Abstract
High-quality in situ observations of aerosol particle optical properties, namely extinction, scattering, and absorption, provide important information needed to constrain the role of aerosols in the climate system. This paper outlines the design and performance of an aircraft instrument utilizing cavity ringdown spectroscopy for the measurement of aerosol extinction. The 8-channel cavity ringdown spectrometer measures extinction at multiple wavelengths (405, 532, and 662 nm) and at multiple relative humidities (e.g., 10%, 70%, and 95%). Key performance characteristics include a 1-s detection limit better than 0.1 Mm−1, accuracy of <2% for dry aerosol measurements, and a 1-s precision better than 40% for extinction levels of >10 Mm−1. Laboratory and field data demonstrate that the 1-s precision is limited by the statistics of aerosol particles in the laser beam rather than the precision of the extinction measurement per se. The measurement precision improves with averaging to 5% at 60 s for extinction levels of >10 Mm−1. Field data collected during a recent airborne campaign in California, which involved eighteen research flights during May and June 2010, are used to demonstrate the in-flight performance of new instrument.
1. INTRODUCTION
Atmospheric aerosol particles are recognized as important forcing agents of climate (Solomon et al. Citation2007). They modify the earth's energy budget both directly, through absorption and scattering of solar radiation, and indirectly, through the modification of cloud properties. This work concerns the direct aerosol forcing, which has been the focus of significant research effort in recent years, yet remains highly uncertain and a significant constraint on the evaluation of climate sensitivity (Schwartz et al. Citation2010). Continued work to improve understanding in this area is underway, encompassing a range of complementary approaches, including long-term monitoring of aerosol properties from remote sensing platforms (Dubovik et al. Citation2002; Anderson et al. Citation2005), improved representation of aerosols in large-scale models (Kinne et al. Citation2006; Textor et al. Citation2006), and, the focus of this work, detailed in situ characterization of optical properties of aerosol particles (Quinn et al. Citation2006; Massoli et al. Citation2009a).
In situ characterization of aerosol optical properties provides a uniquely detailed means to evaluate the fundamental physiochemical aerosol characteristics controlling optical behavior. It allows the determination of key climate-relevant parameters such as single scattering albedo ω (the ratio of aerosol light scattering to light extinction) with high accuracy as a function of variables such as wavelength and relative humidity. This detailed understanding is important for the development of realistic model parameterizations, but is gained at the expense of measurement spatial coverage. This limitation is remedied to some extent by utilizing mobile platforms, such as aircraft.
Conventional techniques for the measurement of aerosol scattering and absorption coefficients, namely integrating nephelometry (Heintzenberg and Charlson Citation1996; Anderson et al. Citation2003) and filter-based photometry (Bond et al. Citation1999) respectively, have been widely used aboard research aircraft (Clarke and Kapustin Citation2010) to sample varied aerosol types, including biomass burning (Haywood et al. Citation2003b; Clarke et al. Citation2007), dust (Haywood et al. Citation2003a; Howell et al. Citation2006), marine (Hegg et al. Citation2002; Marshall et al. Citation2007), and pollution (Mallet et al. Citation2005; Morgan et al. Citation2010). Despite their considerable value, these measurement techniques no longer represent the most accurate means with which to probe these key climate-relevant properties (Massoli et al. Citation2009b). New instrumentation for the measurement of both aerosol absorption, through photoacoustic spectroscopy, PAS (Arnott et al. Citation1999; Lack et al. Citation2006), and extinction, through cavity ringdown spectroscopy, CRDS (Strawa et al. Citation2003; Moosmuller et al. Citation2005; Baynard et al. Citation2007; Radney et al. Citation2009), yield higher accuracies than conventional techniques (Lack et al. Citation2006; Baynard et al. Citation2007). In addition, the combination of CRDS and PAS has yielded significantly lower uncertainties (up to a factor ∼4) in determined aerosol intensive properties such as single scattering albedo (Baynard et al. Citation2007). Given that uncertainties in aerosol optical property measurements contribute significantly to current uncertainties in climate prediction (Schwartz et al. Citation2010), researchers have increasingly used these techniques to attain improved surface-based measurements (Lack et al. Citation2008; Nakayama et al. Citation2010); however, use on aircraft platforms has thus far been limited (Arnott et al. Citation2006; Strawa et al. Citation2006).
This work outlines the design and performance of a comprehensive new instrument package for the measurement of aerosol optical properties from aircraft. It encompasses an eight-channel cavity ringdown spectrometer for the measurement of multi-wavelength extinction and its relative humidity dependence (this paper), and a five-channel photoacoustic spectrometer for the measurement of multi-wavelength aerosol absorption and coating-derived absorption enhancements (companion paper, Lack et al. Aircraft Instrument for Comprehensive Characterization of Aerosol Optical Properties, Part 2: Black and Brown Carbon Absorption and Absorption Enhancement Measured using Photo-Acoustic Spectroscopy, Aerosol Sci. Technol., in review). This paper is divided into three sections. The first section provides a comprehensive description of the CRDS instrument design, encompassing its optical setup, data acquisition (DAQ) system, and software. The second section demonstrates the operational performance of the instrument and addresses topics including detection limits and measurement uncertainty using data from both laboratory and field environments. The final section presents highlights of airborne field data recorded by the instrument aboard the National Oceanic and Atmospheric Administration (NOAA) WP-3D aircraft during its first deployment in May/June 2010 as part of the California air quality and climate nexus research project CalNex.
2. INSTRUMENT
Cavity ringdown spectroscopy (O’Keefe and Deacon Citation1988) is a well-established method for performing highly sensitive extinction measurements. It has been used extensively for both gas phase and aerosol measurements of atmospherically relevant constituents and processes (Brown Citation2003). Of particular relevance, researchers have established the technique as a means with which to perform field measurements of aerosol extinction (Strawa et al. Citation2003; Moosmuller et al. Citation2005; Baynard et al. Citation2007; Radney et al. Citation2009; Nakayama et al. Citation2010). The development of the current instrument draws strongly on this prior work and our intention here is not to re-evaluate the use of CRDS for aerosol measurements, but rather to provide confidence in the high-quality extinction measurements that the new instrument can achieve while operating from an airborne platform. The reader is referred to references provided in section one for discussion regarding the benefits of CRDS aerosol extinction measurements compared to, for example, scattering measurements performed using nephelometry.
A number of comprehensive review papers (e.g., Berden et al. Citation2000; Mazurenka et al. Citation2005) serve as good references for the underlying principles of CRDS and only a very brief description is provided here. In its simplest form, cavity ringdown spectroscopy involves injecting a short laser pulse into an optically stable cavity formed by two highly reflective plano-concave mirrors. For a correctly aligned system, the intensity of the circulating pulse decays exponentially with time, principally due to transmission losses through cavity mirrors and absorption/scattering interactions within the cavity. The decay rate of light in the cavity, which is characterized by its 1/e folding time (termed the ringdown time, τ), is determined both with (τ) and without (τ 0) the absorbing/scattering species of interest present. Subsequently, the extinction coefficient α is calculated directly from
A number of variants of CRDS have been developed, including those that adopt continuous wave diode lasers in place of the more typically used pulsed laser systems (Mazurenka et al. Citation2005). This approach is attractive for use in field instruments as diode lasers are readily available in compact and inexpensive units at a wide range of wavelengths. However, it also brings a number of complications not encountered for pulsed systems. For example, the intrinsic line widths of single mode diode lasers are typically narrower (c.a. 1–10 MHz) than the free spectral range of optical cavities used (c.a. 150 MHz). Thus overlap of the laser frequency with that of a cavity mode is not guaranteed and must be intentionally induced in order to couple light into the cavity. This is often achieved by either scanning the cavity length through at least one free spectral range by mounting one mirror on a piezoelectric stage (Romanini et al. Citation1997), or by sweeping the laser frequency (He and Orr Citation2005). Though effective, these approaches add significant complexity, making them less attractive options for field applications, especially those involving more than one cavity. Recently, Fuchs et al., Citation(2009) showed that multi-mode diode lasers could be passively coupled into optical cavities and used for high-quality ringdown measurements. This result is rather surprising, as the broad bandwidth emission of typical multi-mode diode lasers (c.a. 0.5–1 nm) excites a multitude of cavity modes, which one would expect to lead to the multi-exponential ringdown events that hinder quantitative analysis (Zalicki and Zare Citation1995). Nevertheless, single exponential decays from cavities pumped with multi-mode continuous wave diode lasers have been demonstrated, indicating that such effects do not contribute significantly. As described in the following section, the new aerosol CRDS instrument adopts schemes employing both pulsed laser and multi-mode diode laser excitation.
2.1. Optical Hardware
The CRDS instrument comprises 8 separate measurement cells: three measuring dry (relative humidity <10%) aerosol extinction at wavelengths of 405, 532, and 662 nm, two measuring aerosol extinction at 532 nm and elevated relative humidity, and remaining three measuring the contribution of gas phase extinction at each wavelength.
All optical components are located on an aluminium breadboard measuring 56 × 63 × 3.8 cm. Eight identical optical cells are arranged adjacently, each constructed from two custom-built stainless steel mirror mounts joined by 1-cm ID aluminium tubing. The total mirror-to-mirror length is 48 cm for each cell. Aerosol samples enter the cells through 1-cm ID stainless steel tubes brazed onto the mirror mount assemblies at 45° angles. The sample inlet to outlet distance is 39.7 cm, yielding an RL correction factor (see Equation 1) of 1.21. We note that determination of RL from physical dimensions is appropriate in this system as the flow is laminar (Reynolds number ∼140), and thus particles are expected to closely follow stream lines within the cells with negligible penetration into the volumes beyond the inlet/outlet ports. A port is located adjacent to each mirror to enable a small purge flow (typically 5–10 cm3/min) of filtered zero air to be injected over the mirror surface to prevent contamination during sampling. The optical axis height is minimized in order to optimize the stability of optical components and lies at 3.8 cm above the breadboard surface.
The instrument uses three separate laser sources centered at 405.5, 532.1, and 662.1 nm. These wavelengths will from hereon be referred to as 405, 532, and 662 nm for convenience. The four 532-nm channels are simultaneously pumped by a single, compact, frequency-doubled diode-pumped Nd:YAG laser (QL532-500, CrystaLaser, Reno, NV, USA) emitting 10–30-ns pulses with 100 μJ/pulse at 1-kHz repetition rate. The laser has a manufacturer-specified spectral bandwidth of 0.1 nm full width at half maximum (fwhm). It also incorporates an internal optical isolator to protect it from damage caused by back-reflected radiation. The 662-nm and 405-nm channels are pumped by two multi-mode diode lasers (Power Technology, Little Rock, AR, USA), each of which emit circular beams with approximately 45-mW average power when driven at 1 kHz with a 50% duty cycle. The bandwidths of 662-nm and 405-nm lasers, measured using a grating spectrometer, are 0.8 nm and 0.7 nm fwhm, respectively. The diode lasers have fall times of less than 10 ns, making it possible to initiate ringdown events without the need for fast external optical switching devices. In order to protect the lasers from back-reflected radiation each is optically isolated using the combination of a Glan-Laser calcite polarizor and a ¼ wave plate. Partially transmitting beam splitters are used to direct the laser intensity approximately equally to appropriate cells.
The cavities are constructed from high-reflectivity mirrors (ATFilms, Boulder, CO, USA) manufactured for optimal reflectivity at the relevant laser wavelength. The laser radiation is coupled directly into the optical cells, with no effort to match to particular spatial cavity modes. Directly behind each output mirror, a fiber collimator collects light transmitted by the cavity and focuses it into a fiber optic patch cable. The optical fibers transmit light to an external bank of 8 amplified photomultiplier tubes (HC120, Hamamatsu, Hamamatsu City, Shizuoka Pref., Japan), each of which is protected from stray light using a narrow band interference filter centered at the laser wavelength.
No effort was made to vibration isolate the CRDS optical breadboard from the instrumentation rack for flight operation. Instead, the whole CRDS/PAS rack, which measures 63 × 94 × 111 cm, was vibration isolated from the aircraft using six heavy-duty wire rope isolators (Enidine, Orchard Park, New York, USA). This provided sufficient vibration isolation for operation on an aircraft platform.
2.2. Flow System
A schematic of the instrument's flow system is shown in . On the NOAA WP-3D research aircraft, all fuselage-mounted aerosol instruments sample from a common low turbulence inlet (LTI; Wilson et al. Citation2004). The CRDS system extracts a sample from this common line at 3 litres per minute (LPM). The sample flow passes through a computer-controlled ball valve assembly (MDM–000DT–5, Hanbay, Beaconsfield, PQ, Canada) in which the sample may be periodically diverted through a low-pressure drop aerosol filter (DIF-LK50, United Filtration Systems, Sterling Heights, MI, USA) for the acquisition of τ 0 background measurements. During field operation, τ 0 measurements were typically acquired every 300 s. A multi-tube nafion dryer (PD-100T, Perma Pure, Toms River, NJ, USA, 30.5-cm length, stainless steel housing) dried the sample air to dew points of less than 263 K.
The 8 ringdown cells are organized into the following three groups: elevated humidity channels (2 × 532 nm), dry aerosol measurement channels (532, 662, and 405 nm), and gas phase reference channels (532, 662, and 405 nm). The cells within each group are connected in series via short lengths of 4.5-mm ID aluminium tubing. Each group pulls a total flow of 1 LPM, yielding approximate residence time of 2 s within each cell.
Samples entering the elevated relative humidity (RH) channel flow path are humidified using a single tube nafion humidifier (Perma Pure MH-050, 30.5-cm length, stainless steel housing) that is filled with de-ionized water. The water is circulated through a closed loop encompassing the humidifer, a reservoir, and a micropump. Circulating the water, as opposed to filling the humidifier with stagnant water, prevents it from cooling by evaporative latent heat release. In turn, this enables high RH (typically >90%) to be maintained in the first detection cell without heating the humidifier, which if not done carefully could lead to undesirable condensation of water downstream within the cells. Air exiting the first measurement cell is heated to reduce the RH using a 50-cm length of 4.5-mm ID stainless steel tubing wrapped in heating tape. The residence time within this heater is of the order of 0.5 s, approximately twice that required for the air temperature to reach equilibrium. The second measurement cell is operated at the same elevated temperature. For typical ambient conditions (25–35°C), approximately 5–6°C of heating is required to reduce the RH from 95 to 70%. Under operating conditions in the NOAA WP-3D, where the cabin temperatures can occasionally exceed 35°C, the heater temperature is limited to 40°C in order to minimize potential for loss of aerosol mass through evaporation of semi-volatile components (Huffman et al. Citation2009).
Samples entering the gas reference flow path pass through an aerosol filter (United Filtration Systems DIF-BN40), thus enabling quantification of the contribution of gaseous absorption to the total extinction measured in the aerosol channels. Real-time measurement of gas phase absorption is particularly important during flight, where rapid variability in sampled air masses renders simple interpolation between periodic background checks ineffective. This issue is examined in further detail in Section 3.
A range of housekeeping data is collected for each group of cells. Pressure is measured using a high-precision pressure transducer (PPT0015, Honeywell, Morristown, NJ, USA) and RH is measured using a thermo-hygrometer (HMP50, Vaisala, Vantaa, Finland), which is calibrated using saturated salt solutions. In addition, each of the elevated RH cells is equipped with two high-precision thermistors (Omega Engineering, Stamford, CT, USA), which are used in conjunction with the hygrometer measurements to determine the individual cell RH.
2.3. Particle Losses
Particle losses in the CRDS system can be divided into two categories: those occurring in and between the optical cells, which affect measurements of both aerosol extensive properties (e.g., total extinction) and intensive properties (e.g., wavelength or RH dependence of extinction), and those occurring in the dryer, which generally affect only extensive property measurements. Both are examined below.
Particle losses in the CRDS cells arising from gravitational settling and inertial deposition were evaluated using the Max Planck Institute Particle Loss Calculator (von der Weiden et al. Citation2009). For a 1-LPM flow rate, estimated loss within each cell is <1% for sub-1-μm particles, ∼4% for 2-μm particles, and ∼20% for 5-μm particles. Loss in the sections of tubing connecting cells is <0.25% for sub-1-μm particles, ∼0.75% for 2-μm particles, and ∼5% for 5-μm particles. Thus, in its current configuration the instrument is best used in conjunction with an upstream 1 to 2-μm impactor to primarily monitor accumulation mode aerosol. The negligible loss predicted for sub-1-μm aerosol was confirmed experimentally. Extinction measurements of size selected sub-1-μm ammonium sulphate by two sequential CRDS cells showed losses of <1% for both dry operation and operation at ∼92% RH.
Particle losses through the aerosol dryer were evaluated under laboratory conditions. Extinction by size-selected ammonium sulphate was measured by two independent ringdown cells positioned before and after the dryer. Total losses through the dryer ranged from 5–10% for all aerosol diameters below 2 μm; larger particle sizes were not tested.
We note that the dominant loss mechanism for larger particles in the current system is gravitational settling. By increasing the flow rate to 10 LPM, total cell losses would decrease to <1% for sub-3-μm particles, ∼3% for 5-μm particles, and ∼9% for 8-μm particles. At higher flow rates, the measurement cells should be operated in parallel rather than series, as inertial losses in the inter-cell connection tubing would be significant for particles with diameters greater than 1-μm. Furthermore, higher flow rates would adversely affect the performance of humidification system and therefore the system would need modification. Nevertheless, in future these relatively minor changes in setup could be implemented in order to achieve quantitative sampling of coarse mode particles.
2.4. Data Acquisition (DAQ) and Analysis
The DAQ system is built around a compact four-slot PXI chassis (PXI-1031 DC, National Instruments, Austin, TX, USA) housing three DAQ cards and a Pentium-based quad core controller (PXI-8110, National Instruments). Cavity ringdown decay traces recorded by each of the 8 photomultiplier tubes are digitized by a high-speed 8-channel analog input card (PXI-6133, National Instruments). Each channel samples coherently at a rate of 2.5 MS/s, providing a temporal resolution of 0.4 μs. A multifunction card (PXI-6225, National instruments) outputs digital signals controlling modulation of the diode and YAG lasers. Laser timing and data acquisition are controlled through software written in the LabVIEW programing environment. The DAQ program also performs real-time analysis of the ringdown data. Individual ringdown traces are analyzed using a high-speed exponential fitting routine (Everest and Atkinson Citation2008). At typical laser repetition rates of 1 kHz, 1000 decay traces are acquired and analyzed per channel per second with no co-adding. For all 8 instrument channels this corresponds to the analysis of 8000 traces per second. The retrieved ringdown times (τ and τ 0) are typically averaged for each cell and logged at 1 s.
Extinction is calculated from the ringdown time constants as follows: (i) τ and τ 0 are corrected for Rayleigh scattering to common conditions of 1000 mb and 273.15 K using Equation (1) together with known Rayleigh scattering cross sections for air (Bucholtz Citation1995). This procedure is necessary as during flight τ and τ 0 are often measured at different pressures (altitudes) and are therefore subject to different levels of Rayleigh scattering. Without correction the measured extinction would include a contribution from the difference in Rayleigh scattering encountered at the two pressure levels; (ii) extinction is calculated via Equation (1); (iii) corrections are applied to account for dilution by the mirror purge flows; and (iv) measured gaseous absorption is subtracted from each of the five aerosol measurement channels to yield the aerosol extinction. All of these calculations are performed in real time in the software.
Finally, we note that the CRDS analysis is best performed by the averaging of time constants retrieved from fits of individual traces, rather than co-adding traces before exponential fitting (Mazurenka et al. Citation2005). The reason for this is that co-adding traces with different decay rates can lead to multi-exponential functions, for which analysis based upon a single exponential model is not appropriate. Although the analysis of individual traces is adopted here, and will always represent the most rigorous approach, in practice we found that field data analyzed by co-adding 1000 traces yielded ringdown times that differed from those analyzed on a single-shot basis by less than 1%, even under high aerosol loadings.
3. OPERATIONAL PERFORMANCE AND VALIDATION
3.1. Optical Stability and Detection Limits
The optical performance of the CRDS cells is illustrated in , which shows data recorded on the NOAA WP-3D on a stable flight leg at high altitude (490-mb pressure) during which sample air was filtered. Panel (a) shows 1-s average ringdown traces for each of the aerosol measurement channels (the gas phase reference channels are omitted for clarity). Of particular importance is the single exponential nature of decay traces for both pulsed YAG and continuous wave diode laser pumped cells, illustrated by the straight lines displayed on a log scale. The ringdown times measured at 490 mb are given, together with the ringdown times that would have been measured for Rayleigh scattering losses encountered at standard conditions of 1000 mb.
Panel (b) is an Allan variance plot (Werle et al. Citation1993), which describes how the instrument 1-sigma (1σ) detection limit, expressed as the Allan deviation, varies as a function of sample averaging time. Measurements from the same level flight leg spanning a total period of 190 s (190,000 ringdown events per channel) were used for this analysis. The detection limits of all channels are seen to improve out to averaging times of 10 s, illustrating the high mechanical stability of optical setup. Typically, flight data are averaged to 1-s temporal resolution where the detection limits of 532-nm channels are approximately 0.03 Mm−1 and that of 405- and 662-nm channels is 0.07 Mm−1. These detection limits compare very well with existing aerosol instrumentation based on CRDS (Baynard et al. Citation2007) and are several orders of magnitude below commonly encountered atmospheric extinction levels.
FIG. 2 In-flight optical performance of the CRDS instrument sampling aerosol-filtered air. Panel (a) shows 1-s average single-exponential ringdown traces recorded by each of the aerosol measurement channels. Panel (b) shows the instrument detection limit, expressed as the Allan deviation, as a function of averaging time. For 1-s averages (1000 laser shots) the instrument detection limit is <0.1 Mm−1 for all measurement channels.
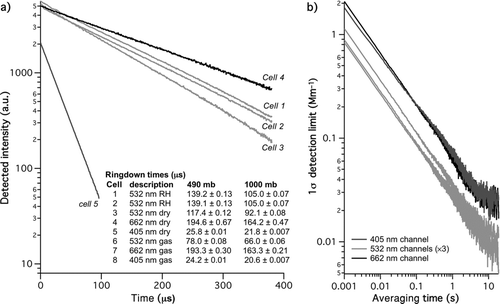
We note that while this analysis is useful in enabling the optical performance of the instrument to be characterized, it does not provide a good representation of the measurement uncertainty associated with aerosol sampling. It is well established that statistical variations in the aerosol population intersecting the CRDS detection beam contribute a significant source of random uncertainty in the aerosol CRDS measurements (Pettersson et al. Citation2004). The contribution of this source of uncertainty to airborne measurements is examined later in Section 3.5.
3.2. Pressure Stability
During flight it is important that the mechanical rigidity of the CRDS cells is sufficient to withstand distorting forces imparted by the intra-cell to cabin pressure differences encountered. One might imagine that frequent measurements of τ 0 could be used to track changes in optical alignment, thus eliminating errors from propagating to extinction calculations. However, adopting such an approach is problematic, as the optical misalignment that can occur between τ 0 measurement periods is potentially large and unpredictable in nature. Therefore, frequent τ 0 measurements are a poor substitute for a mechanically stable cell design.
FIG. 3 Dependence of τ 0 on pressure (square points) recorded during aircraft ascent and descent. Little variability (±0.2 μs) in τ 0 remains following correction of data to standard conditions of 1000 mb and 273.15 K (circular points).
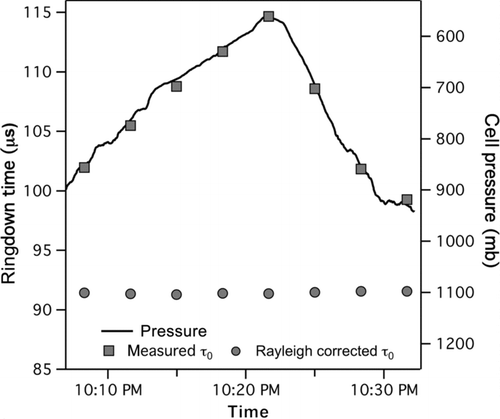
In order to illustrate the optical stability of the current cell design, shows data for one 532-nm channel recorded during a climb and descent leg during which the instrument was periodically sampling aerosol-filtered air. The measured τ 0 values (square points) vary by some 15 μs, as the cell pressure changes from 550 to 925 mb. This strong dependence is expected and results from the linear correlation of Rayleigh scattering with pressure. In order to remove the dependency, ringdown times were corrected to standard conditions of 1000 mb, 273.15 K, leaving only the following potential contributions to remaining variability: (i) cell misalignment, (ii) changes in concentration of gas phase species absorbing at 532 nm, and (iii) inherent measurement scatter associated with the determination of τ 0 from ringdown traces. The corrected τ 0 values (solid circles) show little variability (2σ = 0.2 μs), indicating small contributions from all of the aforementioned mechanisms. The variability that remains could reasonably be explained by small changes in gas phase absorption during the sampling period at levels of <0.1 Mm−1. We conclude that cell alignment changes during variable pressure operation do not represent a significant source of uncertainty to measurements of aerosol optical extinction using the current system.
3.3. Elevated Relative Humidity Measurements
Aerosol optical property measurements are most commonly made on dry particles at low RH. These conditions establish a useful commonality between measurements; however, understanding how optical properties change as aerosols take up water in response to elevated RH is also key information needed to evaluate climate impacts (Bates et al. Citation2006; Bian et al. Citation2009). The RH dependence of aerosol extinction is most commonly expressed as the quantity f(RH), defined as the ratio of light extinction measured at elevated RH to that at low reference RH. The magnitude of f(RH) is a function of both the aerosol composition and size (Baynard et al. Citation2006). The CRDS instrument is typically operated to measure f(RH) at two fixed elevated relative humidities of ∼70 and 95%. This enables the RH dependence of f(RH) to be characterized and the potential for large f(RH) values close to 100% RH to be explored.
In order to demonstrate the quantitative validity of the elevated RH measurements made using the current instrument, a series of laboratory experiments were conducted in which f(RH) was measured for size-selected ammonium sulphate particles and results compared to model calculations. Ammonium sulphate particles were generated by atomization, dried and size-selected using a custom-built differential mobility analyzer (DMA). For each aerosol size selected, the full dry size distribution, including larger multiply charged particles transmitted by the DMA, was characterized using an ultra high sensitivity aerosol spectrometer (UHSAS, Droplet Measurement Technologies, Boulder, CO, USA).
Modeled f(RH) values were calculated for each measured dry size distribution as follows: (i) equilibrium sizes at a series of elevated RHs were determined using Köhler theory (Seinfeld and Pandis Citation2006) with solution water activities given by Tang and Munkelwitz (Citation1994); (ii) size-resolved extinction at each elevated RH was calculated using Mie theory (Bohren and Huffman Citation1983) with refractive indices of wet particles determined through volume weighting of the refractive indices of water and ammonium sulphate; (iii) total extinction was calculated by summing across the full size distribution, and finally (iv) f(RH) was evaluated for each RH using the total calculated dry and wet particle extinctions. Uncertainty associated with calculated f(RH) values was determined by propagating estimated uncertainty in the measured RH (±2%) and measured particle diameters (±1%).
shows results for size-elected 300-nm-diameter particles. Based upon UHSAS size distribution measurements, contributions from doubly and triply charged particles were approximately 7.5% and 0.6% by number. The grey triangles show measurements from the high RH channel, which maintained a constant RH of ∼99% throughout the experiment. The black circles show measurements from the heated, variable RH channel, which operated at RH ranging from ∼38–98%. Error bars denote the 1σ uncertainty in f(RH) calculated by propagating uncertainty in the mean extinction for both dry and elevated RH channels. In general, there is excellent agreement between measured and modeled values, which provides a high degree of confidence in the quality of f(RH) measurements made using the current instrument.
FIG. 4 Laboratory measurements showing the relative humidity dependence of extinction for 300-nm dry diameter ammonium sulphate particles. The measured data show excellent agreement to predictions from Köhler theory (solid line). The dashed lines show the estimated uncertainty bounds for theoretical calculations.
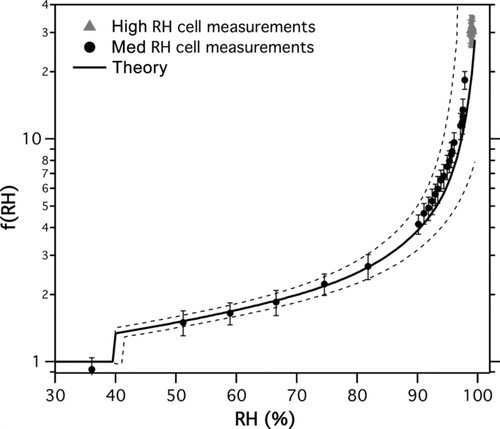
3.4. Gas Phase Reference Measurements
Three ringdown channels in the current instrument are dedicated to measuring gas phase absorption at each of the operating wavelengths. These measurements enable the separation of gas and aerosol contributions to total extinction measured in the remaining five aerosol channels. The gas phase measurement channels do not quantify absolute absorption, but rather monitor relative changes in absorption between filter periods. This approach is adopted as it removes the requirement for τ 0 to be measured using zero air. Instead, τ 0 is measured as all 8 instrument channels sample the same aerosol-filtered ambient air, which may contain absorbing species. Acquiring τ 0 on a common sample in this way ensures that the relative gas phase measurements are appropriate for correction of total extinction data. The purpose of this section is to examine the gas phase species contributing to absorption, to illustrate the need for high temporal resolution measurements for accurate correction of flight data, and to use the CalNex data to assess the necessity for performing gas phase measurements at all three instrument wavelengths.
FIG. 5 In-flight gas phase absorption measurements made during the CalNex field mission. Panel (a) shows absorption at 405 nm, where NO2 is the only absorbing species. The CRDS absorption compares extremely well with that calculated from NO2 concentrations measured concurrently aboard the NOAA WP-3D aircraft. Panel (b) shows the correlation between gas phase absorption measured at 405 and 532 nm during sixteen research flights. The data are well correlated owing to the dominance of NO2 absorption at both wavelengths.
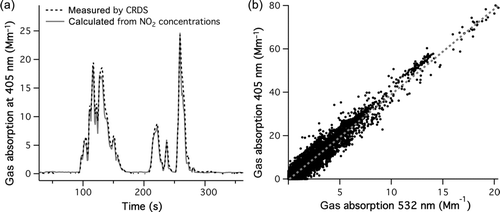
The most significant contribution of gas phase absorption occurs at 405 nm, where NO2 is the sole absorbing species. The NO2 absorption cross section at 405 nm is 6.23 × 10−19 cm2 (Vandaele et al. Citation1998) (1-Mm−1 extinction = 0.6 parts per billion by volume (ppbv) NO2 at standard conditions). At 532 nm, the NO2 absorption cross section is approximately four times smaller (1.5 × 10−19 cm2), yet it remains the principal absorbing species at this wavelength. Ozone (O3) also absorbs at 532 nm, albeit rather weakly with an absorption cross section of 2.8 × 10−21 cm2 (Burrows et al. Citation1999) (1-Mm−1 extinction = 135-ppbv O3 at standard conditions). At 662 nm both NO2 and O3 absorb weakly, with absorption cross sections of 3.87× 10−21 cm2 and 2.1 × 10−21 cm2, respectively. Water vapor also absorbs at this wavelength with a cross section of ∼1.9× 10−26 cm2 at the laser resolution of ∼0.6 nm (Rothman et al. Citation2009). However, measurements at 662 nm are exclusively made under dry conditions (water mixing ratios below 0.5% = 0.25-Mm−1 extinction at standard conditions), making water vapor extinction relatively insignificant. The final species absorbing at this wavelength is NO3, which although only present at night, absorbs strongly with an absorption cross section of 2.17× 10−17 cm2 (Orphal et al. Citation2003) (1-Mm−1 extinction = 2 parts per trillion by volume (pptv) NO3 at standard conditions). NO3 is however readily lost to the metallic surfaces used throughout the CRDS instrument. During five-night flights flown throughout CalNex, no evidence of NO3 absorption in either the aerosol or gas phase 662-nm channels was observed despite independent measurements indicating that NO3 concentrations regularly exceeded several hundred pptv (S. Brown, personal communication, 2011).
Given the principal contribution of NO2 and O3 to gas phase absorption, laboratory tests were performed to assess the transmission of these species through the filter used to remove particulates for gas measurements. At 1 LPM the transmission of both species through the filter exceeded 98%. Given that the magnitude of aerosol extinction generally dominates that of gases for ambient samples, these small transmission losses contribute very little uncertainty to aerosol extinction measurements.
shows example data collected by the 405-nm gas phase reference measurement channel. During this period the aircraft sampled at low altitude over the ocean and intercepted a number of narrow plumes containing NO2 derived from shipping activity. Measurements of absolute NO2 absorption were possible during this period, as the instrument was able to acquire τ 0 measurements in clean marine background air in which no NO2 was present. Also shown on this plot is the extinction calculated from accompanying NO2 concentration measurements (Ryerson et al. Citation2000). The temporal resolution of these data was reduced to match that of CRDS. Considering that the sampling inlet used for aerosol measurements is not optimized for the transmission of gaseous species, the level of agreement between these measurements is remarkably good. The data also provide an example of the fast variability of gas phase absorption that can be encountered during aircraft sampling, illustrating the necessity for fast gas phase measurements by the CRDS instrument.
shows the correlation between total gas phase absorption measured at 532 and 405 nm during sixteen research flights in California. As expected, the data are well correlated, as NO2 is the major absorber at both wavelengths. A fit to the gradient indicates that absorption at 405 nm is generally 3.95 times larger than at 532 nm, which agrees well with the ratio of the NO2 cross sections at these wavelength of 4.15. This result, together with observations during CalNex of minimal gas phase absorption at 662 nm, indicates that a single gas phase absorption measurement performed at 405 nm would be sufficient for correction of aerosol flight data at all three wavelengths. It is therefore likely that the 532- and 662-nm gas reference channels will be re-purposed for future deployments.
3.5. Measurement Uncertainty
A number of previous studies have examined the factors contributing to uncertainty in aerosol CRDS measurements (Pettersson et al. Citation2004; Baynard et al. Citation2007). They have highlighted the importance of both fundamental instrument uncertainty and uncertainty associated with the statistical fluctuations of aerosol particles within the optical sensing volume. The latter of these sources becomes particularly significant at low number concentrations and can act to make measurement uncertainty a function of specific characteristics of the aerosol population being sampled. Here we use both laboratory data and airborne data collected during the CalNex field campaign to evaluate typical uncertainties associated with field measurements made using the current instrument.
3.5.1. Precision
The precision associated with 1-s extinction measurements, which includes the aforementioned effects of aerosol number counting statistics, is calculated by propagating errors in 1-s mean values of τ and τ 0. For typical operation at 1-kHz laser repetition rates, the error in τ 0 is determined from the standard error of the mean of 1000 ringdown events. The error in τ is somewhat more complex to evaluate, as the time between successive laser shots at 1 kHz is insufficient for particles to redistribute themselves throughout the laser beam. Under these conditions, successive τ measurements are correlated and therefore Poisson statistics are not directly applicable (Butler et al. Citation2009). Indeed, evaluating the error in τ from the 1-s standard error of the mean of 1000 laser shots (as for τ 0) would significantly underestimate the true degree of uncertainty. This subtlety has not been noted in previous work examining the errors in the CRDS aerosol extinction data (Pettersson et al. Citation2004; Baynard et al. Citation2007), but becomes particularly important for fast time resolution measurements. This is illustrated in , which shows the standard deviation for a series of τ measurements as a function of averaging time for laboratory-generated 300-nm diameter ammonium sulphate aerosol and for a subset of measurements made during the CalNex mission. Each trace was calculated from a time series of single-shot τ values spanning less than 5 min. The three traces show similar behavior, with little change in standard deviation for averaging time of <1 s and improvement that approximately follows Poisson averaging (gradient of dotted lines) for periods of >1 s. These data indicate that the timescale associated with redistribution of aerosol in the laser volume is of the order of 1 s, which is comparable to the cell residence time. It follows that signal averaging only becomes effective at improving measurement precision for averaging periods greater than 1 s, and thus the benefits of operating at high laser repetition rates are not being fully realized in the current system.
FIG. 6 Effect of signal averaging on the standard deviation of τ measured for laboratory-generated ammonium sulphate aerosol and ambient aerosol during the CalNex field mission. Averaging time of 0.001 s represents single shot data. The dotted lines show the expected improvement based on the Poisson statistical averaging of single shot data.
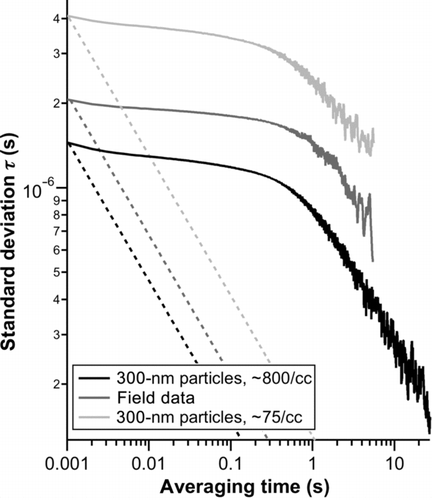
The precision of 1-s mean τ measurements, which contributes to the precision of extinction measurements, is calculated from the standard deviation of 1000 single-shot τ values divided by 1.5. The factor of 1.5 accounts for slight improvement in standard deviation that arises from averaging to 1-s resolution, as seen in . The 1-s precision is a factor of ∼21 greater than that evaluated assuming that the Poisson statistics apply. illustrates the validity of using this approach. It shows time series of both single shot and 1-s average extinction data, collected at 405 and 532 nm for laboratory-generated 300-nm diameter ammonium sulphate particles. The positive error bars were evaluated from the standard deviation of single-shot measurements divided by 1.5, whereas the negative error bars (which are smaller than the marker points) were evaluated assuming that Poisson statistics applied to the single-shot data. Clearly, the positive error bars better represent the observed variability in extinction. The negative error bars suggest that the observed variability was real and derived from instability in the aerosol source; however, the lack of correlation between 405- and 532-nm measurements indicates this to have not been the case.
FIG. 7 Single shot (1 kHz) and 1-s average extinction measured for 300-nm ammonium sulphate particles at 405 and 532 nm. The positive error bars indicate the true 1-s measurement precision and the negative error bars (which are smaller than the marker points) indicate the precision determined assuming that the Poisson statistics apply to the 1-kHz measurements.
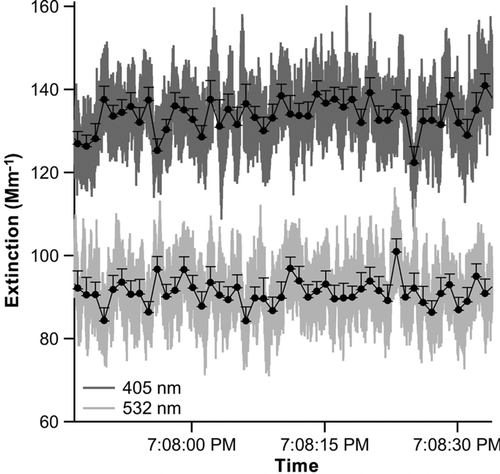
Extending this analysis to field data, shows the precision in 1-s extinction (calculated using an error in τ derived from the standard deviation of 1000 single shots divided by 1.5) measured for sub-2-μm aerosol during an 8-h research flight conducted over the Los Angeles basin region during May 2010. Data given for each of the five aerosol measurement channels show very similar performance, with 1-s precision generally within the range 25–65% for extinction of 5 Mm−1, better than 40% for extinction above 10 Mm−1, and 4–10% for extinction of 100 Mm−1. The tight correlations indicate that a relatively robust measure of precision can be attained from the magnitude of the extinction signal alone, i.e., without considering the specific characteristics of the aerosol population. The 1-s precision determined for the current system is similar to that reported by Pettersson et al., Citation(2004) of 30% for 5-Mm−1 extinction, but is larger than that given by Baynard et al., Citation(2007) of 7% for 5-Mm−1 extinction. These differences may arise from the smaller laser volume and slower aerosol flow rates used in the current system; future work will address improvements in both of these areas.
also shows the extinction precision for data averaged to 60 s. The 60-s precision is significantly better than that at 1 s given that for averaging times beyond 1 s the precision improves according to Poisson statistical averaging (see ). For extinction levels exceeding 10 Mm−1, the 60-s measurement precision is better than 5%.
3.5.2. Accuracy
The accuracy of dry extinction measurements, calculated by combining in quadrature contributions from systematic errors in RL (1%), τ and τ0 (1% for extinction <3000 Mm−1), purge flow dilution (0.2%), and gas phase corrections (0.4%), is better than 2% for the current system.
The accuracy of humidified particle extinction measurements is significantly larger and dominated by the accuracy with which cell RH is measured. RH errors are not important for measurements made under dry conditions because particle extinction shows little sensitivity to small changes in RH at low RH (see ). Based on calibrations using saturated salt solutions, the absolute accuracy of RH measured in each of the humidified cells is estimated to be ±2%. The extent to which this impacts the accuracy of measured extinction depends on the cell RH, together with the size distribution and composition of particles being measured. In order to explore this parameter space, a series of idealized calculations were performed for ammonium sulphate particles with (i) relatively narrow dry diameter size distributions characterized by Gaussians centered at 0.1, 0.4, and 0.7 μm with σ = 1.1, and (ii) a 300-s average sub-2-μm size distribution measured in-flight over the Los Angeles basin region during CalNex. Calculations were performed using Köhler theory (Seinfeld and Pandis Citation2006) to model particle growth and Mie theory (Bohren and Huffman Citation1983) to evaluate extinction cross sections for humidified particles.
summarizes the uncertainty estimates for each size distribution, with shaded regions representing the uncertainty bounds for the center RH ±2%. The measurement accuracy is seen to increase sharply at high RH and is most significant for the smallest particle sizes. Using the ambient flight data as a representative case, the extinction accuracy is approximately ±5% at RH = 85% and 10–20% at RH = 95%. We note that these results are likely to represent an upper limit for the accuracy of ambient measurements, as the assumption of pure ammonium sulphate particles will for most cases overestimate the hygroscopicity of ambient particles (Andreae and Rosenfeld Citation2008).
3.5.3. Total Uncertainty
The total uncertainty associated with extinction measurements made under specific conditions of interest can be evaluated using the data presented in sections 3.5.1 and 3.5.2. For 1-s data, dry extinction measurement uncertainty is dominated by random errors, except at extinction levels above c.a. 100 Mm−1, where systematic errors of the order of 1–2% become significant. Humidified particle extinction measurements are subject to larger uncertainties on account of larger systematic errors, which for one field-derived example reached c.a. 20% at 95% RH. We note that this analysis has only considered uncertainty introduced by the CRDS measurements themselves and not contributions from particle sampling efficiencies. The contribution from these sources will depend on the specific inlet, size cut, and sampling manifold used during field deployment. Especially for the measurement of dry extinction, it is possible that these sources of uncertainty could exceed those inherent to CRDS measurements themselves.
4. FIELD MEASUREMENTS
The instrument operated during eighteen research flights as part of the CalNex field campaign in May and June 2010. shows an illustrative example of 1-s resolution data recorded during this campaign on the 8 May 2010. The data were collected on one flight between 250–2200 m during a spiral descent near San Nicolas Island, located approximately 100 km off the coast of Los Angeles, California.
FIG. 9 Theoretical estimates of the accuracy of aerosol extinction measurements made at elevated relative humidity. The measurement accuracy depends strongly on particle size and composition. Example calculations are shown for ammonium sulphate particles for three idealized mono-disperse aerosol populations and for an example size distribution measured in-flight during CalNex.
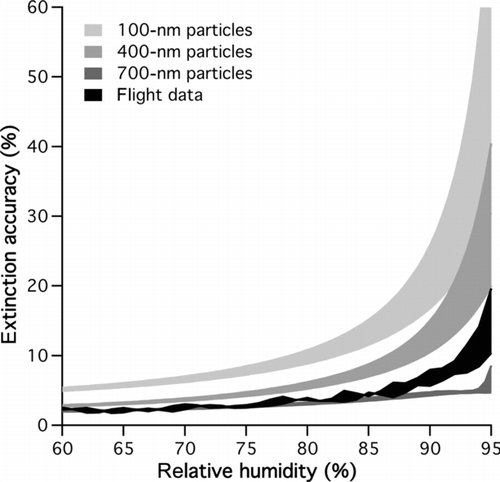
Panel (a) shows the measured sub-2-μm dry extinction at each of the 3 instrument wavelengths corrected to standard conditions. Elevated extinction is seen below 500 m and within a lofted layer spanning ∼1500–2000 m. We note that these levels were relatively low compared to those often seen in the Los Angeles basin area, which could reach several hundred inverse megameters for dry aerosol at 532 nm. However, this example was chosen to illustrate the instrument performance for air mass representative of clean to modestly polluted environments.
Panel (b) shows the extinction Ångström exponent determined from the dry extinction data (black line). It shows low values <1 at the lowest altitudes, indicative of large super-micrometer particles, and relatively uniform values of ∼1.6 for the elevated aerosol layer aloft, indicative of finer mode particles. Also shown on this plot (grey line) is the extinction Ångström exponent calculated from sub-2-μm-size distribution data (Brock et al. Citation2010), assuming particles have the refractive index of ammonium sulphate (n = 1.52). Considering the use of this simplifying assumption, excellent agreement between measured and modeled values is seen.
FIG. 10 1-s resolution aircraft measurements collected during a spiral descent off the coast of Los Angeles on the 8 May 2010. The CRDS measurements indicate the presence of hygroscopic coarse mode particles near the surface, together with a layer of hygroscopic fine mode particles aloft.
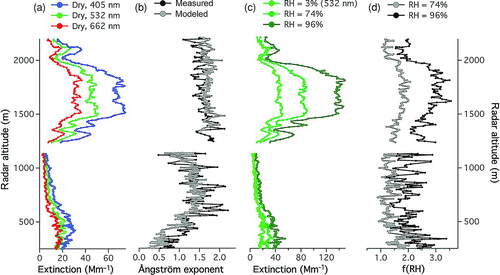
Panel (c) shows extinction measured in the elevated humidity channels, which ran at 74% and 96% RH during this period. These data were used to calculate the f(RH) extinction enhancement factors shown in panel (d), which reached ∼2 at 74% RH and ∼3.5 at 96% RH. The measurements indicate that both low- and high-altitude regions of elevated extinction were associated with notably hygroscopic aerosol.
Taken collectively, these data yield a coherent picture consistent with the presence of coarse, hygroscopic sea-salt particles near the surface, and fine mode aerosol aloft. Additional data indicated that the fine mode aerosol mass was dominated by partially neutralized sulphate and dew point temperatures within the lofted layer were below −15°C. These observations are consistent with aerosol that has undergone cloud-modified long-range transport (Brock et al. Citation2004).
5. SUMMARY
This paper has described the performance of an 8-channel cavity ringdown spectrometer designed to measure multi-wavelength aerosol extinction under dry and elevated RH conditions from aircraft. For data collected at 1 Hz, the in-flight instrument detection limit was better than 0.1 Mm−1, which is sufficient to detect ambient aerosol in all but the most pristine of environments. The aerosol measurement precision was evaluated using flight data and shown to be generally better than 40% for extinction >10 Mm−1 and 1-s averaging. The measurement accuracy was also examined and was shown to be <2% for dry aerosol, but significantly larger for humidified measurements, reaching ∼20% at 95% RH for one example flight. These results, together with additional laboratory and field data, have demonstrated the utility of the instrument for high temporal resolution, high-accuracy measurements of aerosol extinction from aircraft. In its current form, the instrument thus serves as a needed tool for investigating the radiative effects of aerosol particles in the climate system. Future modifications will target improved measurement precision, where a factor of ∼21 improvement should be possible based upon the averaging limit imposed by Poisson statistical considerations.
Acknowledgments
This work was supported by NOAA climate and air quality funding. JML thanks CIRES for the award of a visiting postdoctoral fellowship. The authors thank Drs. Ilana Pollack and Tom Ryerson for supplying NO2 measurement data and Dr. Charles Brock for useful discussions together with aerosol size distribution data from the WP-3D aircraft.
REFERENCES
- Anderson , T. L. , Charlson , R. J. , Bellouin , N. , Boucher , O. , Chin , M. Christopher , S. A. 2005 . An “A-Train” Strategy for Quantifying Direct Climate Forcing by Anthropogenic Aerosols . Bull. Am. Meteorol. Soc , 86 : 1795 – 1809 .
- Anderson , T. L. , Masonis , S. J. , Covert , D. S. , Ahlquist , N. C. , Howell , S. G. Clarke , A. D. 2003 . Variability of Aerosol Optical Properties Derived from in Situ Aircraft Measurements During ACE-Asia . J. Geophys. Res , 108 : 8647 doi:10.1029/2002JD003247
- Andreae , M. O. and Rosenfeld , D. 2008 . Aerosol-Cloud-Precipitation Interactions. Part 1. The Nature and Sources of Cloud-Active Aerosols . Earth Sci. Rev , 89 : 13 – 41 .
- Arnott , W. P. , Moosmuller , H. , Rogers , C. F. , Jin , T. F. and Bruch , R. 1999 . Photoacoustic Spectrometer for Measuring Light Absorption by Aerosol: Instrument Description . Atmos. Environ , 33 : 2845 – 2852 .
- Arnott , W. P. , Walker , J. W. , Moosmuller , H. , Elleman , R. A. , Jonsson , H. H. Buzorius , G. 2006 . Photoacoustic Insight for Aerosol Light Absorption Aloft from Meteorological Aircraft and Comparison with Particle Soot Absorption Photometer Measurements: DOE Southern Great Plains Climate Research Facility and the Coastal Stratocumulus Imposed Perturbation Experiments . J. Geophys. Res , 111 : D05S02 doi:10.1029/2005JD005964
- Bates , T. S. , Anderson , T. L. , Baynard , T. , Bond , T. , Boucher , O. Carmichael , G. 2006 . Aerosol Direct Radiative Effects Over the Northwest Atlantic, Northwest Pacific, and North Indian Oceans: Estimates Based on In-Situ Chemical and Optical Measurements and Chemical Transport Modeling . Atmos. Chem. Phys , 6 : 1657 – 1732 .
- Baynard , T. , Garland , R. M. , Ravishankara , A. R. , Tolbert , M. A. and Lovejoy , E. R. 2006 . Key Factors Influencing the Relative Humidity Dependence of Aerosol Light Scattering . Geophys. Res. Lett , 33 : L06813 doi:10.1029/2005GL024898
- Baynard , T. , Lovejoy , E. R. , Pettersson , A. , Brown , S. S. , Lack , D. Osthoff , H. 2007 . Design and Application of a Pulsed Cavity Ring-Down Aerosol Extinction Spectrometer for Field Measurements . Aerosol Sci. Technol , 41 : 447 – 462 .
- Berden , G. , Peeters , R. and Meijer , G. 2000 . Cavity Ring-Down Spectroscopy: Experimental Schemes and Applications . Int. Rev. Phys. Chem , 19 : 565 – 607 .
- Bian , H. , Chin , M. , Rodriguez , J. M. , Yu , H. , Penner , J. E. and Strahan , S. 2009 . Sensitivity of Aerosol Optical Thickness and Aerosol Direct Radiative Effect to Relative Humidity . Atmos. Chem. Phys , 9 : 2375 – 2386 .
- Bohren , C. F. and Huffman , D. R. 1983 . Absorption and Scattering of Light by Small Particles , New York : John Wiley & Sons .
- Bond , T. C. , Anderson , T. L. and Campbell , D. 1999 . Calibration and Intercomparison of Filter-Based Measurements of Visible Light Absorption by Aerosols . Aerosol Sci. Technol , 30 : 582 – 600 .
- Brock , C. A. , Cozic , J. , Bahreini , R. , Froyd , K. D. , Middlebrook , A. M. McComiskey , A. 2010 . Characteristics, Sources, and Transport of Aerosols Measured in Spring 2008 During the Aerosol, Radiation, and Cloud Processes Affecting Arctic Climate (ARCPAC) Project . Atmos. Chem. Phys. Discuss , 10 : 27361 – 27434 .
- Brock , C. A. , Hudson , P. K. , Lovejoy , E. R. , Sullivan , A. , Nowak , J. B. , Huey , L. G. , Cooper , O. R. , Cziczo , D. J. , de Gouw , J. , Fehsenfeld , F. C. Holloway , J. S. 2004 . Particle Characteristics Following Cloud-Modified Transport from Asia to North America . J. Geophys. Res. , 109 D23S26, Doi 10.1029/2003jd004198
- Brown , S. S. 2003 . Absorption Spectroscopy in High-Finesse Cavities for Atmospheric Studies . Chem. Rev. , 103 : 5219 – 5238 .
- Bucholtz , A. 1995 . Rayleigh-Scattering Calculations for The Terrestrial Atmosphere . Appl. Opt , 34 : 2765 – 2773 .
- Burrows , J. P. , Richter , A. , Dehn , A. , Deters , B. , Himmelmann , S. and Orphal , J. 1999 . Atmospheric Remote-Sensing Reference Data from GOME – 2. Temperature-Dependent Absorption Cross Sections of O-3 in the 231–794 nm Range . J. Quant. Spectrosc. Radiat. Transf. , 61 : 509 – 517 .
- Butler , T. J. A. , Mellon , D. , Kim , J. , Litman , J. and Orr-Ewing , A. J. 2009 . Optical-Feedback Cavity Ring-Down Spectroscopy Measurements of Extinction by Aerosol Particles . J. Phys. Chem. A , 113 : 3963 – 3972 .
- Clarke , A. and Kapustin , V. 2010 . Hemispheric Aerosol Vertical Profiles: Anthropogenic Impacts on Optical Depth and Cloud Nuclei . Science , 329 : 1488 – 1492 .
- Clarke , A. , McNaughton , C. , Kapustin , V. , Shinozuka , Y. , Howell , S. Dibb , J. 2007 . Biomass Burning and Pollution Aerosol Over North America: Organic Components and Their Influence on Spectral Optical Properties and Humidification Response . J. Geophys. Res. , 112 : D12S18 doi:10.1029/2006JD007777
- Dubovik , O. , Holben , B. , Eck , T. F. , Smirnov , A. , Kaufman , Y. J. King , M. D. 2002 . Variability of Absorption and Optical Properties of Key Aerosol Types Observed in Worldwide Locations . J. Atmos. Sci. , 59 : 590 – 608 .
- Everest , M. A. and Atkinson , D. B. 2008 . Discrete Sums for the Rapid Determination of Exponential Decay Constants . Rev. Sci. Instrum. , 79 : 023108 doi:10.1063/1.2839918
- Fuchs , H. , Dube , W. P. , Lerner , B. M. , Wagner , N. L. , Williams , E. J. and Brown , S. S. 2009 . A Sensitive and Versatile Detector for Atmospheric NO2 and NOx Based on Blue Diode Laser Cavity Ring-Down Spectroscopy . Environ. Sci. Technol. , 43 : 7831 – 7836 . Doi 10.1021/Es902067h
- Haywood , J. , Francis , P. , Osborne , S. , Glew , M. , Loeb , N. Highwood , E. 2003a . Radiative Properties and Direct Radiative Effect of Saharan Dust Measured by the C-130 Aircraft During SHADE: 1. Solar Spectrum . J. Geophys. Res. , 108 : 8577 doi:10.1029/2002JD002687
- Haywood , J. M. , Osborne , S. R. , Francis , P. N. , Keil , A. , Formenti , P. Andreae , M. O. 2003b . The Mean Physical and Optical Properties of Regional Haze Dominated by Biomass Burning Aerosol Measured from the C-130 Aircraft During SAFARI 2000 . J. Geophys. Res. , 108 : 8473 doi:10.1029/2002JD002226
- He , Y. B. and Orr , B. J. 2005 . Continuous-Wave Cavity Ringdown Absorption Spectroscopy with a Swept-Frequency Laser: Rapid Spectral Sensing of Gas-Phase Molecules . Appl. Opt. , 44 : 6752 – 6761 .
- Hegg , D. A. , Covert , D. S. , Crahan , K. and Jonssen , H. 2002 . The Dependence of Aerosol Light-Scattering on RH Over the Pacific Ocean . Geophys. Res. Lett. , 29 : 1219 doi:10.1029/2001GL014495
- Heintzenberg , J. and Charlson , R. J. 1996 . Design and Applications of the Integrating Nephelometer: A Review . J. Atmos. Ocean Tech. , 13 : 987 – 1000 .
- Howell , S. G. , Clarke , A. D. , Shinozuka , Y. , Kapustin , V. , McNaughton , C. S. Huebert , B. J. 2006 . Influence of Relative Humidity Upon Pollution and Dust During ACE-Asia: Size Distributions and Implications for Optical Properties . J. Geophys. Res. , 111 : D06205 doi:10.1029/2004JD005759
- Huffman , J. A. , Docherty , K. S. , Aiken , A. C. , Cubison , M. J. , Ulbrich , I. M. DeCarlo , P. F. 2009 . Chemically Resolved Aerosol Volatility Measurements from Two Megacity Field Studies . Atmos. Chem. Phys , 9 : 7161 – 7182 .
- Kinne , S. , Schulz , M. , Textor , C. , Guibert , S. , Balkanski , Y. Bauer , S. E. 2006 . An AeroCom Initial Assessment – Optical Properties in Aerosol Component Modules of Global Models . Atmos. Chem. Phys. , 6 : 1815 – 1834 .
- Lack , D. A. , Cappa , C. D. , Covert , D. S. , Baynard , T. , Massoli , P. Sierau , B. 2008 . Bias in Filter-Based Aerosol Light Absorption Measurements Due to Organic Aerosol Loading: Evidence from Ambient Measurements . Aerosol Sci. Technol. , 42 : 1033 – 1041 .
- Lack , D. A. , Lovejoy , E. R. , Baynard , T. , Pettersson , A. and Ravishankara , A. R. 2006 . Aerosol Absorption Measurement Using Photoacoustic Spectroscopy: Sensitivity, Calibration, and Uncertainty Developments . Aerosol Sci. Technol , 40 : 697 – 708 .
- Mallet , M. , Van Dingenen , R. , Roger , J. C. , Despiau , S. and Cachier , H. 2005 . In Situ Airborne Measurements of Aerosol Optical Properties During Photochemical Pollution Events . J. Geophys. Res. , 110 : D03205 doi:10.1029/2004JD005139
- Marshall , J. , Lohmann , U. , Leaitch , W. R. , Lehr , P. and Hayden , K. 2007 . Aerosol Scattering as a Function of Altitude in a Coastal Environment . J. Geophys. Res , 112 : D14203 doi:10.1029/2006JD007793
- Massoli , P. , Bates , T. S. , Quinn , P. K. , Lack , D. A. , Baynard , T. Lerner , B. M. 2009a . Aerosol Optical and Hygroscopic Properties During TexAQS-GoMACCS 2006 and their Impact on Aerosol Direct Radiative Forcing . J. Geophys. Res , 114 : D00F07 doi:10.1029/2008JD011604
- Massoli , P. , Murphy , D. M. , Lack , D. A. , Baynard , T. , Brock , C. A. and Lovejoy , E. R. 2009b . Uncertainty in Light Scattering Measurements by TSI Nephelometer: Results from Laboratory Studies and Implications for Ambient Measurements . Aerosol Sci. Technol. , 42 : 1064 – 1074 .
- Mazurenka , M. , Orr-Ewing , A. J. , Peverall , R. and Ritchie , G. A. D. 2005 . Cavity Ring-Down and Cavity-Enhanced Spectroscopy Using Diode Lasers . Annu. Rep. Prog. Chem., Sect. C , 101 : 100 – 142 .
- Moosmuller , H. , Varma , R. and Arnott , W. P. 2005 . Cavity Ring-Down and Cavity-Enhanced Detection Techniques for the Measurement of Aerosol Extinction . Aerosol Sci. Technol. , 39 : 30 – 39 .
- Morgan , W. T. , Allan , J. D. , Bower , K. N. , Esselborn , M. , Harris , B. Henzing , J. S. 2010 . Enhancement of the Aerosol Direct Radiative Effect by Semi-Volatile Aerosol Components: Airborne Measurements in North-Western Europe . Atmos. Chem. Phys. , 10 : 8151 – 8171 .
- Nakayama , T. , Hagino , R. , Matsumi , Y. , Sakamoto , Y. , Kawasaki , M. Yamazaki , A. 2010 . Measurements of Aerosol Optical Properties in Central Tokyo During Summertime Using Cavity Ring-Down Spectroscopy: Comparison with Conventional Techniques . Atmos. Environ. , 44 : 3034 – 3042 .
- O’Keefe , A. and Deacon , D. A. G. 1988 . Cavity Ring-Down Optical Spectrometer for Absorption-Measurements Using Pulsed Laser Sources . Rev. Sci. Instrum. , 59 : 2544 – 2551 .
- Orphal , J. , Fellows , C. E. and Flaud , P. M. 2003 . The Visible Absorption Spectrum of NO3 Measured by High-Resolution Fourier Transform Spectroscopy . J. Geophys. Res. , 108 : 4077 doi:10.1029/2002JD00248
- Pettersson , A. , Lovejoy , E. R. , Brock , C. A. , Brown , S. S. and Ravishankara , A. R. 2004 . Measurement of Aerosol Optical Extinction at 532 nm with Pulsed Cavity Ring Down Spectroscopy . J. Aero. Sci. , 35 : 995 – 1011 .
- Quinn , P. K. , Bates , T. S. , Coffman , D. , Onasch , T. B. , Worsnop , D. Baynard , T. 2006 . Impacts of Sources and Aging on Submicrometer Aerosol Properties in the Marine Boundary Layer Across the Gulf of Maine . J. Geophys. Res , 111 : D23S36 doi:10.1029/2006JD007582
- Radney , J. G. , Bazargan , M. H. , Wright , M. E. and Atkinson , D. B. 2009 . Laboratory Validation of Aerosol Extinction Coefficient Measurements by a Field-Deployable Pulsed Cavity Ring-Down Transmissometer . Aerosol Sci. Technol , 43 : 71 – 80 .
- Romanini , D. , Kachanov , A. A. , Sadeghi , N. and Stoeckel , F. 1997 . CW Cavity Ring Down Spectroscopy . Chem. Phys. Lett. , 264 : 316 – 322 .
- Rothman , L. S. , Gordon , I. E. , Barbe , A. , Benner , D. C. , Bernath , P. E. Birk , M. 2009 . The HITRAN 2008 Molecular Spectroscopic Database . J. Quant. Spectrosc. Radiat. Transf. , 110 : 533 – 572 .
- Ryerson , T. B. , Williams , E. J. and Fehsenfeld , F. C. 2000 . An Efficient Photolysis System for Fast-Response NO2 Measurements . J. Geophys. Res , 105 : 26447 doi:10.1029/2000JD900389
- Schwartz , S. E. , Charlson , R. J. , Kahn , R. A. , Ogren , J. A. and Rodhe , H. 2010 . Why Hasn't Earth Warmed as Much as Expected? . J. Climate , 23 : 2453 – 2464 .
- Seinfeld , J. H. and Pandis , S. N. 2006 . Atmospheric Chemistry and Physics: From Air Pollution to Climate Change , New York : John Wiley and Sons .
- Solomon , S. , Qin , D. , Manning , M. , Marquis , M. , Averyt , K. Tignor , M. M. B. 2007 . Climate Change 2007: The Physical Science Basis , Cambridge, , UK : Cambridge University Press .
- Strawa , A. W. , Castaneda , R. , Owano , T. , Baer , D. S. and Paldus , B. A. 2003 . The Measurement of Aerosol Optical Properties Using Continuous Wave Cavity Ring-Down Techniques . J. Atmos. Ocean Tech , 20 : 454 – 465 .
- Strawa , A. W. , Elleman , R. , Hallar , A. G. , Covert , D. , Ricci , K. Provencal , R. 2006 . Comparison of In Situ Aerosol Extinction and Scattering Coefficient Measurements Made During the Aerosol Intensive Operating Period . J. Geophys. Res , 111 : D05S03 doi:10.1029/2005JD006056
- Tang , I. N. and Munkelwitz , H. R. 1994 . Water Activities, Densities, and Refractive-Indexes of Aqueous Sulfates and Sodium-Nitrate Droplets of Atmospheric Importance . J. Geophys. Res. , 99 : 18801 doi:10.1029/94JD01345
- Textor , C. , Schulz , M. , Guibert , S. , Kinne , S. , Balkanski , Y. Bauer , S. 2006 . Analysis and Quantification of the Diversities of Aerosol Life Cycles within AeroCom . Atmos. Chem. Phys. , 6 : 1777 – 1813 .
- Vandaele , A. C. , Hermans , C. , Simon , P. C. , Carleer , M. , Colin , R. Fally , S. 1998 . Measurements of the NO2 Absorption Cross-Section from 42 000 cm−1 to 10 000 cm−1 (238–1000 nm) at 220 K and 294 K . J. Quant. Spectrosc. Radiat. Transf. , 59 : 171 – 184 .
- von der Weiden , S. L. , Drewnick , F. and Borrmann , S. 2009 . Particle Loss Calculator – A New Software Tool for the Assessment of the Performance of Aerosol Inlet Systems . Atmos. Meas. Tech. , 2 : 479 – 494 .
- Werle , P. , Mucke , R. and Slemr , F. 1993 . The Limits of Signal Averaging in Atmospheric Trace-Gas Monitoring by Tunable Diode-Laser Absorption-Spectroscopy (TDLAS) . App. Phys. B , 57 : 131 – 139 .
- Wilson , J. C. , Lafleur , B. G. , Hilbert , H. , Seebaugh , W. R. , Fox , J. Gesler , D. W. 2004 . Function and Performance of a Low Turbulence Inlet for Sampling Supermicron Particles from Aircraft Platforms . Aerosol Sci. Technol., , 38 : 790 – 802 .
- Zalicki , P. and Zare , R. N. 1995 . Cavity Ring-Down Spectroscopy for Quantitative Absorption-Measurements . J. Chem. Phys. , 102 : 2708 – 2717 .