry , P. H. and Eisele , F. L. 2003 . Thermal Desorption Chemical Ionization Mass Spectrometer for Ultrafine Particle Chemical Composition . Aerosol Sci. Technol. , 37 : 471 – 475 .
- Wang , S. Y. and Johnston , M. V. 2006 . Airborne Nanoparticle Characterization with a Digital Ion Trap-Reflectron Time of Flight Mass Spectrometer . Int. J. Mass Spec. , 258 : 50 – 57 .
- Wang , S. Y. , Zordan , C. A. and Johnston , M. V. 2006 . Chemical Characterization of Individual, Airborne Sub-10-nm Particles and Molecules . Anal. Chem. , 78 : 1750 – 1754 .
- Zordan , C. A. , Pennington , M. R. and Johnston , M. V. 2010 . Elemental Composition of Nanoparticles with the Nano Aerosol Mass Spectrometer . Anal. Chem. , 82 : 8034 – 8038 .
- Zordan , C. A. , Wang , S. and Johnston , M. V. 2008 . Time-Resolved Chemical Composition of Individual Nanoparticles in Urban Air . Environ. Sci. Technol. , 42 : 6631 – 6636 .