Abstract
Control strategies such as variations in injection pressure and timing have been used by researchers to reduce in-cylinder exhaust emissions and meet legislation standards. Postinjection has been studied for several years and is now well known as an efficient strategy for reducing soot emission. Diesel gaseous and particle mass emissions have been progressively reduced over the last twenty years as a consequence of increasingly restrictive emission legislation and the application of aftertreatment devices. The main objective of this work is to better understand the effect of postinjection on particle size distribution in diesel exhaust. The approach uses a modern, well-instrumented research engine test cell equipped with a flexible high pressure fuel injection system. The results of this work provide guidelines for developing strategies to reduce particle size distribution in diesel engines. A major improvement in particle size distribution was found in the accumulation mode by using a close postinjection of a small quantity of fuel. For reduction in nucleation mode, a relationship was found with close postinjections of large quantities of fuel.
INTRODUCTION
Diesel engines have evolved considerably in recent decades. This is one of the reasons why they have become the most used automotive powertrain in Europe. Huge improvements in diesel injection systems have been part of this success story (CitationAnrigo 2006). The common rail system is the most common injection system used in HSDI diesel engines. This system enables flexible control of the injection parameters such as injection pressure, timing, duration, and number of injections (Guerrassi and Dupraz 1998). By being able to select the number of injections and the split of those injections, additional freedom is gained in the possible methods for performing injection events, and this greatly contributes to the process of achieving optimized solutions for any operating conditions. Furthermore, common rail systems can use a wide range of injection pressures and this extends the potential for improving combustion efficiency and reducing exhaust emissions in modern diesel engines.
TABLE 1 Engine operating conditions used in the current research
In mixing-controlled combustion, injection and combustion processes are coupled, and so injection timing and pressure are highly influential parameters for emission formation. Engine efficiency can be increased by injecting the precise amount of fuel that is needed in a well-atomized spray that enhances the mixing processes. When injection pressure is increased, the flame lift-off (the distance from the injector nozzle to the start of the combustion region) also increases. This leads to more air-entrainment before the fuel reaches the flame zones, resulting in better mixing of the fuel with the surrounding air. As a result, leaner conditions are obtained upstream of the reaction zone and so higher heat release rates and peak temperatures are reached (CitationPickett and Siebers 2004). This leaner mixture upstream of the flame reduces the amount of unburned hydrocarbons [HC (a soot precursor)] formed in the rich premixed burn region that is located between the liquid length (close to the injector nozzle) and the diffusion flame front. As a result, a lower soot production rate is obtained. Additionally, higher peak temperatures in the diffusion flame front increase the soot oxidation process—resulting in lower total engine-out soot emissions.
The use of postinjection represents an additional strategy to decrease soot emissions, while reducing other pollutant emissions at the same time. It is known from previous investigations, that the use of postinjection strategies has an influence on soot oxidation and/or soot formation processes (CitationArregle et al. 2008; Heywood 1988). It was established that the behavior of soot emissions associated with postinjection depends mainly on the temperature of the unburned gases at the end of injection of the postinjection. There is a temperature threshold below which there will be no soot formation, independently of the postinjection size, while the amount of soot increases as the temperature rises beyond this threshold. This increase is caused by a rise in temperature that leads, in turn, to an increase in the fuel/air ratio at the lift-off length. Hence, the scope of this work is focused on these postinjection strategies.
As stated above, soot trends for variations in injection strategies (injection pressure and post injections) have been analyzed in detail in previous research. Nevertheless, there is increasing concern regarding the relationship between the number of particles and particle size distribution.
Experimental studies have shown an increase in particle numbers in new diesel engines (CitationBaumgard and Johnson 1996). As a result, further investigations have been published concerning the effect of some injection strategies on particle size distribution (CitationDesantes et al. 2006; Baumgard and Johnson 1996; Kreso et al. 1998; CitationBertola et al. 2001). Several hypotheses have been formulated to explain the increased number of particles observed in new diesel engines, and it is thought that there are two major causes: a higher concentration of volatile nanoparticle precursors (HC, SO2), and a decrease in mass of accumulation mode particles.
Based on this background, the motivation and objective of this work is to further understand the effect of postinjection strategies on particle size distributions.
EXPERIMENTAL
Test Methodology
The experiments presented in this paper were all performed on a single-cylinder engine under three different engine operating conditions as shown in . The operating conditions were selected to show the effect of different postinjection strategies on particle size distribution. The first operating condition is at low engine speed and 75% load (termed A75 mode). Particle size distribution for this mode is dominated by nucleation. The second operating condition (termed B50 mode) is at medium engine speed and 50% load, and this condition shows a bimodal distribution for particle size distribution. The third and last condition (termed C25) is at high engine speed and low load (25%). In this condition, particle size distribution is dominated by accumulation.
The methodology for the experimental tests is based on a comparison between simple injection and postinjection strategy tests, using the same quantity of injected fuel mass in both cases. For this purpose, a set of tests, hereafter called the test set, is defined, and composed of the following:
• | A reference test corresponding to a simple injection strategy M (main injection) under specific engine conditions. | ||||
• | Several tests of multiple injections, M* + P (shortened main + post injection). The mass of the postinjection P was removed from the main injection. The injection timing is the only parameter that is changed in the different postinjection tests in a given test set. Also the gap between the main injection and the postinjection (ΔαP) is changed in such a way that ΔαP = 0 corresponds to a postinjection and a main injection that start to overlap. |
To better understand the methodology explained above, an example of a test set is given in .
FIG. 1 Schemes of the different test included in a test set: A reference case (M), and several postinjections cases (M* + F) with different timings for P.
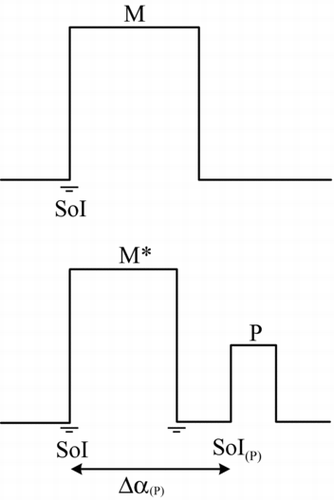
The number of tests in each test set depends on the number of SoI (start of injection) variations in the P pulse for the postinjection cases. In the frame of the current study, the number of postinjection cases is never less than five. In each operating condition, the start of postinjection [SoI (p)] is swept from 15°CAD after the top dead center (°aTDC) to 40°CAD in steps of 5°CAD. In some cases, the maximum angle of postinjection was 35°aTDC because of an observed increase in carbon monoxide (CO) and total hydrocarbon (THC) emissions and problems with engine stability.
In the case of A75 operating mode, the mass of the postinjection was set at 10, 8, and 4 mg/stk for each test set. In the case of B50 operating mode, the mass of the postinjection was set at 8, 4, and 2 mg/stk for each test set. And finally, in the case of C25, the mass of the postinjection was set at 6, 4, and 2 mg/stk for each test. According to the procedure explained above, the tests are repeated in order to check repeatability; hence the total number of tests is around 36 for each test set.
Engine and Fuel Properties
The study was carried out using a research single-cylinder, four-stroke, direct-injection diesel engine, derived from a PSA® DV6 1.6HDi engine. The engine displacement volume was 0.4 L and it was equipped with a 1600 bar, solenoid-controlled common rail injection system. Further detailed specifications of the engine are given in . The engine was installed in a fully equipped test cell, with all the auxiliary devices required for correct engine operation and control. This test cell was designed with the criteria detailed by CitationPlint and Martyr (1999).
TABLE 2 Research light-duty engine specifications
Ultra low sulfur diesel (ULSD) fuel was used for all the experiments. The fuel properties are provided in .
TABLE 3 Ultra Low-Sulphur Diesel specifications
General Instrumentation
In-cylinder pressure was measured with a Kistler 6043A pressure transducer. The pressure traces for 25 engine cycles were recorded in order to compensate for the cycle-to-cycle variation during engine operation (Lancaster et al. 1975). The heat release law (HRL) was obtained by means of a filtered and averaged pressure signal. For this purpose, an in-house combustion diagnostic code called CALMEC was used (Lapuerta et al. 1999; Lapuerta et al. 2000). This code is based on the resolution of the energy equation applied to the cylinder under the assumption of a uniform pressure and temperature across the whole combustion chamber volume. This single-zone model approach makes it possible to calculate the instantaneous mean temperature, as well as the instantaneous heat release from the burned fuel. With simple additional hypotheses (Ishida et al. 1996; CitationGarcía et al. 2000) the single-zone model can be extended to a two-zone model that provides information on the instantaneous temperature of burned and unburned gas fractions.
Exhaust emissions were acquired using a HORIBA 7100DEGR gas analyzer. The sample line of the equipment was connected directly to the exhaust pipe and was heated to maintain a wall temperature of around 191°C to avoid the condensation of hydrocarbons in the line. The hydrocarbon analyzer was a heated flame ionization detector (HFID). Nitrogen oxides (NOx) were measured on a dry basis using a heated chemiluminescent detector (HCLD) with an NO2/NO converter. The CO was measured with a nondispersive infrared (NDIR) absorption-type analyzer, based on the property of infrared light absorption of CO molecules. Soot emissions were measured with an AVL 415 smoke meter that provided results directly in FSN. This instrument uses a reflectometer to compare the blackness of the filtered carbonaceous-particle portion of the exhaust sample with an empirical correlation for filter smoke number (FSN) in a reference scale 0–10. These measurements were transformed into mg/m3 using the correlation proposed in the device user manual:
Additionally, in some cases the injection rate was also measured using a method developed by CitationPayri et al. (2008).
Particle Size Distribution Measurement Protocol
A single-stage partial flow dilution system was coupled with an scanning mobility particle sizer (SMPS) to measure particle size distributions. The measurement systems for particle emission measurement are briefly described later.
A minitunnel AVL-SPC 472 dilution system was used—consisting of a heated tunnel 635 mm in length and with a 30 mm inner diameter. The dilution ratios achievable with this system range from 4 to 40 for 0.2–2 g/s of total dilution flow. At the specification of the dilution ratio and the total flow (constant flow), the sum of the dilution air and the extracted fraction of the exhaust flow is pumped using a pumping system.
The SMPS is a widely used instrument for measuring the size distribution of submicron particles in a gas. The operating principle of the SMPS is based on the extraction of an original polydisperse aerosol in different monodisperse fractions by separating the particles according to their size as determined by their electrical mobility inside an electrical field. The extracted monodispersed particle fractions are counted in a condensation particle counter that provides the number of particles per air volume unit for each particle size.
A TSI SMPS-3936 was equipped with an inertial impactor to remove particles larger than 1 μm, as well as a long differential mobility analyzer (LDMA) with a range from 5.6 to 550 nm. After selecting the specific particle size to be measured by the LDMA, the particles were counted in a CPC with d50: 10 nm.
To extract a diluted sample for the particle size measurement, the SMPS was connected downstream of the dilution system. The sampling point where the sample probe of the SMPS is connected is considered to be an influencing factor for particle size measurements, since the mixing conditions between dilution air and exhaust determine particle dynamics and, as a result, affect particle size measurements (CitationSuresh and Johnson 2001; Tow et al. 1994) To ensure uniform mixing of the dilution air and the extracted sample flow, the filter holder piece after the dilution tunnel (which is normally used for the gravimetric analysis) was chosen as the location for connecting the 6 mm inner diameter perforated—type tube sample probe. The SMPS system was positioned to obtain the shortest possible sampling line (≈ m) to reduce losses in the sampling tube. Diffusion losses at a particle size of 10 nm are below 7%—assuming laminar flow and using the equation of diffusion losses in a cylindrical tube proposed by Hinds using a model tube of 1 m with an inner diameter of 8 mm and a temperature of 400 K.
RESULTS AND DISCUSSION
Combustion Process
For the different operating conditions tested, normalized brake specific fuel consumption (BSFC) versus postinjection ΔαP is shown in . The BSFC trends for the various postinjection quantities (tested for each operating mode) are shown in comparison with the single injection nominal case (dotted horizontal line at the value of 1). Independently of the ΔαP between main injection and postinjection, no variation or only slight variations of BSFC are observed for the smaller postinjection quantities (as seen in modes A75 and B50). For the case of C25, a significant increment in BSFC is observed for the small and large postinjection quantities and for large ΔαP values. This is mainly because the mass of the postinjection represents a high percentage of the total injected mass, and also because the postinjection timing is delayed in the expansion stroke and this results in lower temperatures for the unburned gases and lower oxidation rates. For the largest postinjection quantities in each operating mode (10, 8, and 6 mg/stk for A75, B50, and C25, respectively) an increase in BSFC that is related to the increase of ΔαP or delay is observed for all three cases; and the highest increase in BSFC corresponds to the C25 mode with a ratio of 1.8 times the nominal case. This trend can also be explained by the reasons given earlier (high percentage of postinjection mass and lower temperatures with longer delays).
FIG. 2 The BSFC for all operating conditions, small postinjected fuel mass do not affect the fuel consumption, on the opposite large postinjected fuel mass increase the penalty in fuel consumption.
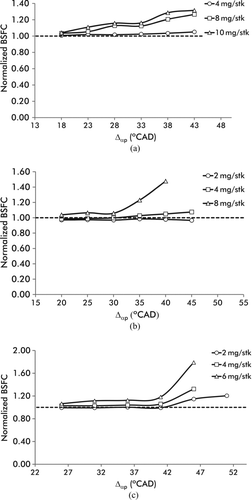
FIG. 3 Thermodynamic analysis results from the three operating modes and using different postinjected mass fuel at different Δαp.
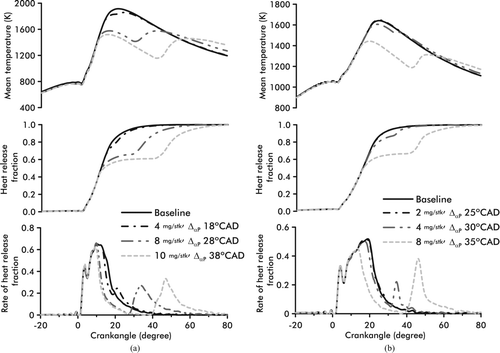
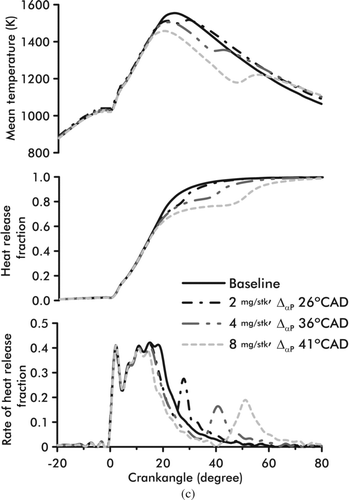
In , the instantaneous values (in engine °CA) for the mean temperature, the normalized HRL, and the normalized rate of heat release (HRR) are shown for the three operating modes and various postinjected quantities with different ΔαP values.
In the HRL graph, an imaginary line at the point of 0.8 on the vertical axis (which means that 80% of the mixture is completely burned) is traced, then this line is intersected with each curve, and the corresponding crank angle degree is found; this angle represents the combustion duration for each postinjection quantity. Based on this analysis, it can be clearly seen that for increasing values of postinjection quantities the duration of the combustion increases, therefore, the end of combustion is delayed relative to TDC.
For small postinjection quantities, the combustion duration is relatively similar to the baseline case, which implies similar temperature and pressure profiles, and so a similar efficiency with respect to the reference is achieved–and, therefore, a similar specific fuel consumption. This confirms the small differences in BFSC observed in for the smaller postinjected quantities.
For larger postinjection quantities, the combustion duration increases, so further delaying the combustion relative to TDC and implying lower instantaneous temperature and pressure profiles. This results in a worse indicated efficiency, and as a direct consequence, the penalty on fuel consumption is increased.
Pollutant Emissions
In this section the results of pollutant emissions are discussed to illustrate the effect of various postinjection quantities, as well as the effects of ΔαP injection between the main and postinjection on the emission of HC, CO, NOX, and soot.
Regarding the CO and THC emissions, in , the normalized HC and CO emissions for the B50 operating mode are compared to the reference case for single injection. This operating condition was chosen only for visualization purposes, the results observed for the other two modes (A75 and C25) also show similar trends.
FIG. 4 The CO and THC engine-out emissions for operating mode B50. No significant incomplete combustions seem to be achieved with the late postinjections explored in this study.
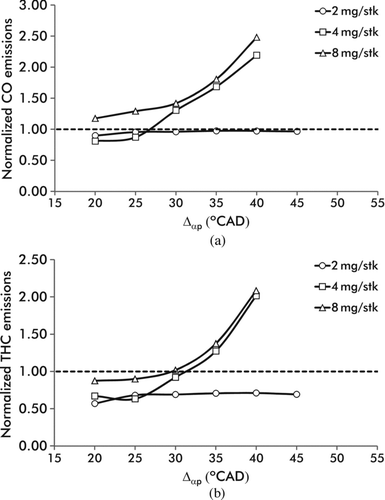
The trend observed for CO and HC emissions regarding the ΔαP parameter and the postinjection quantity is coherent with the previous results obtained for BSFC. As explained previously, for small postinjections the indicated efficiency and BSFC are similar to the reference case, resulting in emission formations that are similar to the single injection case. For large postinjection quantities and large ΔαP values, the degradation of the combustion causes a decrease in indicated efficiency (increase in BFSC) and, therefore, an increase in CO and THC.
In the case of NOX emissions, shows the trend of normalized NOX depending on the ΔαP parameter, specifically for A75 mode and for different postinjection quantities. The highest temperatures are obtained at high load and, therefore, the production of NOX emissions is the highest of the three studied operating modes. It can be observed that for all the postinjection cases tested in this mode, the obtained values of NOX emission are below the reference of the single injection strategy.
FIG. 5 The NOX engine-out emissions for operating mode A75, the obtained values of NOX emissions are below the reference of the single injection strategy.
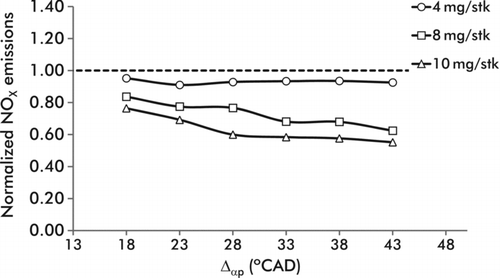
In general, a correlation between NOX formation and the maximum temperature of burnt gases was found in previous publications using various single injection strategies (CitationYu and Shahed 1981; CitationAhmad and Plee 1983; Lida and Watanabe 1990; CitationDesantes et al. 1999). It can also be assumed that the total amount of NOX produced at the end of combustion is the combination of the NOX formed during the combustion of the main injection and the NOX formed during the combustion of the postinjection (CitationArregle et al. 2008). Therefore, the observed reduction in NOX emissions for the postinjection strategies can be explained with the temperature profiles shown in . When postinjections are used (especially large postinjections) less fuel is burnt in the main injection (closer to TDC) and this results in lower temperatures during the combustion in the main injection, and so less NOX is produced during the combustion of the main injection. Later, when the combustion of the postinjection occurs, more NOX will be created, but because of the degradation of the conditions inside the chamber (the temperature and pressure are lower when further from TDC) and the fact that the quantity of fuel injected during postinjection is always less that the main injection, the potential to create NOX is lower. Therefore, the total amount of NOX formed is always lower than the reference case of the single injection, as is seen in .
FIG. 6 Soot engine-out emissions from operating mode C25, for a standard injection strategy (M) and a strategy with a postinjection (M* + P; in this case the gap between the main and the postinjection pulse is modified). The two regions where soot emissions are improved with the postinjection are shaded in the graph.
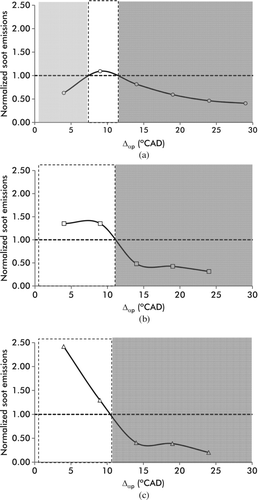
It is known that the use of a postinjection strategy influences the soot oxidation process and/or the soot formation process. There are many studies dealing with postinjection in the literature (Hasse et al. 1999; Chen 2000; CitationBenajes et al. 2001; Bianchi et al. 2001; Hotta et al. 2005; Desantes et al. 2007; CitationArregle et al. 2008). Because of the wide variety of scenarios studied by the different authors, an analysis of the conclusions must be performed very carefully.
Engine-out soot emissions are the result of a complex balance between in-cylinder soot formation and oxidation (CitationHiroyasu and Kadota 1976; CitationTree and Svensson 2007). Soot is formed in the diffusion flame just after the lift-off rich premixed burn region (Dec and Espey 1995). The size and the mass of the soot particles increase when traveling inside the diffusion flame (Dec 1997); and finally the soot particles are partially oxidized in the diffusion flame front (CitationHiroyasu and Kadota 1976). Final soot emissions, therefore, depend on the amount of formed and oxidized soot.
In , different scenarios of soot formation for the C25 operating condition are shown for different postinjection quantities. The horizontal line in this figure represents the soot emission level obtained with an M (main injection) standard injection strategy. The other curves show the normalized soot emissions for each postinjection quantity depending on the ΔαP between the main and the postinjection.
In , two possible regions can be seen where soot emissions are improved with the use of postinjection, one region where the postinjection occurs very close to the main injection (lower ΔαP values) and the other region where the postinjection occurs further away from the main injection.
The condition with close postinjections was described in depth and explored in a previous study (Desantes et al. 2007). In our case, an acceleration of the final stages of combustion takes place and this leads to the following: an earlier EoC (end of angle combustion for 90% of heat release); an increase in indicated efficiency; and a decrease in exhaust gas temperature. The earlier EoC implies that the soot oxidation process takes place earlier, hence at a higher temperature. Consequently, this process will be more efficient and this explains why final soot emissions are lower.
However, the scenario changes completely with far postinjections. Several theories have been proposed by different authors on how postinjection achieves a reduction in the final soot emissions. CitationNehmer and Reitz 1994; Hasse et al. 1999; Bianchi et al. 2001 and Hotta et al. 2005 proposed that the extra energy for mixing introduced in the chamber by the postinjection pulse improves the oxidation process and, therefore, reduces the final soot level. CitationBakenhus and Reitz 1999 and Chen 2000 showed that the high temperature in the chamber prompted by postinjection is responsible for a better oxidation and, consequently, a reduction in final soot emissions. Other researchers state that the final soot emission decreases because the main pulse produces less soot and postinjection does not produce significant additional soot (Han et al. 1996; Desantes et al. 2007; CitationArregle et al. 2008).
Nevertheless, in and c, it is only possible to see a reduction in soot emissions in the far postinjection region. In cases where the postinjection quantity is large, the effect that close postinjection has on the acceleration of the final stages of combustion is less important than the decrease in the temperature of the diffusion flame, which increases the capability to produce more soot (CitationArregle et al. 2008).
Particle Size Distributions
Particle size affects the atmospheric residence time of particles, the optical properties of the particles, the role of particles in atmospheric chemistry, and the health impact of the particles (Kittelson 1999; Lapuerta et al. 2008). Influence of postinjection fuel quantity and Δαp is studied in this work by analyzing its effect on the characteristics of the particle number size distributions. However, it should be noted that the objective of the work is to explore the effect of a postinjection pattern on the particle size distribution and not to carry out an optimizing work for reducing the number of particles.
To obtain accurate values for accumulation particle numbers and geometric mean diameters (GMD), the accumulation mode was extracted from the particle number distribution by fitting the experimental data to a theoretical equation for normalized log-normal distributions (Seinfeld and Pandis 1998):
Where Ni , is the particle number concentration of particle size dpi , N is the total particle number concentration, dpg is the GMD, and σg is the geometric standard deviation. The fitting was achieved by minimizing the mean square error function by means of the Nelder–Mead simplex method. The difference between the experimental data and the fitted size distributions was calculated for the C25 operating mode, for which a direct comparison was possible since the nucleation mode was lower. The deviation in both total particle number and mean size was less than 3%. The case of bimodal size distributions can be described as the combination of two individual distributions by assuming the log-normal size distribution function
Where, x is the ratio of the total number of concentrations of two distributions and dp 1 , dp 2 , σg 1, and σg 2 are the GMDs and geometric standard deviations of each peak.
Diverse studies proposed the limits of the nucleation mode at various diameter values of between 50 and 30 nm (Kittelson 1998; Lapuerta et al. 2008). In this study, the decomposition of the particle number size distribution was: nucleation mode particles from 5.6 to 30 nm; accumulation mode particles from 30 to 560 nm. To calculate total particle number concentration and GMD for each mode Equations (4) and (5) were used:
Particle number size distributions measured with the SMPS under diluted conditions were correctly scaled so that they could be expressed for the raw exhaust gas. In the discussion that follows, the results are described separately for the various steady-state engine conditions used in the experimental study. In –9, particle size distributions corresponding to each operating condition are shown; the influence on the accumulation mode of total particle number and GMD is shown in and 11; and for the variations in the nuclei mode, total particle numbers; and GMDs are shown in and 13.
FIG. 7 Influence of postinjection Δαp (a, b, and c figures) at constant fuel mass postinjected on the particle size distributions. Engine operating conditions A75 (1500 RPM, 75% Load).
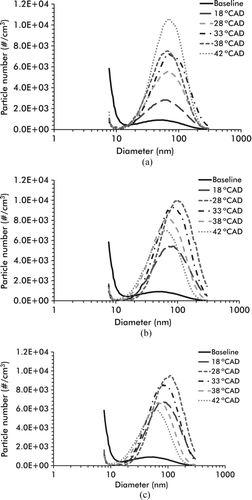
FIG. 8 Influence of postinjection Δαp (a, b, and c figures) at constant fuel mass postinjected on the particle size distributions. Engine operating conditions B50 (2250 RPM, 50% Load).
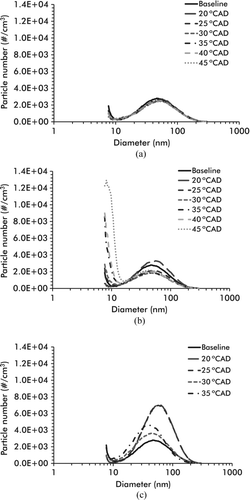
FIG. 9 Influence of postinjection Δαp (a–c) at constant fuel mass postinjected on the particle size distributions. Engine operating conditions C25 (3000 RPM, 25% Load).
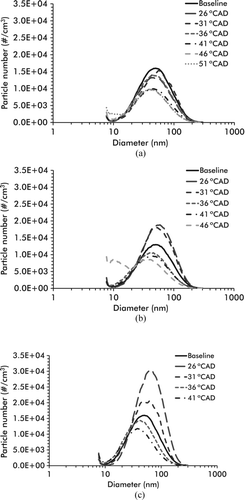
FIG. 10 Effects of postinjection fuel mass and Δαp on accumulation mode particle number at different operating conditions.
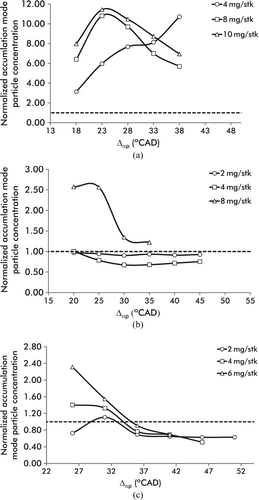
FIG. 11 Effects of postinjection fuel mass and Δαp on accumulation mode particle GMD at different operating conditions.
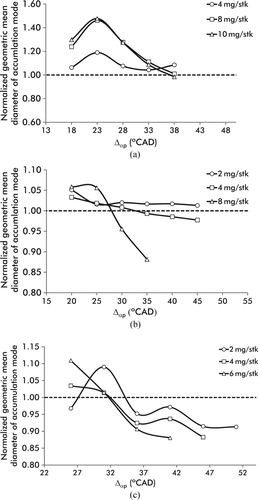
FIG. 12 Effects of postinjection fuel mass and Δαp on nucleation mode particle number at different operating conditions.
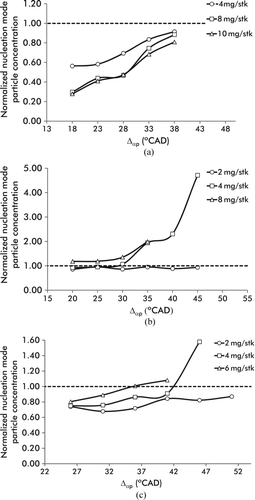
FIG. 13 Effects of postinjection fuel mass and Δαp on nucleation mode particle GMD at different operating conditions.
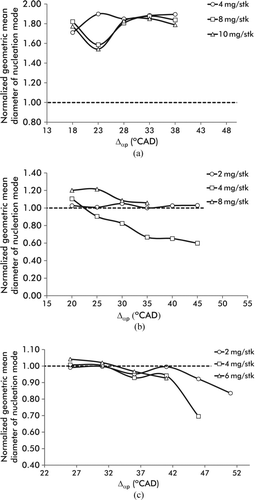
A75 Operating Mode
Particle number size distributions for A75 operating mode are shown in , where plots (a), (b), and (c) show the influence of split injection Δαp at a constant postinjection fuel quantity. The aerosol from these operating conditions consists of a large nucleation mode and very small accumulation mode. Little carbonaceous aerosol is observed due to the low speed conditions (i.e., long residence times) that favor soot oxidation.
shows how the accumulation mode increases with the increase in Δαp, the 18°CAD case shows the lowest increase of number concentration for any fuel amount. For a small quantity of postinjected fuel (4 mg/stk) the increase in Δαp increases the number concentrations in the accumulation mode. The intermediate Δαp 28°CAD, 33°CAD, and 38°CAD results were close to the 42°CAD. In the cases, 8 mg/stk and 10 mg/stk of postinjected fuel, the higher increase in the accumulation mode was shown in the Δαp of 28°CAD. The effects of injection Δαp and fuel quantity (as a percentage of total fuel) on the accumulation mode particle number when compared with the baseline case are shown in the A75 plot in .
The effects of postinjection Δαp and fuel quantity on the accumulation mode particle number, compared with the baseline case, are shown in the A75 plot in . It can be seen from this plot that the particle number increases for fuel quantity 4 mg/stk, while at 8 mg/stk and 10 mg/stk a rise is produced when the Δαp is 38°CAD. Nevertheless, in all cases the increase in the accumulation particle number size distribution is too high compared with the main injection case. This increase in the accumulation mode may be due to a fall in the mean temperature in the cylinder that reduces the soot oxidation process. These effects can be shown in the increase in the accumulation mode GMD (). Moreover, the nucleation mode was lower than the reference case for all cases. This decrease is more pronounced when the postinjected fuel mass increases, and less than great Δαp ().
A relationship was found between the ΔαP and the nucleation mode emissions. The use of postinjection reduces the nucleation mode particle emissions, and this reduction was more pronounced with the use of close postinjection and a large quantity of postinjected fuel. Three phenomena could be responsible for achieving the particle number reduction:
• | Nucleation from supersaturated vapor condensation (homogenous nucleation) diminishes due to the reduction in THC emissions and a reduction in the saturation ratio in the exhaust gases (with the use of close postinjections). | ||||
• | Increase in volatile deposition with a meta-stable equilibrium on the soot due to increases in the soot surface (heterogeneous nucleation). This can be explained by Van der Waals forces. | ||||
• | In some cases, even if nucleation particles are formed, removal by coagulation in the soot mode would be greatly enhanced. |
In the works of Payri et al. 2002, a similar study was performed by analyzing the influence of the postinjection pattern on soot smoke emissions from a heavy-duty diesel engine. A comparison of the trends observed by Payri can be achieved with the results of accumulation mode particles present in this operating mode. These authors found that at operating conditions in which low smoke emissions were measured, the postinjection results were close to or above the baseline conditions. The same occurs for particle numbers in the current experimental results for the A75 operating mode. The reduction achieved in the referred work was greatest with respect to the other operating conditions in which higher levels of soot were emitted. The results of Payri et al. 2002, together with the observations for the A75 operating mode indicate that postinjections are not so efficient at operating conditions in which few particles are emitted. More precisely, in our results, the injection schemes do not provide a particle number reduction with respect to the baseline case.
B50 Operating Mode
shows the particle number size distribution in B50 operating mode. This operating mode presents bimodal particle size distributions without postinjection. In these distributions, adsorption and/or desorption phenomena and heterogonous nucleation are important. In this case, the balance between the nucleation mode and accumulation mode can be disturbed by postinjection.
A slight change in particle size distribution is obtained with postinjection Δαp when a small amount of fuel is postinjected (2 mg/stk). The reduced influence of the small amount of postinjected fuel in the combustion process () does not present significant changes in the particle number size distributions. The small modifications of particle number size distributions under these operating conditions makes it difficult to obtain precise conclusions about how the small amount postinjected fuel affects the particle number size distributions.
For large postinjected masses (4 mg/stk) the accumulation mode was reduced with the increase of ΔαP. Reductions of 33% with respect to the baseline case can be achieved. However, this reduction in the accumulation mode results in an increase in the nucleation mode because of adsorption and/or desorption, and homogenous nucleation is beginning to be replaced by homogenous nucleation as a result of reductions in the available soot surface. The decrease in the accumulation mode was higher with the increase of the ΔαP (). In nucleation mode, the increase in the ΔαP shows a four-fold rise in particle concentration with a maximum ΔαP = 45°CAD when compared with the reference case (). The phenomena of adsorption and/or desorption, and heterogenous nucleation can be seen in and 13, with the reduction of the GMD accumulation mode evidencing the improvements in the balance of soot formation and oxidation. The reduction in the GMD nucleation mode reveals the size of the increase in homogenous nucleation.
In the case of an even greater amount of postinjected fuel mass (8 mg/stk) the particle size distributions cannot be improved due to changes produced in the combustion process. When the postinjected mass is 8 mg/stk, some 36% of the total injected fuel mass is postinjected. In these cases, the conditions of temperature and pressure in the combustion chamber change drastically—revealing an increase in particle concentrations, as well as in the specific brake fuel consumption and emissions of CO and HC.
TABLE 4 Coefficients for relative mean difference variations for particle number calculated between measurement from the study of the influence of start of postinjection and fuel mass quantity
C25 Operating Mode
This operating mode presents a mono-modal particle size distribution in the accumulation zone. In this case, particle size distribution is determined by in-cylinder soot formation and oxidation; however, it should be kept in mind that the oxidation rate of soot depends on the amount of soot formed and the activation temperatures. These parameters may, therefore, be affected by the use of postinjection to decrease the soot oxidation rate, causing the resulting soot formation and oxidation balance to be higher than the reference case (CitationHiroyasu and Kadota 1976).
Small amounts of postinjected mass fuel (2 mg/stk) show improvements in the particle size distribution in nucleation mode—and especially in accumulation mode. A slight decrease in the mean diameter can be observed ( and 13). Nevertheless in the case of 2 mg/stk with an intermediate ΔαP = 31°CAD, an increase in particle number size distributions was observed in comparison with the reference case—especially in the accumulation mode particles. In some preceding studies (Desantes et al. 2007; CitationArregle et al. 2008), two interesting phenomena were observed that were related to the combustion process and the evolution of soot emissions (mostly regarding particles in accumulation mode). The first phenomenon is acceleration in the final stages of combustion when close postinjection is used; the second phenomenon is an apparent disconnection between the main and postcombustion (when a far postinjection is used) which is termed split flame. Acceleration of the final stages of combustion implies that soot formation was high—but that the soot oxidation process took place earlier, and thus at a higher temperature. Consequently, the process is more efficient and this explains why final soot emissions are lower. This reduction can be observed in and 9a in the case of ΔαP = 26°CAD. With the use of far injections (ΔαP ≥ 36°CAD), the scenario differs completely from the previous scenario. In this case, the final level of soot decreases because the main injection produces less soot and the postinjection does not produce significant additional soot (CitationArregle et al. 2008). The case of ΔαP = 31°CAD is a middle point between the phenomenon of increased soot formation typical of close postinjection and lower temperatures to oxidize the soot that are characteristic of far postinjection.
It can be noted that the reduction in the particle number in accumulation mode () and the reduction of soot emissions () does not present the same value—but shows the same tendency. Differences can be noticed because the particle number for accumulation mode is mostly soot, while also containing particles from heterogeneous nucleation.
In , it can also be observed that the fuel mass of the postinjection with the use of high ΔαP ≥ 36°CAD does decrease the particle number size distributions. This can be explained as follows: the use of a large amount of postinjected fuel with high ΔαP reduces the quantity of the fuel in the diffusion flame combustion. Therefore, soot cannot be produced in this phase. The reduction produced by the disrepair of the combustion process is penalized with an increase in particle number concentrations in nucleation mode. This increase is caused by the large amount of THC in the engine exhaust gases and the super saturation of the volatile compounds taking place in the homogenous nucleation. When the postinjections are close to the main injection ΔαP ≤ 31°CAD, the diffusion flame cannot be established and the soot precursors generated in the fuel-rich premixed combustion are not oxidized in the diffusion flame. Significant numbers of final accumulation mode particles are also presented in the particle number size distributions.
To determine if the changes observed in the particle number size distributions measured in this study represent significant variations, relative mean variations were calculated for each group for an independent evaluation of postinjected fuel mass and the start of postinjection for all the operating conditions. The coefficients of variability (calculated on an accumulation and nuclei mode particle number basis) are listed in . From these results it can be seen that the change in the mass fuel quantity affects the number of particles in the accumulation mode more than the nuclei mode; the ΔαP also affects the number of accumulation mode particles more than the nuclei mode in A75 operating mode. In the B50 operation mode, the accumulation mode was more affected by the use of large amounts of postinjected fuel and the nucleation mode was affected by the use of far injections. For the case of C25 operating mode, both accumulation and nuclei mode were affected by the use of small amounts of postinjected fuel.
CONCLUSIONS
The influence of postinjection fuel quantity and ΔαP on the particle size distributions from a light-duty diesel engine was investigated under three steady-state engine conditions. As a general trend, when postinjection is applied, the quantity of postinjected fuel mass affects the accumulation mode particle number and GMD more than the accumulation mode for low speed conditions with high load (A75 operating mode). In conditions of medium and high speed with medium load and low load respectively (B50 and C25 operating modes), the ΔαP influences accumulation mode. Slight changes in the nuclei mode for all operating modes were observed with postinjected fuel mass. Injection ΔαP also affects the nuclei mode particles–but less so.
A reduction in the particle number size distributions in comparison with the baseline case of single injection was observed when postinjections were applied for B50 with the use of small amounts of postinjected fuel mass and under the C25 operating condition with the use of small amounts of postinjected fuel with a gap between the main injection and the postinjection of 36°CAD and 51°CAD. Falls in efficiency was dependent on the postinjection scheme and operating conditions. The maximum reduction in the accumulation mode was found in the C25 operating mode with a ΔαP = 36°CAD, regardless of the postinjected fuel mass. For the accumulation mode, the maximum reduction was found in the A75 operating condition with a ΔαP = 18°CAD.
Conversely, for the case of low accumulation mode particle number (A75), an increase in the particle number was produced when postinjection was applied in any injection scheme.
A certain relation was found between the combustion process and GMD with the balance of formation/oxidation of soot.
Finally, the results of this research provide some quantitative criteria to optimize postinjection strategies, and although this study is focused on postinjection particle number emission size distribution, the study can be considered as an initial approach to a more general analysis of overall particle emission numbers. The effects on this process of other parameters (i.e., exhaust gas recirculation and biodiesel) will be explored in future research.
DEFINITIONS/ABREVIATIONS
ΔαP | = |
Gap between the main injection and postinjection |
aTDC | = |
After top dead center |
BSCF | = |
Brake specific fuel consumption |
CAD | = |
Crank angle degree |
EoC | = |
End of combustion |
FSN | = |
Filter smoke number |
GMD | = |
Geometric mean diameter |
HCLD | = |
Heated chemiluminescence detector |
HFID | = |
Heated flame ionization detector |
HRL | = |
Heat release law |
HRR | = |
Heat release rate |
HSDI | = |
High speed direct injection |
M | = |
Main injection |
M* | = |
Shortened main injection |
NDIR | = |
Nondispersive infrared |
P | = |
Postinjection |
SMPS | = |
Scanning mobility particle sizer |
SoI | = |
Start of injection |
stk | = |
Stroke |
TDC | = |
Top dead center |
ULSD | = |
Ultra-low sulfur diesel |
Acknowledgments
We would like to thank the R&D&I Linguistic Assistance Office, Universidad Politécnica de Valencia, Spain, for granting financial support for the linguistic revision of this paper.
REFERENCES
- Ahmad , T. and Plee , S. L. 1983 . Application of Flame Temperature Correlations to Emissions from Direct-Injection Diesel Engine , Warrendale , PA : SAE . SAE Technical Paper, 831734
- Anrigo , P. Evolution of the Diesel Engines; From the Kaizen to the Innovate Concepts, the Various Roads to Environment-Friendly Progress . 4th Conference on Thermo- and Fluid Dynamic processes in Diesel engines, THIESEL, Valencia, Spain .
- Arregle , J. , Pastor , J. V. , López , J. J. and García , A. 2008 . Insights on Post-Injection-Associated Soot Emissions in Direct Injection Diesel Engine . Combust. Flame , 157 : 448 – 461 .
- Bakenhus , M. and Reitz , R. D. 1999 . Two-Color Combustion Visualization of Single and Split Injections in a Single-Cylinder Heavy-Duty D.I. Diesel Engine Using an Endoscope-Based Imaging System , Warrendale , PA : SAE . SAE Technical Paper, 1999-01-1112
- Baumgard , K. J. and Johnson , J. H. 1996 . The Effect of Fuel and Engines Design on Diesel Exhaust Particles Size Distributions , Warrendale , PA : SAE . SAE Technical paper, 960131
- Benajes , J. , Molina , S. and García , J. M. 2001 . Influence of Pre- and Post-Injection on the Performance and Pollutant Emissions in a HD Diesel Engine , Warrendale , PA : SAE . SAE Technical paper, 2001-01-0526
- Bertola , A. , Schubiger , R. , Kasper , A. , Matter , U. , Forss , A. M. , Mohr , M. , Boulouchos , K. and Lutz , T. 2001 . Characterization of Diesel Particulate Emissions in Heavy Duty DI Diesel Engines with Common Rail Fuel Injection: Influence of Injection Parameters and Fuel Composition , Warrendale , PA : SAE . SAE Technical paper, 2001-01-3573
- Bianchi , G. M. , Pelloni , P. , Corcione , F. E. and Luppino , F. 2001 . Numerical Analysis of Passenger Car HSDI Diesel Engines with the 2nd Generation of Common Rail Injection Systems: The Effect of Multiple Injections on Emissions , Warrendale , PA : SAE . SAE Technical Paper, 2001-01-1068
- Chen , S. K. 2000 . Simultaneous Reduction of NOx and Particulate Emissions by Using Multiple Injections in Small Diesel Engine , Warrendale , PA : SAE . SAE Technical Paper, 2000–01-3084
- Dec , J. E. and Espey , C. 1995 . Ignition and Early Soot Formation in a DI Diesel Engine Using Multiple 2-D Imaging Diagnostics , Warrendale , PA : SAE . SAE Technical Paper, 950456
- Dec , J. E. 1997 . A Conceptual Model of DI Diesel Combustion Based on Laser-Sheet Imaging , Warrendale , PA : SAE . SAE Technical Paper, 970873
- Desantes , J. M. , Arregle , J. , López , J. J. and García , A. 2007 . A Compreshive Study of Diesel Combustion and Emissions with Post Injection , Warrendale , PA : SAE . SAE Technical Paper, 2007-01-0195
- Desantes , J. M. , Bermúdez , V. , Pastor , J. V. and Fuentes , E. 2006 . Investigation of the Influence of Post-Injection on Diesel Exhaust Aerosol Particle Size Distributions . Aerosol Sci. Tech. , 40 : 80 – 96 .
- Desantes , J. M. , Pastor , J. V. and Molina , S. 1999 . Analysis of the Combustion Process in a Heavy Duty D.I. Diesel Engine Through In-Cylinder Visualitation . ASME Spring Technical Conference , 2 : 105 – 113 .
- García , J. , Gil , A. and Estellés , V. 2000 . Theoretical and Experimental Model for the Burnt Products of Combustion Temperature Calculating on Direct Injection Diesel Engines. (in Spanish) . An. de Ing. Mecánica , 13 ( 2 ) : 2023 – 2028 .
- Guerrassi , N. and Dupraz , P. 1998 . A Common Rail Injection System for High Speed Direct Injection Diesel Engines , Warrendale , PA : SAE . SAE Technical paper, 980803
- Han , Z. , Uludogan , A. N. , Hampson , G. J. and Reitz , R. D. 1996 . Mechanism of Soot and NOx Emissions Reduction Using Multiple-Injection in a Diesel Engine , Warrendale , PA : SAE . SAE Technical Paper, 960633
- Hasse , C. , Barths , H. and Peters , N. 1999 . Modeling the Effect of Split Injections in Diesel Engines using Representative Interactive Flamelets , Warrendale , PA : SAE . SAE Technical Paper, 1999-01-3547
- Heywood , J. 1988 . Internal Combustion Engine Fundamentals , Mc Graw-Hill, New York .
- Hiroyasu , H. and Kadota , T. 1976 . Models for Combustion and Formation of Nitric Oxide and Soot in Direct Injection Diesel Engines , Warrendale , PA : SAE . SAE Technical Paper, 760129
- Hotta , Y. , Inayoshi , M. , Nakakita , K. , Fujiwara , K. and Sakata , I. 2005 . Achieving Lower Exhaust Emissions and Better Performance in an HSDI Diesel Engine with Multiple Injection , Warrendale , PA : SAE . SAE Technical Paper, 2005-01-0928
- Ishida , M. , Ueki , H. , Matsumura , N. , Yamaguchi , M. and Luo , G. F. 1996 . Diesel Combustion Analysis Based on Two Zone Model (comparison between model analysis and experiment) . JSME Int. J., Ser. B , 39 : 185 – 192 .
- Kittelson , D. B. 1998 . Engines and Nanoparticles: A Review . J. Aerosol Sci. , 29(5–6) : 575 – 588 .
- Kittelson , D. B. and Abdul-Khalek , I. S. 1999 . Tuscon , AZ Formation of Nanoparticles during Exhaust Dilution Presented at EFI Members Conferences: Fuels, Lubricants, Engines, and Emissions
- Kreso , A. M. , Johnson , J. H. , Gratz , L. D. , Bagley , S. T. and Leddy , D. G . 1998 . A Study of the Effects of Exhaust Gas Recirculation on Heavy-Duty Diesel Engine Emissions , Warrendale , PA : SAE . SAE Technical paper, 981422
- Lancaster , D. R. , Krieger , R. B. and Lienesch , J. H. 1975 . Measurement and Analysis of Engine Pressure Data , Warrendale , PA : SAE . SAE Technical paper, 750026
- Lapuerta , M. , Armas , O. and Bermudez , V. 2000 . Sensitivity of Diesel Engine Thermodynamic Cycle Calculation to Measurement Errors and Estimated Parameters . Appl. Therm. Eng. , 20 : 843 – 861 .
- Lapuerta , M. , Armas , O. and Hernandez , J. J. 1999 . Diagnostic of DI Diesel Combustion in-Cylinder Pressure Signal by Estimation of Mean Thermodynamic Properties of the Gas . Appl. Therm. Eng. , 19 : 555 – 519 .
- Lapuerta , M. , Armas , O. and Rodrguez-Fern´ndez , J. 2008 . Effect of Biodiesel Fuels on Diesel Engine Emission . Prog. Energ. Combust. Sci. , 34 : 198 – 223 .
- Lida , N. and Watanabe , J. 1990 . Surrounding Gas Conditions Effects on NOx And Particulates . Proceedings International Symposium COMODIA , 90 : 625 – 632 .
- Nehmer , D. and Reitz , R. 1994 . Measurement of the Effect of Injection Rate and Split Injection on Diesel Engine Soot and NOx Emissions , Warrendale , PA : SAE . SAE Technical Paper, 940668
- Payri , F. , Benajes , J. , Pastor , J. V. and Molina , S. 2002 . Influence of the Post-Injection Pattern on Performance, Soot and NOx Emissions in a HD Diesel Engine , Warrendale , PA : SAE . SAE Technical Paper
- Payri , R. , Salvador , J. , Gimeno , J. and Bracho , G. 2008 . A New Methodology for Correcting the Signal Cumulative Phenomenon on Injection Rate Measurements . Exp. Techniques , 32 : 46 – 49 .
- Pickett , L. M. and Siebers , D. L. 2004 . Soot in Diesel Fuel Jets: Effects of Ambient Temperature, Ambient Density, and Injection Pressure . Combust. Flame , 138 : 114 – 135 .
- Plint , M. and Martyr , A. 1999 . Engine Testing Theory and Practice. , Oxford : Butterworth-Heinemann .
- Seinfeld , J. H. and Pandis , S. N. 1998 . Atmospheric Chemistry and Physics: from Air Pollution to Climate Change Wiley, New York
- Suresh , A. and Johnson , J. H. 2001 . A Study of the Dilution Effects on Particle Size Measurement from Heavy-Duty Diesel Engine with EGR , Warrendale , PA : SAE . SAE Technical Paper, 2001-01-0220
- Tow , T. C. , Pierpont , D. A. and Reitz , R. D. 1994 . Reducing Particulate and NOx Emission by Using Multiple Injections in a Heavy Duty Diesel Engine , Warrendale , PA : SAE . SAE Technical Paper, 940897
- Tree , D. R and Svensson , K. I. 2007 . Soot Processes in Compression Ignition Engines . Prog. Energ. Combust. Sci. , 22 : 272 – 309 .
- Yu , R. C. and Shahed , S. M. 1981 . Effects of Injection Timing and Exhaust Gas Recirculation on Emissions from a DI Diesel Engine , Warrendale , PA : SAE . SAE Technical Paper, 811234