Abstract
The ultrafine size fraction of ambient particles (ultrafine particles [UFP], diameter < 100 nm) has been identified as being particularly potent in their adverse health effects, yet, the detailed mechanisms for why UFP display such distinctive toxicity are not well understood. In the present study, mitochondria were exposed to ambient UFP while monitoring mitochondrial electron transport chain (ETC) activity as a model system for biochemical toxicity. UFP samples were collected in rural and urban environments, and chemically characterized for trace metals, ferrous (Fe(II)) and easily reducible ferric (Fe(III)) iron, polycyclic aromatic hydrocarbons (PAHs), and surface constituents with X-ray photoelectron spectroscopy (XPS). Fixed doses of UFP (8 μg mL−1) inhibited mitochondrial ETC function compared to controls in 94% of the samples after the 20 min of exposure. Significant moderate to weak correlations exist between initial %ETC inhibition (0 – 10 min) and Fe(II) (R = 0.55, P = 0.03, N = 15), anthracene (R = 0.74, P < 0.01, N = 13), and %C–O surface bonds (R = 0.56, P = 0.03, N = 15), whereby anthracene and %C–O correlate with each other (R = 0.58, P = 0.03, N = 14). Multivariate linear regression showed that when combined, Fe(II) and anthracene best describe the initial %ETC inhibition (R = 0.91, P = 0.00, N = 14). No significant associations were identified with total Fe and other trace metals. Results from this study indicate that Fe(II) and anthracene-related, C–O containing, surface structures may contribute to the initial detrimental behavior of UFP, also supporting the idea that the Fe(II)/Fe(III) and certain efficient hydroquinone/quinone redox pairs play important roles due to their potential to produce reactive oxygen species (ROS).
INTRODUCTION
A slew of epidemiological and toxicological studies over the past thirty years have established that inhalation of particulate matter (PM) emitted from combustion sources has negative effects on respiratory and cardiovascular health (Santacesaria et al. Citation1999; Pope III et al. 2002; de Kok et al. 2006; Schwarze et al. Citation2006; Ritz et al. Citation2007; Hoek et al. Citation2010), and more recently, particular attention has been directed toward the potent smallest fraction of these PM, namely the ultrafine particles (UFP, diameter < 100 nm) (Vinzents et al. Citation2005; Brauner et al. Citation2007; Londahl et al. Citation2009). UFP are primarily emitted from internal combustion engines as a result of incomplete combustion of fuels and condensation of volatilized constituents, and they are therefore ubiquitous in an urban environment. General consensus is that their detrimental health effect stems primarily from the combination of their minute size and particle surface functional groups, which allow UFP to reach deep into the alveolar region of the lung, facilitate their traverse through cell membranes and translocation to sensitive target organs, and induce oxidative stress that ultimately results in cell death (Kelly Citation2003; Li et al. Citation2003; Oberdörster et al. Citation2005). Despite a spur of diverse studies on this topic, the underlying chemical and biochemical mechanisms for UFP toxicity are not well characterized. The focus of the current study is to describe the role of iron speciation and surface functional groups in UFP toxicity.
The importance of small particle size on UFP chemical reactivity was confirmed in a recent review of nanoparticles’ role in Earth systems: the high degree of curvature on the surface leads to crystal lattice distortion and straining within the particle as well as on its surface (Hochella et al. Citation2008). As particles become smaller, their electronic structure and consequently their chemical and physical properties fail to follow classical rules and thus their behavior cannot be extrapolated from larger analogs. The second most important UFP characteristic leading to reactivity, i.e., the surface chemical aspect, has generally been correlated with chemical constituents that are able to catalyze the production of reactive oxygen species (ROS, i.e., OH•, HO2 •/O2 •−, H2O2). The two chemical groups identified as the main culprits are (i) trace metals (Wilson et al. Citation2002; Chen and Lippmann Citation2009), and (ii) aromatic hydrocarbons (Li et al. Citation2003; Xia et al. Citation2004). Not surprisingly, however, in a study where extractable components had been removed by organic solvent or acid washes, the UFP maintained their ability to catalyze the generation of ROS, indicating an inherent activity of the particle itself (Pan et al. Citation2004). This latter finding has been backed by the apparent correlation between the UFP bulk property of elemental carbon (EC) content and toxicity (Wilson et al. Citation2002; Garza et al. Citation2008).
The underlying mechanism by which UFP are assumed to be key players in the production of ROS is in catalyzing the otherwise enzymatically inhibited reduction of O2 by ubiquitous biologic reductants present in cells (Pan et al. Citation2004; Ntziachristos et al. Citation2007a; Vidrio et al. Citation2009). In this process, an effective redox active UFP surface compound, such as a trace metal or aromatic hydrocarbon, can temporarily take up an electron, which is subsequently supplied to O2, leading to production of a superoxide radical, O• 2, while regenerating the UFP surface catalyst. The initial electron donor could be a series of biological molecules, for instance those that partake in the regulation of the redox environment within the cell: glutathione, nicotinamide adenine dinucleotide phosphate (NADPH), dithiothreitol (DTT), and ascorbate (Ntziachristos et al. Citation2007a; Pan et al. Citation2004). Although the electron transfer from one such electron donor to O2 is thermodynamically favorable, this process is highly regulated by enzymes in a biological system. However, it is postulated that in the presence of UFP this regulatory system begins to fail (Schafer and Buettner Citation2001).
The presence of trace metals may further exacerbate the oxidative state in the cell by their ability to partake in additional fast and reversible redox processes that lead to ROS generation (Chen and Lippmann Citation2009). In this context, iron is of particular interest. First, once reduced to Fe(II), it is known to react with H2O2 (available from the disproportionation of 2 O•− 2) to produce the most powerful oxidant, hydroxyl radical, OH•, in the Fenton reaction (Vidrio et al. Citation2009). Second, iron is the most abundant transition metal present in ambient UFP (Lin et al. Citation2005; Geller et al. Citation2006), stemming from engine wear, lube oil additives, and in Europe also from fuel additives (Miller et al. Citation2007). Third, recent evidence from exposure studies confirms that iron and carbon act synergistically in producing ROS (Zhou et al. Citation2003; Waldman et al. Citation2007).
Although the total iron content in UFP is easily determined (Lin et al. Citation2005; Geller et al. Citation2006) its physicochemical state is not as readily characterized and its reactivity remains elusive. Hereto, the iron has generally been found to vary widely in time and space and as a mixture of metallic and oxidized iron (Weber et al. Citation2000; Yang et al. Citation2001; Kim et al. Citation2005; Lee et al. Citation2006; Miller et al. Citation2007). However, a number of studies performed in urban and remote environments have shown that significant proportions, a few to tens %, of iron are present as Fe(II), and that these concentrations far exceed those predicted from thermodynamic considerations (Pehkonen et al. Citation1993; Siefert et al. Citation1996; Johansen et al. Citation2000; Chen and Siefert Citation2004; Majestic et al. Citation2006). Processes that lead to increased Fe(II) are only partially known and include photochemical reduction of Fe(III) in the presence of organic molecules with carboxylic acid moieties and stabilization in acidic environment (Pehkonen et al. Citation1993; Siefert et al. Citation1994; Borer et al. Citation2005; Barbeau Citation2006; Key et al. Citation2008).
The importance of PAH in the toxicity of UFP by inducing inflammation and/or increasing oxidative stress capacity has been demonstrated in a number of studies (Bonvallot et al. Citation2001; Li et al. Citation2003; Li et al. Citation2009b; Zhang et al. Citation2009). In particular, it may be the cyclic dione moieties, i.e., hydroquinones/quinones, within these molecules that are responsible for the catalytic assistance in ROS production (Bolton et al. Citation2000; Sklorz et al. Citation2007). PAHs are released into the atmosphere primarily via incomplete combustion processes that may include both fossil fuel combustion and biomass burning (Ravindra et al. Citation2008). Their low volatilities favor condensation into the particle phase. Studies have demonstrated that a strong anthropogenic source of PAH is known to come from both diesel and gasoline emissions (Geller et al. Citation2006), and UFP PAH concentration has been correlated with early morning traffic in urban areas (Ning et al. Citation2007). This suggests that urban UFP may induce a greater toxic response than rural UFP.
Thus, despite indicators that a myriad of mechanisms are likely forming an intricate web of processes, fundamental knowledge gaps exist in both the identification of specific atmospheric components that play essential roles and in the underlying pathophysiological mechanisms that lead to mortality or morbidity. In the current investigation, in vitro experiments of the effect of UFP on the electron transport chain (ETC) functionality in isolated mitochondria, shed light on some of the effectors of mentioned UFP chemical characteristics and provide a more realistic subcellular measure of particle toxicity than the commonly used acellular alternative involving chemical reducing agents such as DTT and ascorbic acid (Ayres et al. Citation2008).
METHODS
UFP Collection
A high-volume collector (ChemVol 2400, Thermo Fisher Scientific Inc., Waltham, MA) was operated at an average flow rate of 760 L min−1 fractionating aerosols into four size fractions. The collector was designed initially by scientists at the Harvard School of Public Health for use in toxicological studies (Demokritou et al. Citation2002). Of particular importance to the current project are the smallest captured particles, which have an aerodynamic diameter of 100 nm and less (d50 < 100 nm, UFP). These particles are collected in a filtration step on a dense polypropylene filter of 17 cm diameter. Due to their low mass these particles are not easily separated from the gas stream and the ability of this collector to do so, at high sampling rate, makes it a unique piece of equipment that can collect substantial amounts of UFP during relatively short periods of time (days to 2 weeks, depending on atmospheric particle loadings). In the current study, samples were collected an average of 2 weeks, with average total collection volumes of 9.8 × 103 (±45%) m3.
The pumping system was connected to a datalogger and sector sampling system (CR1000, Campbell Scientific Inc., Logan, UT), which was configured to turn the collector off during rain events. Rural samples were collected in Ellensburg, which is located at the bottom of Kittitas Valley (Central Washington University, WA) where frequent formation of stable low lying inversion layers occur in the winter months and lead to the buildup of car exhaust and emissions from wood and pellet burning stoves (no significant industrial activities). The collector was set up on the roof of a university building in the NW corner of campus, in the NW part of the ∼12,000 inhabitant town. Since the predominant wind direction is from the NW, the sampling site is upwind of potential local pollutant sources. Urban samples were collected in Seattle approximately 150 m west of I-5, on the roof-top of the sciences building at North Seattle Community College. Of the 39 total samples that were collected between 2005 and 2008 over a time frame of 2.5 years, sixteen samples were used for this study. In most cases the filters were deemed unusable due to low particle loadings that did not provide sufficient material for the toxicity analyses. In other cases, the analyses were incomplete.
Extraction and Mass Determination of Ultrafine Particles
The mass of UFP collected on each polypropylene filter was determined in triplicate with the following technique derived from similar studies (Molinelli et al. Citation2002; R&P 2002; Li et al. Citation2003; Schins et al. Citation2004). Three 3.1 cm diameter circular sections were cut out of one of the 17.0 cm diameter polypropylene sample filters using a ceramic scalpel and polycarbonate stylist. Each section was placed in a separate acid-washed 10 mL Teflon container with the particle side facing down. Five mL of nano- pure water were added to each container along with a Teflon disk to ensure complete filter submersion in the water. Filters were sonicated for 10 min to transfer UFP into solution. During this process, particles are physically dislodged from the filter substrate, and while it is not expected that all particles come off, the assumption is made that a chemically and physically representative portion of the bulk UFP material is transferred into the solution. The liquid was removed from each Teflon container with an automatic pipet and transferred to a disposable syringe fitted with a 0.45 μm Acrodisc disposable syringe filter to remove filter derived particulate matter. Liquid was filtered into an aluminum weigh boat that had been preweighed on a microscale. Separate preweighed aluminum boats were used for each filter punch. The aluminum weigh boats containing UFP aliquots were partially covered and stored in a HEPA filtered clean hood until all water had evaporated. Aluminum boats were reweighed on a microscale, and UFP mass was determined by mass difference. For the in vitro experiments with mitochondria 3 new 3.1 cm filter sections were used for 3 replicates, whereby the amount of buffer added depended on the mass determination results so as to produce a consistent particle suspension of 8 ug of UFP per mL of buffer after filter sonication. This constant mass concentration represents the lower end of concentration ranges that displayed effects on mitochondria in in vitro experiments performed by other investigators (Li et al. Citation2003; Xia et al. Citation2004). Aqueous phase filter extracts of UFP were not analyzed for particle sizes. Thus, although no accumulation of agglomerated particles was visually detected over the course of the experiment, the assumption is that the portion of particle matter that was extracted is representative of the bulk UFP matter in chemical composition but perhaps not in final size distribution.
Trace Metal Analysis
Samples were analyzed for trace metals using an Inductively Coupled Plasma Mass Spectrometer (ICP-MS, X Series, Thermo Fisher Scientific Inc.). A 3-day acid digestion procedure preceded analysis to ensure the full decomposition of aluminosilicate matrices (Dillner et al. Citation2007). In short, predigestion with 30% H2O2 and HNO3 was followed by strong acid digestion involving HNO3-HCl-HF. All acids and the H2O2 were of ultrahigh purity grade (Baseline, Seastar Chemicals Inc., Sidney, British Columbia, Canada).
Quantification of elements was performed with a series of standards containing the following forty-four elements (High Purity Standards Inc., Charleston, SC): Be, Na, Mg, Al, K, Ca, Sc, Ti, Cr, V, Fe, Mn, Ni, Cu, Zn, Ga, Ge, As, Se, Rb, Sr, Zr, Mo, Cd, Sn, Cs, Ce, Ba, La, Nd, Sm, Eu, Gd, Tb, Dy, Ho, Er, Tm, Yb, Lu, Hf, Pb, Th, U. In addition, a 100 ppb acidified mixture of indium and bismuth was used as an internal standard to account for instrument drift, and quality control standards were run regularly. Out of 44 elements listed, the following were below detection limit (BDL) and thus removed from further analysis: Be, Ni, Ge, Se, and Cs.
Crustal enrichment factors are calculated in relation to average crustal abundances (Rudnick and Fountain Citation1999; Sun et al. Citation2001) with Al as the crustal proxy:
Labile Fe Species Analyses
Samples were analyzed for Fe(II) and easily reducible Fe(III) using the ferrozine technique and long pathlength absorbance spectroscopy (Stookey Citation1970; Waterbury et al. Citation1997; Chen and Siefert Citation2003). The complete setup (World Precision Instruments Inc., Sarasota, FL) consisted of a 202 ± 1 cm long Liquid Waveguide Capillary Cell (LWCC), a peristaltic pump (Peri-Star), a fiber optic light source (FO-6000), and a portable spectrometer (J&M TIDAS); all connected with fiber optic cables. The detection limit (DL) was determined to be 2.8 nM Fe(II) which translates into 0.010 to 0.042 ng m−3 atmospheric concentrations depending on air volume sampled and after applying appropriate statistics as outlined in the statistical treatment section.
Sections of samples were extracted by immersion in ultrapure water while gently shaking in the dark. Three forms of labile iron (labFe) were determined: (i) Fe(II)5min, for which an aliquot of the extracting solution was removed after 5 min, then complexed with ferrozine, and analyzed; (ii) Fe(II)15min, for which the original extractant solution containing the filter sample was acidified to a pH of 2 by HCl addition right after the Fe(II)5min aliquot had been removed, then, an additional aliquot was removed after 10 min extraction and analyzed analogously; and (iii) Fe(II) + Fe(III)red, for which hydroxylamine HCl had been added to the remaining extraction solution immediately after removal of the aliquot for Fe(II)15min, allowed to react for 15 min and then analyzed analogously. The role of the hydroxylamine is to reduce available Fe(III) to Fe(II), which provides a measure of the combined Fe(II) + Fe(III) pool that is potentially redox active. Following aliquot removal and before complexation with ferrozine, samples were filtered through 0.2 μm pore size membrane syringe filters (Acrodisc HT Tuffryn Membrane, Pall Corporation).
The last, most inclusive labile Fe determination represents a surrogate low measure for general Fe solubility, the latter of which is operationally defined as the Fe associated with particles that are smaller than 0.2 μm in diameter. The present measure is a low limit because nonreducible compounds, such as Fe alloys or Fe embedded within partices, are not detected. However, it is the redox active Fe pool that is likely more readily bioavailable, whereby in particular the Fe(II) fraction is metabolically preferred (Wells et al. Citation1995). Fe(II)5min was determined in all samples, while the other two measurements were performed on a subset of eleven samples.
PAH Analysis
Thirteen PAHs were analyzed in ultrafine aerosol samples with the help of a method adapted from Christensen et al. (2005). Briefly, samples were extracted from a 3.1 cm diameter punch from the polypropylene filter via sonication, purified with both a liquid–liquid extraction and solid phase extraction (SPE), preconcentrated via evaporation, and analyzed with a GC-FID (Agilent 8890).
Ultrafine aerosols were extracted via sonication in dichloromethane (DCM). Sonication was previously shown to be comparable to the commonly used Soxhlet Extraction (Christensen et al. Citation2005). Liquid was quantitatively transferred to a separate amber vial with a pipet. Preliminary sample purification was accomplished via liquid–liquid extraction with a 1:1 DCM:water solution. A microseparatory funnel was used to collect the DCM layer. Samples were further purified via normal-phase SPE with a selective extraction procedure using an Enviro Clean SPE column (6 mL, 1000 mg, aminopropyl unendcapped column, United Chemical Technologies). Column selection was based on personal communication with United Chemical Technologies representatives to most effectively retain impurities encountered in the sample. SPE columns were prewashed with 5 mL of DCM, samples were quantitatively transferred to the column, and PAHs were eluted with 5–6 mL of DCM. Prior to analysis, samples were preconcentrated to 0.5–0.75 mL by evaporation with filtered N2 gas. A 120 μl portion of sample was mixed with 30 μL of an internal standard (25 ng μL−1 D-10 deuterated acenaphthene) for quantification. Samples were run through an Agilent Series 6890 GC-FID equipped with a Innowax polyethylene glycol capillary (15 × 530 × 1) using the following method parameters: 2.0 μl splitless injection at 350°C, He flow at 19.3 mL min−1, and oven starting at 45°C for 4.00 min with a ramp of 10.0°C min−1 up to 325°C over 40 min. Total runtime was 44 min per sample with an equilibration time of 3 min. The FID detector was set at 340°C, with 40 mL min−1 H2 flow, 216 mL min−1 airflow, and 30 mL min−1 He makeup flow.
Surface Chemical Characterization with XPS
X-ray photoelectron spectroscopy (XPS) measurements were performed using a Phi 5000 VersaProbe and following previously published methods (Nachimuthu et al. Citation2009). In short, the system consists of a monochromatic focused Al Kα X-ray (1486.7 eV) source and a hemispherical analyzer. The X-ray beam is incident normal to the sample and the emitted photoelectrons are collected at an emission angle of 45º relative to the sample normal over a rastering area of 1.3 mm × 0.2 mm of the filter sample. XPS is a surface sensitive technique that provides quantitative abundance of elements and their chemical and electronic states in the uppermost 1–10 nm layer. The following elements were detected and quantified in the present samples: C, N, Si, O, and S. Of particular interest is the C1s band, as it can reveal the relative abundance of oxidized versus elemental carbon. The area under the C1s band, between approximately 291 and 282 eV binding energy, was curve fitted with 3 main contributing components: the C=O bond, the C–O bond, and the combined C–C and C–H bonds. Due to the large background in the C–C band that stems from the polypropylene filter material (very porous), the relative measures of oxidized carbon were also computed with the following example equation for the C–O singe bond (A stands for peak area):
Succinate Oxidase Activity Assay
A succinate oxidase activity (SOA) assay was used to assess electron transport function in mitochondria (Thomas et al. Citation1992; Thomas et al. Citation2001). Succinate oxidase (E.C. 1.3.5.1.) is an enzyme system that comprises Complexes II, III, and IV as well as ubiquinone and cytochrome c of the mitochondrial ETC, which represents 80% of the complete chain. Since oxygen is the final electron acceptor of succinate oxidase, enzyme activity can be measured by monitoring the disappearance of oxygen. For the present study, mitochondria were isolated from bovine heart tissue by differential centrifugation according to published methods (Blair Citation1967; Smith Citation1967). Mitochondrial activity and protein content were determined and concentrations for the in vitro experiments were set at 1 mg protein mL−1 of solution. Experiments were carried out in a 25 mL water-jacketed reaction flask controlled at a temperature of 25°C. The solution also contained 5 mM succinate to act as an electron donor for complex II in the ETC. At time (t) = 0 min, UFP were added to the solution to make a final concentration of 8.0 μg mL−1. At time points just before 0, 5, 10, 20, and 30 min, 0.5 mL of the exposed mitochondrial solution was added to a 1.6 mL capped reaction flask equipped with a Clark-type O2 electrode (YSI, Inc. Yellow Springs, OH) along with 0.08 mL 100 mM disodium succinate and 1.02 mL of 25 mM potassium phosphate buffer, pH 7.4. After the addition of succinate, initial rates of enzyme activity (oxygen consumption) were recorded. All solutions, i.e., buffers for isolating mitochondria, electron transport substrates, and reaction buffers, had been treated with Chelex-100 mesh resin to remove trace metals. To further minimize trace metal contamination, solutions were stored in acid cleaned polypropylene containers.
Oxygen consumption at each time point was plotted against time to evaluate changes in the rate of oxygen consumption over the first 30 min of exposure. Percent inhibition was then determined compared to a control, which consisted of the same mitochondria and reagents, however, the UFP control solution was prepared from an acid-washed blank filter. Since mitochondrial SOA in controls began to decline at the 30 min point, 2 measures were chosen as indicators for mitochondrial function: (i) %ETCInhibition0–10 min, the initial slope of change in oxygen consumption over 0–10 min as compared to the control without UFP, and (ii) %ETCInhibition20 min, the absolute rate of oxygen consumption at time 20 min compared to the control without UFP. Control experiments of UFP alone did not show significant O2 consumption.
Statistical Treatment of Data
Detection Limit and Error Analyses
Limits of detection were determined for each species and every sample. First, the instrument specific analytical DL was estimated from the frequently analyzed quality control (QC) standards by multiplying observed standard deviations for a given component with the Student t-value (for the typical sample number, N = 20, t 95% = 2.086). In addition, 5 field blanks were treated and analyzed as samples, which provided background concentrations that were subtracted. The DLs for species BDL were processed alongside all the other data in order to obtain an upper limit of the undetected species. Iron speciation and metals listed above were above detection limit (ADL) for all samples. In the case of PAHs, the percent of data points BDL ranged from 20% to 80% depending on PAH. In these cases, as is common procedure, the propagated DL was halved in order to be able to incorporate some estimate of the concentrations into the statistical analysis.
Triplicate extractions and analyses were performed for the mass determination, iron speciation, and mitochondrial assays, in which cases errors were determined from the associated standard deviations. ICP-MS and PAH were not performed in triplicate due to lack of sample substrate and errors were propagated following standard guidelines by including the following error estimates: (i) errors introduced from the use of calibration curves were calculated using the Excel function LINEST and associated standard deviation in the concentration (Harris Citation2007), and (ii) procedural errors from cutting of sample sections (2%, based on the differences in section weights), from the volume of air sampled (3%), and from errors of the various volumes used during extractions. Typical final relative errors propagated in such a way amounted to 10% for ICPMS and 50% for PAH analyses. Standard deviations (SD) reported with average values refer to the variance in the sample population and not the actual error estimates, as these latter estimates are small by comparison. However, SD for each data point is included as error bars in all scatter plots.
Volume Weighted Averages
Due to the 45% variation in air volume sampled between samples, volume weighted average concentrations are calculated to provide a more accurate estimate of the average atmospheric concentrations encountered at a given sampling site. The following formula was applied:
Student t-Tests
Two-tailed Student t-tests with unequal variances were performed on data sets from the two sampling sites to establish statistically significant differences based on the criterion that the probability value, P, ≤ 0.10. Paired Student t-tests were used to compare two measured components with each other, e.g., the various labile Fe forms.
Correlation and Multivariate Linear Regression Analyses
Correlation matrices were used in the interpretation of results. For this purpose, Pearson correlation coefficients, R, with two-tailed significance tests were computed on the combined sample set (SPSS 2008). Unless otherwise noted, correlations were deemed statistically significant only when P ≤ 0.05, i.e., 95% Confidence Interval (CI).
Multivariate linear regression analyses were performed to test the potential for using various independent variables, i.e., UFP chemical constituents, to describe the dependent variable, namely the degree of UFP toxicity, analogous to the procedure described in (Ntziachristos et al. Citation2007a).
RESULTS
Atmospheric concentrations of the various constituents are presented first and placed in context of reported analogous data. In order to compare these atmospheric concentrations to the toxicity data, which was determined at a constant UFP mass per mL of solution in the reaction vessel (i.e., 8.0 μg mL−1), the atmospheric data were normalized per mass of UFP extracted. Since the mass of UFP extracted was likely less than the total UFP analyzed for chemical constituents, the concentrations expressed on a per mass UFP basis are positively biased and therefore have no physical meaning. However, assuming that a chemically representative fraction of UFP was sonically transferred into solution for the mitochondrial assay, the relative associations between mass normalized data would be real. Observed relationships between these normalized measures are then presented subsequently.
Atmospheric Concentrations
Metal Concentrations
The seventeen most abundant metals in all samples are presented in in two separate stacked bar plots with different y-axis scales in order to better visualize the extreme low and high metal contributions. Ellensburg samples contained a larger amount of the major cations and typical crustal tracers such as Al and Fe (), while Seattle samples were characterized by larger abundances of the heavy metals, e.g., Cd, Sn, and Pb ( and b).
FIG. 1 Stacked bar plots of atmospheric trace metal concentrations [(a) high concentrations, (b) lower concentrations] in individual UFP samples collected in Ellensburg and Seattle. Sample ID is the Nominal Date of start of collection. Legend within the plot is organized in the same order as shadings appear in each bar.
![FIG. 1 Stacked bar plots of atmospheric trace metal concentrations [(a) high concentrations, (b) lower concentrations] in individual UFP samples collected in Ellensburg and Seattle. Sample ID is the Nominal Date of start of collection. Legend within the plot is organized in the same order as shadings appear in each bar.](/cms/asset/6266b50c-a7fd-4f7e-90db-eab7a7afe09e/uast_a_581255_o_f0001g.gif)
Since Ellensburg is situated in an arid, at times extremely windy, region that is characterized by glacial and volcanic ash deposits, it is likely that nanometer sized crustal dust particles are the source for the higher observed concentration of crustal metals (Hochella et al. Citation2008; Theng and Yuan Citation2008). In fact, enrichment factor analyses (EF) showed that all metals closely track the crustal tracer Al, and that only As and Cd were enriched in Ellensburg samples. Alternatively, fly ash from coal combustion may have also contributed to the crustal fingerprint, as previously observed in single particle analysis of Chinese aerosols (Gao and Anderson Citation2001). However, since the only existing coal fired power plant in WA is not within atmospheric transport's reach at the sampling site, this source is unlikely. Enrichment of Cd could be the result of preferential adsorption to nanometer sized crustal particles (Yuan et al. Citation2002). In Seattle, the list of enriched elements included, besides As and Cd, also Na, K, V, Cu, Zn, Sn, Ce, and Pb, whereby the largest relative enrichments were seen for Cu and Sn, which were 90 and 110 times more enriched in Seattle compared to Ellensburg, respectively. Corresponding values for Cd, As, V, Zn, and Pb ranged from 23 to 55.
Of particular interest was Cd due to its high concentrations: volume weighted averages amounted to 5.3 ± 3.1 ng m−3 in Ellensburg and 22.4 ± 22.0 ng m−3 in Seattle. Thus, for Ellensburg samples, this represents the upper limit of Cd concentrations estimated for ambient air in rural areas, i.e., 0.1–5 ng m−3, while the Seattle concentrations exceeded the corresponding range in urban areas, i.e., 2–15 ng m−3, falling instead within the range of 15–150 ng m−3 observed in industrialized areas (Elinder Citation1985; Friberg et al. Citation1992). Cd stems from the combustion of fossil fuels, the incineration of municipal waste, and zinc, lead, and copper smelting facilities.
In terms of Fe, as pointed out above, the predominant source in both Ellensburg and Seattle appeared to be mainly of crustal nature, and the respective volume weighted average concentrations of 106 ± 86 ng m−3 and 5.3 ± 7.2 ng m−3 show that Fe was twenty times more abundant in Ellensburg. These concentrations fall within the ranges of urban and remote levels (Lin et al. Citation2005; Ntziachristos et al. Citation2007b; Buck et al. Citation2010).
Labile Fe Concentrations
Fe(II)5min concentrations are presented in columns in as a function of sample ID, and superimposed in solid circles are the % relative contributions of Fe(II) to total Fe. Both absolute and relative values were within the range of sparsely reported similar data of UFP (Buck et al. Citation2010; Rastogi et al. Citation2009). The absolute Fe(II)5min concentrations were statistically significantly higher in Ellensburg, however, only by a factor of 2.3 as opposed to the analogous factor of twenty for total Fe. The resulting %Fe(II)5min/Total Fe values reflect the same picture in that Seattle samples average 4.2% and Ellensburg 0.5%. This type of pattern, where Fe solubilities are higher in samples with lower total Fe abundances, has been observed in bulk aerosol studies elsewhere and could be attributed to the differential physical and chemical processing and/or inherent different source characteristics of aerosols (Sedwick et al. Citation2007; Sholkovitz et al. Citation2009). In the present study, the detailed trace metal and EF analyses revealed that the source of Fe in both sets of samples was likely the same, i.e., crustal, which could imply that the observed difference in Fe(II) was a result of enhanced (photo)chemical processing in the more polluted Seattle samples.
FIG. 2 Fe(II)5min (columns) and %Fe(II)5min/Total Fe (solid circles) atmospheric concentrations for each UFP sample.

Further evidence for such processing is found in comparing the Fe(II)5min fraction with two additional analyses of labile Fe components that were performed on a subset of eleven samples: the 15 min acid- and the reductant-enhanced extractions. For comparative purposes, results for all three labile Fe determinations are presented in adjacent columns in . Statistically significant differences between the three data sets (paired student t-test) existed for Ellensburg samples between the Fe(II)5min and the Fe(II) + Fe(III)red measurements (P = 0.06), and for both Ellensburg and Seattle samples between the Fe(II)15min and the Fe(II) + Fe(III)red measurements (P = 0.01 and 0.02, respectively). In each of these cases, higher labile Fe concentrations resulted upon addition of acid or reductant. The relative difference between the two sites was most predominant in the increment from Fe(II)5min to Fe(II) + Fe(III)red concentrations: Ellensburg samples increased by a factor of 4.7 while Seattle samples remained essentially unchanged.
FIG. 3 Fe(II)5min, Fe(II)15min, and Fe(II) + Fe(III)red atmospheric concentrations for eleven UFP subsamples. Legend within plot.
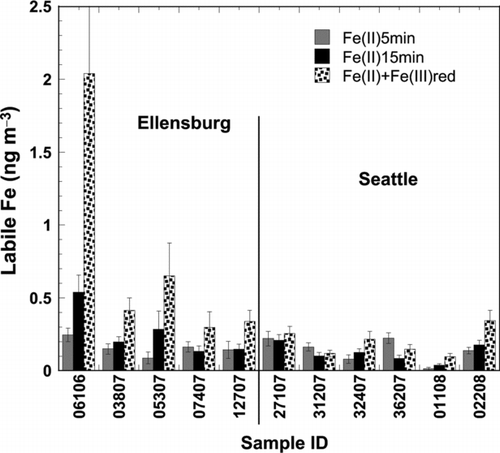
These observations support the idea that chemical processing of iron in a polluted environment is more effective, ultimately leading to the complete conversion of any available redox labile Fe(III) (i.e., Fe(III)red) to Fe(II). It is well known that photochemical reduction of Fe(III) in the presence of organic electron donors, in particularly those containing carboxylic acid moieties, leads to the accumulation of Fe(II) in aerosols (Pehkonen et al. Citation1993; Barbeau Citation2006; Johansen and Key Citation2006). Thus, it seems that (i) Seattle samples contained larger amounts of electron donors and/or were more acidic, the latter of which lead to the stabilization of reduced Fe(II) once formed, and (ii) Ellensburg samples contained substantial reducible Fe(III) that could perhaps have played a role in redox cycling and thus partook in the Fenton chemistry. The sum of unidentified peaks that eluted alongside the PAHs, correlated significantly with both the Fe(II)15min (R = 0.914, P = 0.000) and Fe(II) + Fe(III)red (R = 0.852, P = 0.002), which suggests that some Fe(II) and easily reducible Fe(III) was tied up in strong organic complexes as seen by various investigators (Kieber et al. Citation2005; Willey et al. Citation2005).
PAH Concentrations
Ultrafine particulate concentrations of 13 PAHs are presented in stacked bar plots in . These combined identified peak areas represented only 15 ± 14% of the combined peak area of organic compounds that eluted under the same analytical parameters as the PAHs. The unidentified peaks combined areas were treated alongside all other components in order to detect associations.
FIG. 4 Stacked bar plots of thirteen PAH atmospheric concentrations in each UFP sample. Shading of bars is organized by number of benzene rings, as indicated in the legend within the plot, and order of listing is the same as order of appearance in the bar.
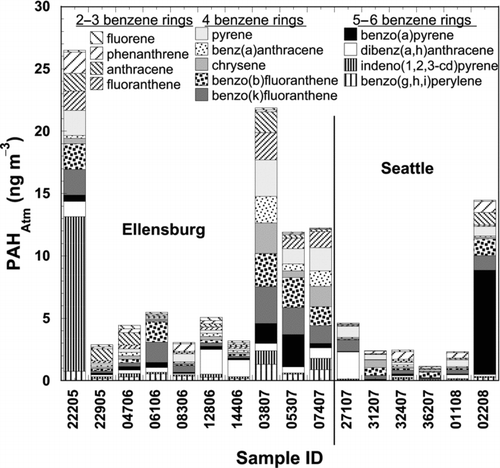
Total identified PAH concentrations in Ellensburg and Seattle were not statistically significantly different with volume weighted average concentrations of 9.8 ± 15.9 ng m−3 and 3.7 ± 6.0 ng m−3, respectively. This is in contrast to results presented in Allen et al. (1996) where PAH concentrations from rural samples were at least one order of magnitude less than urban samples. However, four of the ten rural Ellensburg samples were collected under atmospheric conditions that may have led to an increase in pollutant concentrations above normal rural levels. One was collected while forest and apartment fires were burning nearby (sample 22205) and 3 others were collected during the winter when stagnant inversion layers are common in the Kittitas Valley (samples 03807, 05307, and 07407). Moreover, the Seattle urban site experiences frequent rain events relative to the rural site, so removal of particles through wet deposition was more likely: in Seattle the collector was running an average of 77% of the sampling interval while the corresponding contribution in Ellensburg was 91% (rain events triggered automatic shut-off). Finally, it is likely that sampling artifacts may have contributed to the degradation of PAH by ozone in Seattle samples due to expected larger ozone concentrations in this urban environment (Schauer et al. Citation2003).
Total PAH concentrations presented here are in general agreement with the few published values for ultrafine particulate PAHs in locations elsewhere. Allen et al. (1996) reported 4.02 ng m−3 PAHs in ultrafine samples collected in Boston, MA, while Saarnio et al. (2008) detected ultrafine PAHs in 6 European cities that ranged from 0.170 ng m−3 in Barcelona (spring) to 8.90 ng m−3 in Prague (winter). Rural ultrafine values reported in Allen et al. (1996) were substantially lower than the rural values reported here.
Relative abundances of individual PAHs can be used as indicators of the combustion source (Ravindra et al. Citation2008), and upon visual inspection of , it appears that the contribution of the various PAHs was different across the board. The most abundant PAHs identified in the Ellensburg samples were the 4–5 benzene ring membered indeno(1,2,3-cd)pyrene, benzo(k)fluoranthene, and benzo(b)fluoranthene each with volume weighted average concentrations of 1.3 ± 1.0 ng m−3. The most abundant PAHs identified in Seattle were the 5 benzene ring membered benzo(a)pyrene, 1.1 ± 3.4 ng m−3, and benzo(b)fluoranthene, 0.5 ± 0.5 ng m−3. All of these compounds are on the list of sixteen priority United States Environmental Protection Agency (US EPA) PAHs (ATSDR 1995), and exist primarily in the particle phase. Higher molecular weight PAHs, containing 5–6 benzene rings, are often attributed to light duty (gasoline) vehicle emissions (Wingfors et al. Citation2001), however, light duty vehicles have also been shown to significantly contribute to 4-ringed PAH emissions (Marr et al. Citation1999). This suggests that the predominant source in both locations may have been gasoline vehicle traffic, which is also supported by the fluorene/(fluorene + pyrene) diagnostic ratio with values of 0.12 and 0.14 for Ellensburg and Seattle, respectively, as ratios less than 0.5 are associated with gasoline emissions while ratios greater than 0.5 are associated with diesel emissions (Ravindra et al. Citation2008). Additional diagnostic PAH ratios showed conflicting results, implying that the PAHs are emitted from a variety of additional sources at both locations.
XPS Surface Chemical Results
Amongst all the XPS surface chemical analyses results, those obtained for the C1s band fitting revealed to be the most interesting. Although other constituents were measured, including N, O, Si, and S, the variances between samples were not sufficient to derive significant relationships. Relative contributions of the C–O single and C=O double bonds to the total C1s band are presented in the column plot in . Since these data are normalized to particle mass, they are not directly comparable with atmospheric per m3 concentrations, however, they are compatible for comparative purposes with the toxicity data. Two statistically significant observations can be made from this data: (i) Ellensburg samples contained 1.7 times more C–O bonds relative to total C than Seattle samples, and (ii) the C=O contribution to the combined oxidized C amounted to approximately 35 ± 7% in Seattle samples and 29 ± 9% in Ellensburg samples (Student t-test for significant difference, P = 0.16). In summary, larger relative amounts of C were found as C=O than C–O in Seattle relative to Ellensburg samples. These results are in line with the enhanced chemical processing of Seattle particles deduced for the Fe speciation. In the case of the organic C, however, chemical processing would be associated with a higher degree of oxidation, e.g., by photooxidants (Lee Citation2003; Eiguren-Fernandez et al. Citation2008).
FIG. 5 XPS determined and curve fitted %C1s peak contributions to oxidized carbon in each UFP sample.
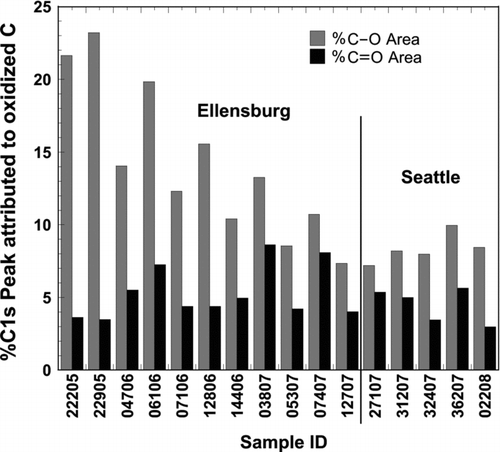
A significant correlation between anthracene and %C-O may be suggestive of an oxidized component in the UFP that was associated with anthracene. Since PAH oxidation leads to the formation of their hydroquinone- and quinone-like derivatives (Lee Citation2003; Eiguren-Fernandez et al. Citation2008), one possibility is that anthracene tracks the actual (not measured) anthrahydroquinone concentration, while another possibility is that for no apparent reason, anthracene and the sum of all hydroquinone type moieties are correlated.
ETC Inhibition and Correlations with Atmospheric Constituents
ETC Inhibition
Initial (%ETCInhibition0–10 min) and 20 min (%ETCInhibition20 min) ETC inhibition, was significant in 73% and 94% of the sixteen samples, respectively. Both determinations are presented in columns in . Due to the low number of Seattle samples and the large overall variance, differences were not statistically significant between Ellensburg and Seattle samples. Averages (ranges) were 31 ± 32% (BDL-83%) and 21 ± 17% (BDL-53%) for %ETCInhibition0–10 min and %ETCInhibition20 min, respectively, and since the 2 measures were deemed sufficiently different (paired student t-test, P = 0.12) they were both included in further analyses.
Correlations with Atmospheric Constituents
Different associations were observed for the initial and 20 min measures of ETC inhibition with the various atmospheric constituents measured. The %ETCInhibition0–10 min correlated significantly with Fe(II)5min (R = 0.55, P = 0.03, N = 15), anthracene (R = 0.74, P < 0.01, N = 13), and %C-O surface bonds (R = 0.56, P = 0.03, N = 15). Out of these three components, only anthracene and %C-O correlate with each other (R = 0.58, P = 0.03, N = 14). The fact that these correlations were weak to moderate is an indication that no sole component is a good descriptor of mitochondrial dysfunction and that instead 2 or more UFP parameters are likely contributing to the cause. Thus, bivariate linear regressions were performed to explore linearly additive effects of independent component pairs, e.g., Fe(II)5min/anthracene and Fe(II)5min/%C-O. While the latter pair did not result in statistically significant results, the model for the Fe(II)/anthracene pair of dependent variables deemed statistically significant (P = 0.00) with an R2 = 0.837 (see upper half of for model output including figures of merit). The results are also visualized in , where stacked bars represent the calculated contribution of %ETCInhibition for each of the dependent variables and the solid circles are the observed values. The standardized coefficients of 0.42 and 0.55 for Fe(II)5min and anthracene, respectively, provide an estimate of the relative contributions by each component and are thus indicative of both compounds playing similar roles in the initial inhibition of the ETC.
TABLE 1 Multivariate linear regression model outputs
FIG. 7 Modeled initial %ETCInhibition attributed to Fe(II)5min and anthracene (both in stacked bars) and observed %ETCInhibition (solid circles). Figures of merit for these data are show in the top half of .

In the case of the %ETCInhibition at 20 min the most predominant correlation was observed with the sum of PAHs (R = 0.601, P = 0.023), however, a multivariate linear regression model that included Fe(II)5min resulted in an improved statistical description of the observed %ETCInhibition: R 2 = 0.778 and P = 0.000 (see second half of ). Analogous to , the results for this model output are presented in in stacked bars overlain by the observed values in solid circles. The standardized coefficients of 0.43 and 0.53 for Fe(II)5min and the sum of PAHs, respectively, also draw a similar picture compared to the initial inhibition, in that the contribution of each component is comparable in magnitude. Association of the 20 min %ETCInhibition was also observed with 2 individual PAHs, namely phenanthrene (R = 0.593, P = 0.026) and indeno(1,2,3-cd)pyrene (R = 0.578, P = 0.03), but multivariate linear regression analyses involving any combination of these components instead of the sum of PAHs did not lead to significant model results.
FIG. 8 Modeled 20-min %ETCInhibition attributed to Fe(II)5min and sum of PAHs (both in stacked bars) and observed %ETCInhibition (solid circles). Figures of merit for these data are show in the second half of .
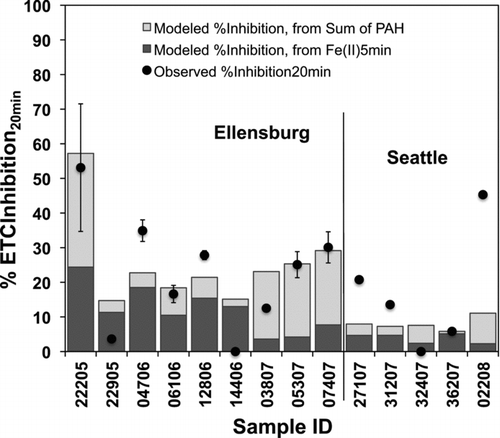
%ETCInhibition did not correlate with other trace metals or with pooled molar concentrations of typical redox active metals (i.e., Fe, Cu, Mn, Ni, V, Cr, Co) or otherwise catalytic metals (i.e., Zn, Pb, Cd, Al).
DISCUSSION
UFP toxicity dependence on PAHs, or PAH derivatives, and trace metals has been attributed mainly to the secondary formation of damaging ROS in the presence of biological electron donors (Wilson et al. Citation2002; Xia et al. Citation2004; Park et al. Citation2006; Bekki et al. Citation2009; Chen and Lippmann Citation2009; Li et al. Citation2009a; Nawrot et al. Citation2009; Shinyashiki et al. Citation2009). The present study is consistent with those results, while revealing more specific novel and potentially important mechanistic information that implicates particular PAHs and trace metal speciation. It is the anthracene (and/or potentially correlating related C-O containing organic molecules, such as anthraquinone and anthrahydroquinone) and Fe(II)5min that emanate as the key players in this regard. These two components are known to be efficient producers of ROS: Fe(II), in the Fenton Reaction with H2O2 produces OH•, and anthrahydroquinone, in the presence of an electron donor and O•− 2 produces H2O2, a reaction also used on an industrial scale for H2O2 production (Santacesaria et al. Citation1999). A simplified schematic representing the potential interconnected web of reactions that involves the consumption and production of the oxidative stress inducing ROS (in bold) is shown in .
FIG. 9 Simplified schematic of suggested ROS producing mechanisms involving anthraquinone/anthrahydroquinone and Fe(III)/Fe(II).
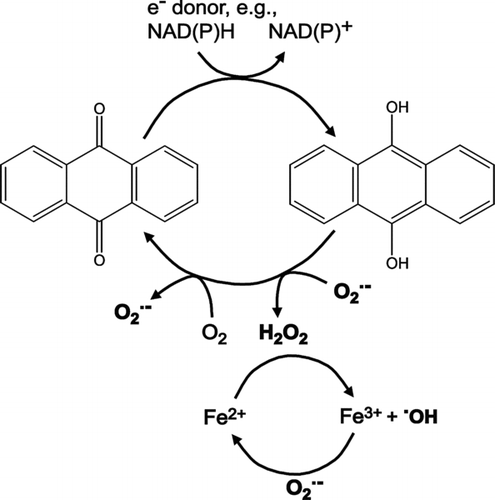
A synergistic effect arises (i) from the production of H2O2 in the first step of hydroquinone to quinone which leads to OH• formation in the presence of Fe(II), and (ii) during the second step of the hydroquinone oxidation where O•− 2 is regenerated to either initiate the oxidation of another hydroquinone or reduce Fe(III).
Of particular value is the fact that of the thirteen analyzed PAHs, anthracene shows the most prevalent effect on ETC inhibition during the initial period. Anthracene is readily and consistently photooxidized at positions 9 and 10, which display some degree of nonaromaticity and thus are susceptible to radical addition. The products are the corresponding ROS generating 9,10-anthrahydroquinone and 9,10-anthraquinone, also shown in (Santacesaria et al. Citation1999; Cordeiro and Corio Citation2009). Similar significance of ROS formation in ambient particulate matter collected in Germany was found for the polycyclic aromatic monoketones (e.g., benz(de)anthracene-7-one) (Sklorz et al. Citation2007).
The fact that only the Fe(II)5min portion of the various labile Fe entities correlated with the initial %ETCInhibition and neither of the two other labile Fe fractions, indicates that Fenton chemistry may be playing a predominant role during the initial stages of ROS formation. At the 20 min time point, additional processes seem to gain in importance: Fe(II)5min still seems to contribute significantly to the modeled %ETCInhibition20 min, while the more prevalent association with the sum of PAHs, and in part with phenanthrene and indeno(1,2,3-cd)pyrene, may indicate that their roles, or that of other compounds that are coemitted or coproduced, become increasingly important with UFP exposure time. These results suggest that at least two separate processes are at play during the inhibition of the ETC.
Assuming that Fe(II)5min and the PAHs as identified in the present study are directly or indirectly involved in the ETC disruption, the mole based coefficients listed in provide an estimate of their relative mechanistic importance. For the initial %ETCInhibition, the coefficients of 2.05 and 4.66, for Fe(II)5min and anthracene, respectively, suggest that on a per mole basis, the anthracene contributes greater than two times to the %ETCInhibition than Fe(II)5min. Analogous evaluation of the 20 min %ETCInhibition reveals that the Fe(II)5min, with a mole based coefficient of 1.35, contributes in excess of 6 times to the %ETCInhibition compared to the molar sum of PAHs, with a mole based coefficient of 0.21. However, since it is likely that some of the PAHs do not contribute to the inhibition (Santacesaria et al. Citation1999) it leads to inflating the relative significance of Fe(II)5min. Nevertheless, this result indicates that if PAHs are quantified and summed up as a group and used as such as an indicator for toxicity, their per mole contribution to toxicity may seem to be significantly less than that of Fe(II).
Interestingly, the two sets of rural and urban samples collected in this study showed significantly different chemical makeup, yet, the degree of toxicity on mitochondria did not reveal a clear association with either group. In particular, the presence of elevated trace metals of crustal origin in the nanometer sized range, albeit of natural origin (Hochella et al. Citation2008; Theng and Yuan Citation2008), seemed to be influencing the adverse effects of UFP mode significantly in rural samples, while in urban samples the higher degree of chemical processing as shown by the relatively higher contribution of Fe(II) and oxidized C, may have been the key contributor.
CONCLUSIONS
The relative toxicity of a range of atmospheric UFPs was determined successfully by testing the in vitro function of a subcellular organelle, the mitochondrion. Despite the disparate chemical makeup of sixteen analyzed samples from urban and regional environments in WA, no statistically significant toxicity differences could be identified between the two sample sets. This discrepancy could be attributed to the elevated (photo)chemical processing in the more polluted urban samples that lead to more active components, namely oxidized surface C and readily soluble Fe(II). The anthracene, which correlated with oxidized surface C, and the Fe(II)5min showed the most significant correlations with %ETCInhibition during the first 10 min of UFP exposure. A multivariate linear regression model with anthracene and Fe(II)5min as the two dependent variables resulted in a relative molar %ETCInhibition of anthracene that amounted to ∼2 times that imposed by Fe(II)5min. After 20 min of exposure, the role of other PAHs became more important than initially, with Fe(II)5min still contributing significantly to the overall %ETCInhibition.
Although no direct causal relationship can be proven without additional detailed experiments, present results, in agreement with published data (Wilson et al. Citation2002; Xia et al. Citation2004; Park et al. Citation2006; Bekki et al. Citation2009; Chen and Lippmann Citation2009; Li et al. Citation2009a; Nawrot et al. Citation2009; Shinyashiki et al. Citation2009), indicate that it is the associated generation of ROS that induces observed adverse effects on mitochondrial function. Even in the absence of a proven mechanistic process, however, results may provide the means to establish relative mitochondrial toxicity of UFP based on two key and specific measurable UFP chemical parameters: Fe(II) and anthracene. Furthermore, since mitochondrial function is representative of a slew of biochemical processes, its use as a surrogate model for UFP toxicity shows great potential and may be more representative than the one-dimensional redox activity tests involving simple electron donors (Ayres et al. Citation2008).
Finally, in light of the data generated in the current study, more work is needed (i) to increase understanding of the atmospheric relationships with anthracene and of the processes that contribute to Fe(III) reduction in UFPs, and (ii) to identify the detailed mechanisms that disrupt ETC function.
Acknowledgments
The work performed in this study was supported by the National Institutes of Health R15 ES013952 AREA grant and Central Washington University. A portion of the research was performed using EMSL, a national scientific user facility sponsored by the Department of Energy's Office of Biological and Environmental Research located at Pacific Northwest National Laboratory.
Notes
aMass unit is based on %inhibition/(mg Fe or PAH/g UFP).
bMol unit is based on %inhibition/(umol Fe or PAH/g UFP).
REFERENCES
- Allen , J. O. , Dookeran , K. M. , Smith , K. A. , Sarofim , A. F. , Taghizadeh , K. and Lafleur , A. L. 1996 . Measurement of Polycyclic Aromatic Hydrocarbons Associated with Size-Segregated Atmospheric Aerosols in Massachusetts . Environ. Sci. Technol. , 30 : 1023 – 1031 .
- ATSDR . 1995 . Toxicological Profile for Polycyclic Aromatic Hydrocarbons , Atlanta, GA : Agency for Toxic Substances and Disease Registry .
- Ayres , J. G. , Borm , P. , Cassee , F. R. , Castranova , V. , Donaldson , K. , Ghio , A. , Harrison , R. M. , Hider , R. , Kelly , F. , Kooter , I. , Marano , F. , Maynard , R. L. , Mudway , I. , Nel , A. , Sioutas , C. , Smith , S. , Baeza-Squiban , A. , Cho , A. , Duggan , S. and Froines , J. 2008 . Evaluating the Toxicity of Airborne Particulate Matter and Nanoparticles by Measuring Oxidative Stress Potential–A Workshop Report and Consensus Statement . Inhal. Toxicol. , 20 : 75 – 99 .
- Barbeau , K. 2006 . Photochemistry of Organic Iron(III) Complexing Ligands in Oceanic Systems . Photoch. Photobio. , 82 : 1505 – 1516 .
- Bekki , K. , Takigami , H. , Suzuki , G. , Tang , N. and Hayakawa , K. 2009 . Evaluation of Toxic Activities of Polycyclic Aromatic Hydrocarbon Derivatives Using in Vitro Bioassays . J. Health Sci. , 55 : 601 – 610 .
- Blair , P. V. 1967 . The Large-Scale Preparation and Properties of Heart Mitochondria from Saughterhouse Material . Methods Enzymol. , 10 : 78 – 81 .
- Bolton , J. L. , Trush , M. A. , Penning , T. M. , Dryhurst , G. and Monks , T. J. 2000 . Role of Quinones in Toxicology . Chem. Res. Toxicol. , 13 : 135 – 160 .
- Bonvallot , V. , Baeza-Squiban , A. , Baulig , A. , Brulant , S. , Boland , S. , Muzeau , F. , Barouki , R. and Marano , F. 2001 . Organic Compounds from Diesel Exhaust Particles Elicit a Proinflammatory Response in Human Airway Epithelial Cells and Induce Cytochrome p450 1A1 Expression . Am. J. Resp. Cell. Mol. Biol. , 25 : 515 – 521 .
- Borer , P. M. , Sulzberger , B. , Reichard , P. and Kraemer , S. M. 2005 . Effect of Siderophores on the Light-Induced Dissolution of Colloidal Iron(III) (Hydr)oxides . Mar. Chem. , 93 : 179 – 193 .
- Brauner , E. V. , Forchhammer , L. , Moller , P. , Simonsen , J. , Glasius , M. , Wahlin , P. , Raaschou-Nielsen , O. and Loft , S. 2007 . Exposure to Ultrafine Particles from Ambient Air and Oxidative Stress-Induced DNA Damage . Environ. Health Persp. , 115 : 1177 – 1182 .
- Buck , C. S. , Landing , W. M. , Resing , J. A. and Measures , C. I. 2010 . The Solubility and Deposition of Aerosol Fe and Other Trace Elements in the North Atlantic Ocean: Observations from the A16N CLIVAR/CO2 Repeat Hydrography Section . Mar. Chem. , 120 : 57 – 70 .
- Chen , L. C. and Lippmann , M. 2009 . Effects of Metals within Ambient Air Particulate Matter (PM) on Human Health . Inhal. Toxicol.: Int Forum Resp. Res. , 21 : 1 – 31 .
- Chen , Y. and Siefert , R. L. 2003 . Determination of Various Types of Labile Atmospheric Iron Over Remote Oceans . J. Geophys. Res.-Atmos. , 108
- Chen , Y. and Siefert , R. L. 2004 . Seasonal and Spatial Distributions and Dry Deposition Fluxes of Atmospheric Total and Labile Iron Over the Tropical and Subtropical North Atlantic Ocean . J. Geophys. Res.-Atmos. , 109
- Christensen , A. , Ostman , C. and Westerholm , R. 2005 . Ultrasound-Assisted Extraction and On-Line LC-GC-MS for Determination of Polycyclic Aromatic Hydrocarbons (PAH) in Urban Dust and Diesel Particulate Matter . Anal. Bioanal. Chem. , 381 : 1206 – 1216 .
- Cordeiro , D. S. and Corio , P. 2009 . Electrochemical and Photocatalytic Reactions of Polycyclic Aromatic Hydrocarbons Investigated by Raman Spectroscopy . J. Brazil Chem. Soc. , 20 : 80 – 87 .
- de Kok , T. , Driece , H. A. L. , Hogervorst , J. G. F. and Briede , J. J. 2006 . Toxicological Assessment of Ambient and Traffic-Related Particulate Matter: A Review of Recent Studies . Mutat. Res.-Rev. Mutat , 613 : 103 – 122 .
- Demokritou , P. , Kavouras , I. G. , Ferguson , S. T. and Koutrakis , P. 2002 . Development of a High Volume Cascade Impactor for Toxicological and Chemical Characterizaion Studies . Aerosol Sci. Technol. , 36 : 925 – 933 .
- Dillner , A. M. , Shafer , M. M. and Schauer , J. J. 2007 . A Novel Method Using Polyurethane Foam Plug (PUF) Substrates to Determine Trace Element Concentrations in Size-Segregated Atmospheric Particulate Matter on Short Time Scales . Aerosol Sci. Technol. , 41 : 75 – 85 .
- Eiguren-Fernandez , A. , Miguel , A. H. , Lu , R. , Purvis , K. , Grant , B. , Mayo , P. , Di Stefano , E. , Cho , A. K. and Froines , J. 2008 . Atmospheric Formation of 9,10-Phenanthraquinone in the Los Angeles Air Basin . Atmos. Environ. , 42 : 2312 – 2319 .
- Elinder , C.-G. 1985 . “ Cadmium: Uses, Occurrence, and Intake ” . In Cadmium and Health: A Epidemiological and Toxicological Appraisal , Edited by: Friberg , L. , Elinder , C.-G. , Nordberg , G. F. and Kjellstrom , T. 23 – 63 . Boca Raton, Florida : CRC Press, Inc. . vol. 1
- Friberg , L. , Elinder , C. G. and Kjellström , T. 1992 . Cadmium: Environmental Health Criteria 134 , Geneva : World Health Organization, IPCS (International Programme on Chemical Safety) .
- Gao , Y. and Anderson , J. R. 2001 . Characteristics of Chinese aerosols Determined by Individual-Particle Analysis . J. Geophys. Res.-Atmos. , 106 : 18037 – 18045 .
- Garza , K. M. , Soto , K. F. and Murr , L. E. 2008 . Cytotoxicity and Reactive Oxygen Species Generation from Aggregated Carbon and Carbonaceous Nanoparticulate Materials . Int. J. Nanomed. , 3 : 83 – 94 .
- Geller , M. D. , Ntziachristos , L. , Mamakos , A. , Samaras , Z. , Schmitz , D. A. , Froines , J. R. and Sioutas , C. 2006 . Physicochemical and Redox Characteristics of Particulate Matter (PM) Emitted from Gasoline and Diesel Passenger Cars . Atmos. Environ. , 40 : 6988 – 7004 .
- Harris , D. C. 2007 . Quantitative Chemical Analysis , New York : W. H. Freeman .
- Hochella , M. F. , Lower , S. K. , Maurice , P. A. , Penn , R. L. , Sahai , N. , Sparks , D. L. and Twining , B. S. 2008 . Nanominerals, Mineral Nanoparticles, and Earth systems . Science , 319 : 1631 – 1635 .
- Hoek , G. , Boogaard , H. , Knol , A. , De Hartog , J. , Slottje , P. , Ayres , J. G. , Borm , P. , Brunekreef , B. , Donaldson , K. , Forastiere , F. , Holgate , S. , Kreyling , W. G. , Nemery , B. , Pekkanen , J. , Stone , V. , Wichmann , H. E. and Van Der Sluijs , J. 2010 . Concentration Response Functions for Ultrafine Particles and All-Cause Mortality and Hospital Admissions: Results of a European Expert Panel Elicitation . Environ. Sci. Technol. , 44 : 476 – 482 .
- Johansen , A. M. and Key , J. M. 2006 . Photoreductive Dissolution of Ferrihydrite by Methanesulfinic Acid: Evidence of a Direct Link Between Dimethylsulfide and Iron-Bioavailability . Geophys. Res. Lett. , 33
- Johansen , A. M. , Siefert , R. L. and Hoffmann , M. R. 2000 . Chemical Composition of Aerosols Collected Over the Tropical North Atlantic Ocean . J. Geophys. Res. , 105 : 15277 – 15312 .
- Kelly , F. J. 2003 . Oxidative Stress: Its Role in Air Pollution and Adverse Health Effects . Occup. Environ. Med. , 60 : 612 – 616 .
- Key , J. M. , Paulk , N. and Johansen , A. M. 2008 . Photochemistry of Iron in Simulated Crustal Aerosols with Dimethyl Sulfide Oxidation Products . Environ. Sci. Technol. , 42 : 133 – 139 .
- Kieber , R. J. , Skrabal , S. A. , Smith , B. J. and Willey , J. D. 2005 . Organic Complexation of Fe(II) and Its Impact on the Redox Cycling of Iron in Rain . Environ. Sci. Technol. , 39 : 1576 – 1583 .
- Kim , S. H. , Fletcher , R. A. and Zachariah , M. R. 2005 . Understanding the Difference in Oxidative Properties between Flame and Diesel Soot Nanoparticles: The Role of Metals . Environ. Sci. Technol. , 39 : 4021 – 4026 .
- Lee , D. , Miller , A. , Kittelson , D. and Zachariah , M. R. 2006 . Characterization of Metal-Bearing Diesel Nanoparticles Using Single-Particle Mass Spectrometry . J. Aerosol. Sci. , 37 : 88 – 110 .
- Lee , R. F. 2003 . Photo-Oxidation and Photo-Toxicity of Crude and Refined Oils . Spill Sci. Technol. Bull. , 8 : 157 – 162 .
- Li , N. , Sioutas , C. , Cho , A. , Schmitz , D. , Misra , C. , Sempf , J. , Wang , M. , Oberley , T. , Froines , J. R. and Nel , A. 2003 . Ultrafine Particulate Pollutants Induce Oxidative Stress and Mitochondrial Damage . Environ. Health Persp. , 111 : 455 – 460 .
- Li , N. , Wang , M. Y. , Bramble , L. A. , Schmitz , D. A. , Schauer , J. J. , Sioutas , C. , Harkema , J. R. and Nel , A. E. 2009a . The Adjuvant Effect of Ambient Particulate Matter Is Closely Reflected by the Particulate Oxidant Potential . Environ. Health Persp. , 117 : 1116 – 1123 .
- Li , Z. , Porter , E. N. , Sjodin , A. , Needham , L. L. , Lee , S. , Russell , A. G. and Mulholland , J. A. 2009b . Characterization of PM2.5-Bound Polycyclic Aromatic Hydrocarbons in Atlanta-Seasonal Variations at Urban, Suburban, and Rural Ambient Air Monitoring Sites . Atmos. Environ. , 43 : 4187 – 4193 .
- Lin , C.-C. , Chen , S.-J. , Huang , K.-L. , Hwang , W.-I. , Chang-Chien , G.-P. and Lin , W.-Y. 2005 . Characteristics of Metals in Nano/Ultrafine/Fine/Coarse Particles Collected Beside a Heavily Trafficked Road . Environ. Sci. Technol. , 39 : 8113 – 8122 .
- Londahl , J. , Massling , A. , Swietlicki , E. , Brauner , E. V. , Ketzel , M. , Pagels , J. and Loft , S. 2009 . Experimentally Determined Human Respiratory Tract Deposition of Airborne Particles at a Busy Street . Environ. Sci. Technol. , 43 : 4659 – 4664 .
- Majestic , B. J. , Schauer , J. J. , Shafer , M. M. , Turner , J. R. , Fine , P. M. , Singh , M. and Sioutas , C. 2006 . Development of a Wet-Chemical Method for the Speciation of Iron in Atmospheric Aerosols . Environ. Sci. Technol. , 40 : 2346 – 2351 .
- Marr , L. C. , Kirchstetter , T. W. , Harley , R. A. , Miguel , A. H. , Hering , S. V. and Hammond , S. K. 1999 . Characterization of Polycyclic Aromatic Hydrocarbons in Motor Vehicle Fuels and Exhaust Emissions . Environ. Sci. Technol. , 33 : 3091 – 3099 .
- Miller , A. L. , Stipe , C. B. , Habjan , M. C. and Ahlstrand , G. G. 2007 . Role of Lubrication Oil in Particulate Emissions from a Hydrogen-Powered Internal Combustion Engine . Environ. Sci. Technol. , 41 : 6828 – 6835 .
- Molinelli , A. R. , Madden , M. C. , McGee , J. K. , Stonehuerner , J. G. and Ghio , A. J. 2002 . Effect of Metal Removal on the Toxicity of Airborne Particulate Matter from the Utah Valley . Inhal. Toxicol. , 14 : 1069 – 1086 .
- Nachimuthu , P. , Kim , Y. J. , Kuchibhatla , S. , Yu , Z. Q. , Jiang , W. , Engelhard , M. H. , Shutthanandan , V. , Szanyi , J. and Thevuthasan , S. 2009 . Growth and Characterization of Barium Oxide Nanoclusters on YSZ(111) . J. Phys. Chem. C , 113 : 14324 – 14328 .
- Nawrot , T. S. , Kuenzli , N. , Sunyer , J. , Shi , T. M. , Moreno , T. , Viana , M. , Heinrich , J. , Forsberg , B. , Kelly , F. J. , Sughis , M. , Nemery , B. and Borm , P. 2009 . Oxidative Properties of Ambient PM2.5 and Elemental Composition: Heterogeneous Associations in 19 European Cities . Atmos. Environ , 43 : 4595 – 4602 .
- Ning , Z. , Geller , M. D. , Moore , K. F. , Sheesley , R. , Schauer , J. J. and Sioutas , C. 2007 . Daily Variation in Chemical Characteristics of Urban Ultrafine Aerosols and Inference of their Sources . Environ. Sci. Technol. , 41 : 6000 – 6006 .
- Ntziachristos , L. , Froines , J. , Cho , A. and Sioutas , C. 2007a . Relationship between Redox Activity and Chemical Speciation of size-Fractionated Particulate Matter . Part. and Fibre Toxicol. , 4 : 5
- Ntziachristos , L. , Ning , Z. , Geller , M. D. , Sheesley , R. J. , Schauer , J. J. and Sioutas , C. 2007b . Fine, Ultrafine and Nanoparticle Trace Element Compositions Near a Major Freeway with a High Heavy-Duty Diesel Fraction . Atmos. Environ. , 41 : 5684 – 5696 .
- Oberdörster , G. , Oberdörster , E. and Oberdörster , J. 2005 . Nanotoxicology: An Emerging Discipline Evolving from Studies of Ultrafine Particles . Environ. Health Persp. , 113 : 823 – 839 .
- Pan , C. J. G. , Schmitz , D. A. , Cho , A. K. , Froines , J. and Fukuto , J. M. 2004 . Inherent Redox Properties of Diesel Exhaust Particles: Catalysis of the Generation of Reactive Oxygen Species by Biological Reductants . Toxicol. Sci. , 81 : 225 – 232 .
- Park , S. , Nam , H. , Chung , N. , Park , J. D. and Lim , Y. 2006 . The Role of Iron in Reactive Oxygen Species Generation from Diesel Exhaust Particles . Toxicol. in Vitro , 20 : 851 – 857 .
- Pehkonen , S. O. , Siefert , R. , Erel , Y. , Webb , S. and Hoffmann , M. 1993 . Photoreduction of Iron Oxyhydroxides in the Presence of Important Atmospheric Organic Compounds . Environ. Sci. Technol. , 27 : 2056 – 2062 .
- Pope , C. A. III , Burnett , R. T. , Thun , M. J. , Calle , E. E. , Krewski , D. , Ito , K. and Thurston , G. D. 2002 . Lung Cancer, Cardiopulmonary Mortality, and Long-term Exposure to Fine Particulate Air Pollution . JAMA , 287 : 1132 – 1141 .
- R&P . 2002 . ChemVol Model 2400 Operating Manual, Draft , Albany, NY : Rupprecht & Patashnik Co., Inc. .
- Rastogi , N. , Oakes , M. M. , Schauer , J. J. , Shafer , M. M. , Majestic , B. J. and Weber , R. J. 2009 . New Technique for Online Measurement of Water-Soluble Fe(II) in Atmospheric Aerosols . Environ. Sci. Technol. , 43 : 2425 – 2430 .
- Ravindra , K. , Sokhi , R. and Van Grieken , R. 2008 . Atmospheric Polycyclic Aromatic Hydrocarbons: Source Attribution, Emission Factors and Regulation . Atmos. Environ. , 42 : 2895 – 2921 .
- Ritz , B. , Wilhelm , M. , Hoggatt , K. J. and Ghosh , J. K. C. 2007 . Ambient Air Pollution and Preterm Birth in the Environment and Pregnancy Outcomes Study at the University of California, Los Angeles . Am. J. Epidemiol. , 166 : 1045 – 1052 .
- Rudnick , R. L. and Fountain , D. M. 1999 . Nature and Composition of the Continental Crust: A Lower Crustal Perspective . Rev. Geophys. , 33 : 267 – 309 .
- Saarnio , K. , Sillanpaa , M. , Hillamo , R. , Sandell , E. , Pennanen , A. S. and Salonen , R. O. 2008 . Polycyclic Aromatic Hydrocarbons in Size-Segregated Particulate Matter from Six Urban Sites in Europe . Atmos. Environ. , 42 : 9087 – 9097 .
- Santacesaria , E. , Di Serio , M. , Russo , A. , Leone , U. and Velotti , R. 1999 . Kinetic and Catalytic Aspects in the Hydrogen Peroxide Production Via Anthraquinone . Chem. Eng. Sci. , 54 : 2799 – 2806 .
- Schafer , F. Q. and Buettner , G. R. 2001 . Redox Environment of the Cell as Viewed through the Redox State of the Glutathione Disulfide/Glutathione Couple . Free. Radical. Bio. , 30 : 1191 – 1212 .
- Schauer , C. , Niessner , R. and Pöschl , U. 2003 . Polycyclic Aromatic Hydrocarbons in Urban Air Particulate Matter: Decadal and Seasonal Trends, Chemical Degradation, and Sampling Artifacts . Environ. Sci. Technol. , 37 : 2861 – 2868 .
- Schins , R. P. F. , Lightbody , J. H. , Borm , P. J. A. , Shi , T. M. , Donaldson , K. and Stone , V. 2004 . Inflammatory Effects of Coarse and Fine Particulate Matter in Relation to Chemical and Biological Constituents . Toxicol. Appl. Pharm. , 195 : 1 – 11 .
- Schwarze , P. E. , Ovrevik , J. , Lag , M. , Refsnes , M. , Nafstad , P. , Hetland , R. B. and Dybing , E. 2006 . Particulate Matter Properties and Health Effects: Consistency of Epidemiological and Toxicological Studies . Hum. Exp. Toxicol. , 25 : 559 – 579 .
- Sedwick , P. N. , Sholkovitz , E. R. and Church , T. M. 2007 . Impact of Anthropogenic Combustion Emissions on the Fractional Solubility of Aerosol Iron: Evidence from the Sargasso Sea . Geochem Geophys Geosyst. , 8
- Shinyashiki , M. , Eiguren-Fernandez , A. , Schmitz , D. A. , Di Stefano , E. , Li , N. , Linak , W. P. , Cho , S. H. , Froines , J. R. and Cho , A. K. 2009 . Electrophilic and Redox Properties of Diesel Exhaust Particles . Environ. Res. , 109 : 239 – 244 .
- Sholkovitz , E. R. , Sedwick , P. N. and Church , T. M. 2009 . Influence of Anthropogenic Combustion Emissions on the Deposition of Soluble Aerosol Iron to the Ocean: Empirical Estimates for Island Sites in the North Atlantic . Geochim. Cosmochim. Acta , 73 : 3981 – 4003 .
- Siefert , R. L. , Pehkonen , S. O. , Erel , Y. and Hoffmann , M. R. 1994 . Iron Photochemistry of Aqueous Suspensions of Ambient Aerosol with Added Organic Acids . Geochim. Cosmochim. Acta. , 58 : 3271 – 3279 .
- Siefert , R. L. , Webb , S. M. and Hoffmann , M. R. 1996 . Determination of Photochemically Available Iron in Ambient Aerosols . J. Geophys. Res. , 101 : 14441 – 14449 .
- Sklorz , M. , Briede , J. J. , Schnelle-Kreis , J. , Liu , Y. , Cyrys , J. , de Kok , T. M. and Zimmermann , R. 2007 . Concentration of Oxygenated Polycyclic Aromatic Hydrocarbons and Oxygen Free Radical Formation from Urban Particulate Matter . J. Tox. Env. Health.-Pt. A-Current Issues , 70 : 1866 – 1869 .
- Smith , A. L. 1967 . Preparation, Properties, and Conditions for Assay of Mitochondria: Slaughterhouse Material, Small-Scale . Methods Enzymol. , 10 : 81 – 86 .
- SPSS . 2008 . SPSS Statistics , Chicago : SPSS Inc. .
- Stookey , L. L. 1970 . Ferrozine-A New Spectrophotometric Reagent for Iron . Anal. Chem. , 42 : 779 – 781 .
- Sun , Y. C. , Po-hsiang , C. and Shiue , M. 2001 . Comparison of Different Digestion Methods for Total Decomposition of Siliceous and Organic Environmental Samples . Anal. Sci. , 17 : 1395 – 1399 .
- Theng , B. K. G. and Yuan , G. 2008 . Nanoparticles in the Soil Environment . ELEMENTS , 4 : 395 – 399 .
- Thomas , C. , MacGill , R. S. , Miller , G. C. and Pardini , R. S. 1992 . Photoactivation of Hypericin Generates Singlet Oxygen in Mitochondria and Inhibits Succinoxidase . Photochem.Photobiol. , 55 : 47 – 53 .
- Thomas , C. , Will , Y. , Schoenberg , S. L. , Sanderlin , D. and Reed , D. J. 2001 . Conjugative Metabolism of 1,2-Dibromoethane in Mitochondria: Disruption of Oxidative Phosphorylation and Alkylation of mtDNA . Biochem. Pharmacol. , 61 : 595 – 603 .
- Vidrio , E. , Phuah , C. H. , Dillner , A. M. and Anastasio , C. 2009 . Generation of Hydroxyl Radicals from Ambient Fine Particles in a Surrogate Lung Fluid Solution . Environ. Sci. Technol. , 43 : 922 – 927 .
- Vinzents , P. S. , Moller , P. , Sorensen , M. , Knudsen , L. E. , Hertel , O. , Jensen , F. P. , Schibye , B. and Loft , S. 2005 . Personal Exposure to Ultrafine Particles and Oxidative DNA Damage . Environ. Health Persp. , 113 : 1485 – 1490 .
- Waldman , W. J. , Kristovich , R. , Knight , D. A. and Dutta , P. K. 2007 . Inflammatory Properties of Iron-Containing Carbon Nanoparticles . Chem. Res. Toxicol. , 20 : 1149 – 1154 .
- Waterbury , R. D. , Yao , W. and Byrne , R. H. 1997 . Long Pathlength Absorbance Spectroscopy: Trace Analysis of Fe(II) Using a 4.5 m Liquid Core Waveguide . Anal. Chim. , 357 : 99 – 102 .
- Weber , S. , Hoffmann , P. , Ensling , J. , Dedik , A. N. , Weinbruch , S. , Miehe , G. , Gutlich , P. and Ortner , H. M. 2000 . Characterization of Iron Compounds from Urban and Rural Aerosol Sources . J. Aerosol. Sci. , 31 : 987 – 997 .
- Wells , M. L. , Price , N. M. and Bruland , K. W. 1995 . Iron Chemistry in Seawater and Its Relationship to Phytoplankton: A Workshop Report . Mar. Chem. , 48 : 157 – 182 .
- Willey , D. , Whitehead , R. F. , Kieber , R. J. and Hardison , D. R. 2005 . Oxidation of Fe(II) in Rainwater . Environ. Sci. Technol. , 39 : 2579 – 2585 .
- Wilson , M. R. , Lightbody , J. H. , Donaldson , K. , Sales , J. and Stone , V. 2002 . Interactions Between Ultrafine Particles and Transition Metals In Vivo and In Vitro . Toxicol. Appl. Pharm. , 184 : 172 – 179 .
- Wingfors , H. , Sjodin , A. , Haglund , P. and Brorstrom-Lunden , E. 2001 . Characterisation and Determination of Profiles of Polycyclic Aromatic Hydrocarbons in a Traffic Tunnel in Gothenburg, Sweden . Atmos. Environ. , 35 : 6361 – 6369 .
- Xia , T. , Korge , P. , Weiss , J. N. , Li , N. , Venkatesen , M. I. , Sioutas , C. and Nel , A. 2004 . Quinones and Aromatic Chemical Compounds in Particulate Matter Induce Mitochondrial Dysfunction: Implications for Ultrafine Particle Toxicity . Environ. Health Persp. , 112 : 1347 – 1358 .
- Yang , G. S. , Teague , S. , Pinkerton , K. and Kennedy , I. M. 2001 . Synthesis of an Ultrafine iron and Soot Aerosol for the Evaluation of Particle Toxicity . Aerosol Sci. Technol. , 35 : 759 – 766 .
- Yuan , G. , Percival , H. J. , Theng , B. K. G. and Parfitt , R. L. 2002 . “ Sorption of Copper and Cadmium by Allophane-Humic Complexes ” . In Developments in Soil Science , Edited by: Violante , A. , Huang , P. M. , Bollag , J.-M. and Gianfreda , L. 37 – 47 . Elsevier Science B.V. .
- Zhang , Y. X. , Tao , S. , Shen , H. Z. and Ma , J. M. 2009 . Inhalation Exposure to Ambient Polycyclic Aromatic Hydrocarbons and Lung Cancer Risk of Chinese Population . Proc. Natl. Acad. Sci. USA , 106 : 21063 – 21067 .
- Zhou , Y. M. , Zhong , C. Y. , Kennedy , I. M. , Leppert , V. J. and Pinkerton , K. E. 2003 . Oxidative Stress and NF Kappa B Activation in the Lungs of Rats: A Synergistic Interaction between Soot and Iron Particles . Toxicol. Appl. Pharm. , 190 : 157 – 169 .