Abstract
We investigated nanoparticle generation from a natural plant extract using the aerosol technique of the nebulization-thermal drying process, and tested its usefulness for antimicrobial air filtration. Sophora flavescens Ait. ethanolic extract was prepared as an antimicrobial natural-product suspension. Suspension droplets were generated using a single-jet Collison nebulizer, passed through an active carbon absorber to remove ethanol, and mixed and dried with sheath air. For drying, natural-product particles were exposed to 200°C for ∼1 s. Finally, particles were introduced into a scanning mobility particle sizer, and their size distribution and morphology were analyzed. For application of natural-product particles to antimicrobial air filtration, the nanosized particles generated were deposited continuously onto air filter medium at various times. Physical characteristics (filtration efficiency, pressure drop, and fiber morphology by scanning electron microscopy), and biological characteristics (antimicrobial tests against Staphylococcus epidermidis, Bacillus subtilis, and Escherichia coli bioaerosols) were then evaluated. We also analyzed the chemical composition of particles deposited on the filter surface. The results showed that the nanoparticles generated were spherical and demonstrated a polydisperse size distribution, ranging from several tens to several hundred nanometers. Although the filter pressure drop increased with the amount of nanoparticle on the filter, the bioaerosol filtration efficiency and antimicrobial activity were enhanced. In particular, the S. flavescens natural-product nanoparticle-deposited filters were more effective for removal of Gram-positive than Gram-negative bioaerosols. These results are promising for the implementation of this new technology for control of air quality against hazardous bioaerosols.
INTRODUCTION
In recent years, attention to antimicrobial air filtration has increased considerably, resulting in new research in the field of indoor air quality (IAQ) and public human health (Samet Citation1993; Lane et al. Citation2001). This developing concern is due to the realization that in developed societies, many people spend most of their time indoors (Spengler and Sexton Citation1983). Bioaerosols, such as airborne bacteria, viruses, fungi, and biological fragments, can flow freely with airflow movement and can spread over a wide area in a short period of time (Burge Citation1990). In suitable hosts, bioaerosols can cause both acute and chronic diseases, which may be infectious, allergenic, or toxigenic (Main Citation2003; Jung et al. Citation2009a). Numerous engineering solutions, such as ultraviolet germicidal irradiation (UVGI) (Lin and Li Citation2002; Walker and Ko Citation2007), air electric ion emission (Grinshpun et al. Citation2005), and thermal exposure, are under development as control methodologies for bioaerosols (Jung et al. Citation2009a, 2009c). However, air filtration technology is the most widely used for the removal of bioaerosols and the most efficient technology for indoor pollution control and prevention (Price et al. Citation1994).
However, indoor air bioaerosols accumulate in large quantities on the filters of heating, ventilation, and air-conditioning (HVAC) systems, where they can multiply, under certain conditions, particularly if high amounts of moisture are present on the filter (Maus et al. Citation2001). After air filtration, organic or inorganic materials deposited on the filter medium can contribute to microbial growth, inevitably leading to decreased filter efficacy and, most likely, to filter deterioration, with the eventual release of microorganisms. In particular, viable biological particles blown off from the filter surface can cause substantial damage to human health and can contaminate the ambient air environment. Volatile organic compounds produced by microbial metabolism can also be emitted from contaminated filters (Verdenelli et al. Citation2003). Although air filtration systems represent a good solution for the improvement of IAQ, they can become a source of contamination from microorganisms harmful to human health. Antimicrobial filters can provide significant benefits, because they can rapidly inactivate captured microorganisms and minimize the number of live/viable particles blown off from the filter by the passing air (Pyankov et al. Citation2008).
Recently, extracts of natural products with antimicrobial activity have been considered as new, efficient, and cost-effective materials for the development of antimicrobial filter media (Dixon Citation2001). Natural products, including chemical entities produced by living organisms, such as bacteria, fungi, and plants, have historically provided important solutions to medical and environmental health concerns (Cordell Citation2000; Butler Citation2004). Improvements in analytical and screening techniques have facilitated the isolation and characterization of the active ingredients, so-called natural products (Gademann Citation2006). Plant extracts, in particular, are known to have various pharmacological properties, including anti-inflammatory, antiviral, and antimicrobial effects. Bacterial and fungal infections are still a major human health concern, despite the development of antibiotics, but the presence of and increase in drug-resistant strains highlight the urgent need to develop novel antimicrobial agents and methods for processing them (Naimi et al. Citation2003). Treatment of filter surfaces with nano-sized natural product is an efficient method for enhancing their antimicrobial activity, because the nano-sized natural products provide the maximum possible specific surface area in contact with surrounding agents.
In the present study, we examined the characteristics of nanoparticles generated from an ethanolic plant extract using aerosol techniques, and tested their applicability to antimicrobial air filtration. As a test natural product in this study, we used Sophora flavescens Ait., a traditional herbal medicine, extracted from a perennial herb that is widely distributed in northeast Asia (De Naeyer et al. 2004). S. flavescens is also known to exhibit antibacterial activity against pathogens such as Staphylococcus, Streptococcus, and Enterococcus spp. (Kuroyanagi et al. Citation1999; Chen et al. Citation2005; Cha et al. Citation2007). In particular, S. flavescens has a high commercial value as a promising natural antimicrobial material, because of both the abundant supply of natural plants and their high antimicrobial activity.
Natural-product nanoparticles were generated by a nebulization-thermal drying process, which is a type of spray drying method. This process is the most commonly used technique for continuous generation of natural-product particles, and consists of two processes: spraying of the natural-product extract and drying of the generated droplet solvent (Coumans et al. Citation1994; Wagner and Warthesen Citation1995; Reineccius Citation2004). The physicochemical properties of the particles generated, such as size distribution, morphology, and composition, were evaluated. The natural-product nanoparticles generated were deposited on the surface of filter media consecutively and continuously during the aerosol process. Filtration characteristics, such as the particle filtration ratio and the pressure drop across the filter, and antimicrobial characteristics, were evaluated at various particle deposition densities.
MATERIALS AND METHODS
The experimental setup was divided into two primary components: (i) a system for aerosol generation and measurements and (ii) a system for evaluating filter performance ().
FIG. 1 Experimental configuration of (a) the nebulization-thermal drying process for generating natural-product nanoparticles and (b) the air filtration test system including bioaerosol generation. (Color figure available online.)
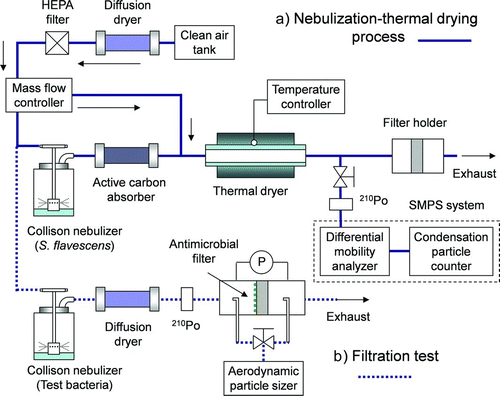
Plant Material and Extraction
Whole S. flavescens plants were purchased in February 2009 from Kyungdong Market in Seoul, Republic of Korea. A control specimen (D-192) was stored at the Natural Products Center at the Korea Institute of Science and Technology's Gangneung Institute at Gangneung, Republic of Korea. The dried whole S. flavescens plants (10 g) were refluxed with 94% ethanol for 3 h. After filtration, the ethanol extract was evaporated under reduced pressure.
Ethanolic Plant Extract for Nebulization
The resulting test natural-product powder was resuspended in pure ethanol (111727; Merck KGaA, Darmstadt, Germany) and sonicated for 10 min. Insoluble residue was then removed by centrifugation (5000 × g, 20 min). The final suspension was passed through a cellulose acetate membrane filter (National Scientific Co., Rockwood, TN) with a 0.45-μm pore size. Finally, a test suspension of 0.625% (w/v) was prepared. The physical properties of the test suspension were measured: electrical conductivity of 0.4 × 10−4 S/m, viscosity of 1.0 mPa·s, surface tension of 21 mN/m, and density of 785 kg/m3.
Nebulization-Thermal Drying System
Ethanolic plant extract droplets were obtained by nebulization using a single-jet collison nebulizer (BGI Inc., Waltham, MA), which has been widely used for generating aerosols from liquid suspensions (May Citation1973; Lefebvre Citation1989; Grinshpun et al. Citation2005; Eninger et al. Citation2009). In total, 20 mL of the test suspension was poured into the nebulizer, supplied with a 1.0 L/min airflow. Droplets of the natural-product extract suspension were passed through an active carbon absorber to remove the ethanol vapor, and mixed to 9.0 L/min of carrier airflow (). All airflows were filtered through a high-efficiency particulate air (HEPA) filter and diffusion dryer before introduction into the experimental system, which was controlled by mass flow controllers (MFC; 1179A Mass-Flo; MKS Instruments, Andover, MA). For the thermal drying process, we used a thermal electric heating system, consisting of a quartz tube (inner diameter, 29 mm; length, 700 mm; thickness, 1 mm) and an electric heating controller (MC-P; Poong Lim Industrial Co., Seoul, Republic of Korea). To prevent obstruction of the nanoparticle flow through the thermal tube, the heating coils were located outside the quartz tube and covered with insulating materials. Details of the thermal electric heating system and its center temperature distribution have been reported previously (Jung et al. Citation2009a, 2009c, 2010). The drying temperature used was 200°C (maximum) and the residence time of the airflow between the inlet and outlet of the quartz tube was about 1.4 s.
Measurement and Sampling
The size of the liquid droplets was further reduced by evaporation of solvent while passing through the thermal drying system, and the size and number of the nanoparticles finally generated were measured using a scanning mobility particle sizer (SMPS) system (Wang and Flagan Citation1990; Jung et al. Citation2006, 2007, 2009b), consisting of a differential mobility analyzer (DMA 3081; TSI Inc., Shoreview, MN) (Chen et al. Citation1998) and a condensation particle counter (CPC 3010; TSI Inc.). This instrument characterizes particle size distributions in the range of 14–673 nm, based on the particle's electrical mobility. The morphology and elemental composition of the airborne natural-product particles and particle-deposited filter fibers were investigated by scanning electron microscopy (SEM; XL30 ESEM-FEG; Philips Electron Optics, Eindhoven, The Netherlands) and an analytical transmission electron microscope (TEM; CM30; Philips Electron Optics). For this purpose, airborne natural-product particles were sampled on a copper TEM grid (type-A, coated with a carbon film; Ted Pella Inc., Redding, CA) using a nanometer aerosol sampler (3089; TSI Inc.) downstream of the nebulization-thermal drying system (Liu et al. Citation1967). Under an applied voltage of 9–10 kV, a nanometer aerosol sampler, which uses the electrostatic precipitation principle, was used to collect nanoparticles for approximately 5 min at a sampling flow rate of 1 L/min. The filter samples deposited by the natural-product nanoparticles were coated with Pt–Pd using an ion sputtering coater (model IB-3; Eiko Co., Ltd., Ibaraki, Japan) and analyzed under high vacuum (10−5 mbar) using SEM.
Preparation of Antimicrobial Filters
The natural-product nanoparticles generated were deposited continuously on the fibers of the inner filter holder (). Polyurethane resin fiber filters with a relatively high efficiency (fiber diameter of 10–20 μm, thickness of 0.3 mm, and packing density of about 33%) were used. After the nebulization-thermal drying process of the natural-product suspension, the natural-product nanoparticles generated were deposited consecutively on filters at varying deposition times of 30, 60, and 120 s. The quantity of deposited natural product on filters was determined by weighing filters before and after the particle deposition process. Before weighing of filter samples using a microbalance (Mettler MT5; Mettler-Toledo International Inc., Seoul, Republic of Korea) to an accuracy of 1 μg, all filter samples were kept in a diffusion dryer box packed with silica beads for 1 day. For chemical composition analysis, natural-product nanoparticles deposited on the filters were extracted three times with 94% ethanol. High-performance liquid chromatography (HPLC) analysis was conducted to determine the chemical composition of the extract using a 1200 Series HPLC instrument (Agilent Technologies, Santa Clara, CA) with a C18-type MGII 5-μm (Shiseido, Tokyo, Japan), 4.6 × 250-mm column (Fine Chemicals, Markham, ON, Canada), and applying an MeCN-H2O gradient of 20:80 to 100:0 in 45 min at 1 mL/min.
Preparation of Bacterial Suspensions
Staphylococcus epidermidis (KCTC 1917), Bacillus subtilis (KCCM 11316), and Escherichia coli (ATCC 8739) were selected as antimicrobial test bacteria. The first two, S. epidermidis and B. subtilis, which are Gram-positive bacteria, are commonly used in bioaerosol research together with E. coli (Gram-negative). Cultures of S. epidermidis and B. subtilis were grown in nutrient broth (NB; Becton Dickinson, Franklin Lakes, NJ) at 37°C for 24 h. E. coli cultures were grown in tryptic soy broth (TSB; Becton Dickinson) at 37°C for 18 h. Stationary-phase organisms were harvested by centrifugation (5000 × g, 10 min).
Filtration Tests
shows a schematic diagram of the experimental setup for filtration tests. For bioaerosol generation, bacteria pellets were carefully washed three times with sterilized deionized water (SDW) using a centrifuge (RC-5B, Sorval Co., CT, USA; 5000 × g, 10 min) to remove residual particles including the components of the nutrient broth. The SDW was also used for dilution to obtain the final bacterial suspension. A 30 mL aliquot was placed in the 6-jet collison nebulizer (BGI Inc.) and nebulized at an airflow rate of 5 L/min. Dispersed bioaerosols were passed through the diffusion dryer to remove moisture and through a 210Po neutralizer to reduce the electrical charge on the bioaerosols, and then introduced into the filter holder where a prepared antimicrobial filter was installed. The face velocity on the filter was controlled from 0.1 to 1.5 m/s using a mass flow controller. The pressure drop across the filter was measured using a micromanometer (FCO12; Furness Controls Ltd., Bexhill, UK). The particle size distribution and the concentrations of the bioaerosols were measured upstream and downstream of the filter using the aerodynamic particle sizer (APS 3320, TSI Inc.), which sizes airborne particles in the range 0.5–10 μm using a time-of-flight (TOF) technique that measures aerodynamic diameter in real time.
The overall particle filtration efficiency (η) is defined as
Antimicrobial Tests
When determining the antimicrobial effect of the filter samples on the measured bioaerosol concentrations and culturability, test organisms were generated during a fixed period of 5 min in all the experiments to obtain similar loading for all filters. Each filter was removed from the filter holder immediately after bioaerosol sampling and placed into a Petri dish (outer diameter, 55 mm) with bacteria residence time of 30 min. Each filter was then soaked in a 50 mL conical tube (Corning Inc., Acton, MA) containing 5 mL (V extraction) of an extraction fluid of phosphate-buffered saline (PBS, pH 7.4) with 0.01% Tween 80 (Biosesang Inc., Sungnam, Republic of Korea). Samples in extraction fluid were vortexed for 2 min. Samples in solution then underwent an agitation step in an ultrasonic bath (Fisher Ultrasonic Cleaners, Model FS20, 3 qt., Thermo Fisher Scientific Inc.) with ice for 10 min. Aliquots of 0.1 mL of the extract suspensions were serially diluted with PBS, plated on nutrient agar (tryptic soy agar for E. coli), and incubated at 37°C for 24 h (12 h for E. coli). After incubation, the colonies that formed on the plates were counted.
A relative microbial survival (RMS) was finally calculated using the following equations:
Additionally, we checked the natural decay of the test bacterial bioaerosols with various residence times (0–60 min) on the control filter medium. A natural decay was calculated using the following equations:
Statistical Analysis
The data were analyzed statistically by analysis of variance, t-test, and linear regression using the SAS software (ver. 9.1 for Microsoft Windows).
RESULTS AND DISCUSSION
Characteristics of Airborne Natural-Product Nanoparticles
shows the size distribution of the natural-product nanoparticles (S. flavescens Ait.) measured using an SMPS and a TEM. The nebulization process produced nanoparticles with a broad size distribution, ranging from a few to several hundred nanometers. The particle size distribution showed a log-normal distribution of a monomodal curve with a mode diameter (peak) of 109.4 nm, a geometric mean diameter (GMD) of 110.7 nm, and a geometric standard deviation (GSD) >1.64 (high polydispersity). In the TEM micrograph (), the morphology of generated nanoparticles was mostly nonagglomerated and spherical. Although we tried to obtain additional elemental composition data from the energy-dispersive spectrometer (EDS) analysis using TEM, no significant inorganic element was detected. The chemical composition of S. flavescens extract is well known. The major compounds are flavonoids and representative compounds have been identified (Kuroyanagi et al. Citation1999; Kang et al. Citation2000). In the ethanolic extract of S. flavescens used in the present study, the major components were lavandulyl flavanones (such as kurarinone (12.525%), prenylated flavonoids (such as sophoraflavanone G (3.248%)), and a lavandulyl chalcone called kuraridin (0.818%) (data not shown). These compounds demonstrate well-known biological activities. For example, sophoraflavanone G displays antibacterial, anti-inflammatory, and anticancer effects (Kim et al. Citation2002; Fakhimi et al. Citation2006), while kurarinone induces apoptosis in tumor cells and exhibits antioxidant and phytoestrogenic effects (De Naeyer et al. 2004; Piao et al. Citation2006; Han et al. Citation2007). In addition to flavonoids, many quinolizidine alkaloids and triterpenoids have also been reported in S. flavescens Ait. (Yagi et al. Citation1989; Yamaki et al. Citation2006). The alkaloids matrine and oxymatrine, in particular, are known to demonstrate strong diuretic, antipyretic, antifungal, antiulcer, antibacterial, and antidermatophytic effects (Yixiang et al. Citation2010). We also compared the major chemical compounds between the original S. flavescens Ait. ethanolic extract and the nanoparticles generated from it by the nebulization-thermal drying process of the aerosol technique. The nanoparticles generated maintained the same chemical composition, compared with the original S. flavescens ethanolic extract, despite the thermal drying procedure ().
FIG. 2 Particle size distribution and TEM image of generated natural-product nanoparticles. (Color figure available online.)
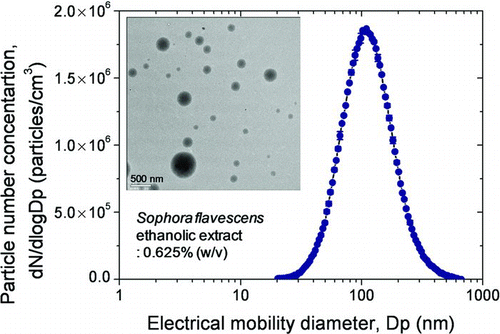
TABLE 1 Comparison of the major chemical compounds in the ethanolic extract of S. flavescens Ait. and the nanoparticles generated
Preparation of Natural-Product Nanoparticle-Deposited Filters
shows the weight of nanoparticles deposited on filters per unit cross-sectional filter surface area (μg/cm2) and images of filters with various nanoparticle deposition times. As the particle deposition time increased, from 30 to 120 s, the total weight per unit filter surface area increased linearly, from 55.2 to 317.3 μg/cm2 (y = 2.484 x; R 2 = 0.9905). shows SEM micrographs of the control and antimicrobial filters with nanoparticle deposition times of 30 and 120 s, respectively. The results showed that the control filter had a smooth and clear surface; whereas filter samples with natural-product nanoparticles deposited had relatively rough surfaces. As the particle deposition time increased, the particles on the surface of filter fibers became denser.
FIG. 3 Variation in the weight of nanoparticles deposited on filters per unit cross-sectional filter surface area (μg/cm2) at different deposition times. The error bars indicate standard deviations (n = 5). (Color figure available online.)
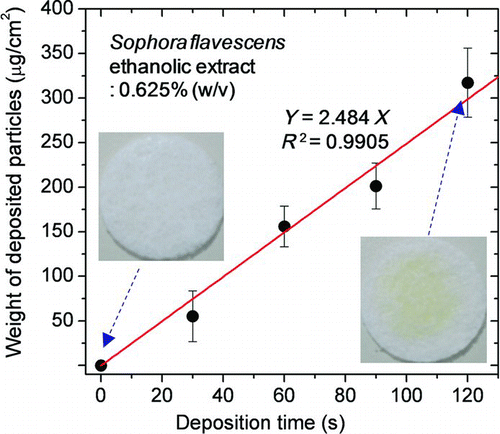
Filtration Testing of Natural-Product Nanoparticle-Deposited Filters
Pressure drops across the filters were measured (). The amount of nanoparticles deposited on the filter increased linearly with filter pressure drop. As the face velocity of airflow increased, from 0.11 to 0.32 m/s (0.5–1.5 L/min of airflow rate), the filter pressure drop increased, from 3.4 ± 0.41 to 11.2 ± 1.36 mmH2O and from 5.3 ± 0.59 to 17.7 ± 1.57 mmH2O at 60 s and 120 s particle deposited filters, respectively, compared with the control filter (2.9 ± 0.18 to 8.15 ± 0.54 mmH2O). According to previous studies, significant changes in the pressure drop across fibrous filters, caused by deposition of solid aerosol particles, normally occur on the order of several grams per square meter of particle deposition (Japuntich et al. Citation1997; Thomas et al. Citation2001). As mentioned above, the range of total weight per unit filter surface area of tested filters was from 55.2 to 317.3 μg/cm2 (0.552–3.173 g/m2). Thus, the amount of natural-product nanoparticles on the filter needs to be optimized to avoid an excessive increase in their pressure drop, considering their antimicrobial activity.
FIG. 6 (a) RMS of the natural-product nanoparticle-deposited filters against test bacterial bioaerosols. (b) Natural decay of test bacterial bioaerosols on the control filter. The error bars indicate standard deviations (n = 5). (Color figure available online).
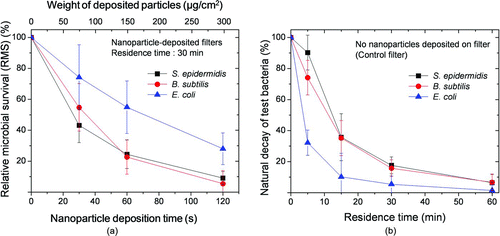
The aerodynamic particle size distribution of test bioaerosols generated by the nebulizer is shown in Figure S1a (online supplemental information). The peak particle concentrations of test bacteria bioaerosols were clearly recognizable with an aerodynamic diameter of about 0.90 μm (0.84 μm for E. coli) as the mode diameter. The GMDs and GSDs were 0.84 ± 0.012 μm and 1.193 ± 0.001 for S. epidermidis, 0.87 ± 0.010 μm and 1.209 ± 0.002 for B. subtilis, and 0.85 ± 0.012 μm and 1.164 ± 0.002 for E. coli, respectively.
As the deposition time of nanoparticles on filters increased, from 0 (control) to 120 s, the filtration efficiency of test bacteria bioaerosols increased, from 0.86 ± 0.013 to 0.96 ± 0.030 at a face velocity of 0.11 m/s, from 0.84 ± 0.008 to 0.93 ± 0.027 at 0.21 m/s, and from 0.79 ± 0.062 to 0.91 ± 0.021 at 0.32 m/s, respectively (Figure S1b; online supplemental information). However, we found no statistically significant difference in filtration efficiency between 30 s and 120 s particle-deposited filters (p > 0.05 by paired t-test). Additionally, the three bacteria species likely had little overall impact on the filtration efficiency because of their similar size distribution. In the present study, a flat panel-type filter was used for the filtration test to assess the effects of natural-product particle deposition on filtration efficiency and filter pressure drop. In real situations, however, folded filter media are typically used to enlarge the filtration area. By enlarging the filtration area, the filtration efficiency and pressure drop can be increased and decreased, respectively.
Antimicrobial Testing of Natural-Product Nanoparticle-Deposited Filters
The antimicrobial effects of filters containing various amounts of nanoparticle-sized natural product were characterized using the culture method. In , data are expressed as the RMS of the test bacterial bioaerosols. The RMS of test microorganisms decreased with increasing levels of nanoparticles deposited. In particular, the S. epidermidis decay trend was similar to that of B. subtilis (p > 0.05 by paired t-test), and the presence of varying amounts of natural-product nanoparticles clearly changed the behavior of the decay curve. However, for the 120-s particle-deposited filter, although >91% of both Gram-positive bacteria were inactivated within 30 min of residence time, the RMS of E. coli (28 ± 10.2%) was relatively higher than that of the others. The lower antimicrobial response of Gram-negative bacterial strains could possibly be explained by the biochemical and physiological characteristics of those bacteria. It is well known that the outer membrane of Gram-negative bacteria is predominantly constructed of tightly packed lipopolysaccharide molecules, which provide an effective resistance barrier (Papo and Shai Citation2005).
To obtain more detailed information about this result, we evaluated the natural decay of test microorganisms with various residence times (). For the control filter, >93% of test microorganisms were inactivated within 60 min of residence time. As the residence time increased, the RMS of test microorganisms decreased. Although the decay trends of the Gram-positive bacteria S. epidermidis and B. subtilis were similar to those of Gram-negative E. coli, E. coli inactivation was relatively higher than that of the others because E. coli is sensitive to environmental stresses, such as temperature, humidity, and impaction (Stewart et al. Citation1995; Jung et al. Citation2009a). From the results of and b, we conclude that those Gram-negative E. coli bacteria that survived the natural decay were more resistant to the antimicrobial effect by natural-product nanoparticles than the corresponding bacteria of the other two species.
CONCLUSIONS
In conclusion, the nebulization-thermal drying process of the aerosol technique can successfully generate natural-product nanoparticles that can be applied to antimicrobial air filters without degradation of the material's chemical or biological properties. Our results indicate that filters with natural-product nanoparticles deposited on them may be effective in the control of bioaerosols by inhibition of bacterial survival on the filters. Although the nebulization-thermal drying process did not influence the chemical composition of the S. flavescens extract, the pressure drop of the nanoparticle-deposited filters increased with increasing nanoparticle deposition time. Because the inactivation efficiency of the antimicrobial filter is affected by the amount of nanoparticles, nanoparticle content needs to be optimized according to the species and concentration of background bioaerosols in the intended area of application of the natural product. Additionally, a long-term evaluation of the chemical stability and antimicrobial performance of generated nanoparticles should be conducted to assess the efficacy of nanoparticle-deposited filters in real world situations. The aerosol technique suggested in this study allows the steady generation of natural-product nanoparticles and so has potential applications in maintenance of IAQ for public human health as well as in biomedical engineering for functional material coatings and deposition. These results are promising for the implementation of this new technology to control air quality against hazardous bioaerosols.
uast_a_602763_sup_20271404.zip
Download Zip (54.9 KB)Acknowledgments
This research was supported by the Converging Research Center Program funded by the Ministry of Education, Science and Technology (2010K001141).
[Supplementary materials are available for this article. Go to the publisher's online edition of Aerosol Science and Technology to view the free supplementary files.]
REFERENCES
- Burge , H. 1990 . Bioaerosols: Prevalence and Health Effects in the Indoor Environment . J. Allergy Clin. Immunol. , 86 : 687 – 701 .
- Burton , N. C. , Adhikari , A. , Grinshpun , S. A. , Hornung , R. and Reponen , T. 2005 . The Effect of Filter Material on Bioaerosol Collection of Bacillus subtilis Spores Used as a Bacillus anthracis Simulant . J. Environ. Monit. , 7 : 475 – 480 .
- Butler , M. S. 2004 . The Role of Natural Product Chemistry in Drug Discovery . J. Nat. Prod. , 67 : 2141 – 2153 .
- Cha , J. D. , Jeong , M. R. , Jeong , S. I. and Lee , K. Y. 2007 . Antibacterial Activity of Sophoraflavanone G Isolated from the Roots of Sophora flavescens . J. Microbiol. Biotechnol. , 17 : 858 – 864 .
- Chen , D. R. , Pui , D. Y. H. , Hummes , D. , Fissan , H. , Quant , F. R. and Sem , G. J. 1998 . Design and Evaluation of a Nanometer Aerosol Differential Mobility Analyzer (Nano-DMA) . J. Aerosol Sci. , 29 : 497 – 509 .
- Chen , L. , Cheng , X. , Shi , W. , Lu , Q. , Go , V. L. , Heber , D. and Ma , L. 2005 . Inhibition of Growth of Streptococcus mutans, Methicillin-Resistant Staphylococcus aureus, and Vancomycin-Resistant Enterococci by Kurarinone, a Bioactive Flavonoid Isolated from Sophora flavescens . J. Clin. Microbiol. , 43 : 3574 – 3575 .
- Cordell , G. A. 2000 . Biodiversity and Drug Discovery—a Symbiotic Relationship . Phytochemistry , 55 : 463 – 480 .
- Coumans , W. , Kerkhof , P. and Bruin , S. 1994 . Theoretical and Practical Aspects of Aroma Retention in Spray Drying and Freeze Drying . Dry. Technol. , 12 : 99 – 149 .
- De Naeyer , A. , Berghe , W. V. , Pocock , V. , Milligan , S. , Haegeman , G. and De Keukeleire , D. 2004 . Estrogenic and Anticarcinogenic Properties of Kurarinone, a Lavandulyl Flavanone from the Roots of Sophora flavescens . J. Nat. Prod. , 67 : 1829 – 1832 .
- Dixon , R. 2001 . Natural Products and Plant Disease Resistance . Nature , 411 : 843 – 847 .
- Eninger , R. M. , Hogan , C. J. , Biswas , P. , Adhikari , A. , Reponen , T. and Grinshpun , S. A. 2009 . Electrospray Versus Nebulization for Aerosolization and Filter Testing with Bacteriophage Particles . Aerosol Sci. Technol. , 43 : 298 – 304 .
- Fakhimi , A. , Iranshahi , M. , Emami , S. A. , Amin-Ar-Ramimeh , E. , Zarrini , G. and Shahverdi , A. R. 2006 . Sophoraflavanone G from Sophora Pachycarpa Enhanced the Antibacterial Activity of Gentamycin Against Staphylococcus aureus . Z. Naturforsch. C , 61 : 769 – 772 .
- Gademann , K. 2006 . Natural Product Hybrids . CHIMIA , 60 : 841 – 845 .
- Grinshpun , S. A. , Mainelis , G. , Trunov , M. , Adhikari , A. , Reponen , T. and Willeke , K. 2005 . Evaluation of Ionic Air Purifiers for Reducing Aerosol Exposure in Confined Indoor Spaces . Indoor Air , 15 : 235 – 245 .
- Han , J. , Sun , M. , Cui , Y. , Wang , T. , Zhang , W. , Guo , M. , Zhou , Y. , Liu , W. , Zhang , M. , Duan , J. , Xiong , S. , Yao , M. and Yan , X. 2007 . Kushen Flavonoids Induce Apoptosis in Tumor Cells by Inhibition of NF-kappaB Activation and Multiple Receptor Tyrosine Kinase Activities . Phytother. Res. , 21 : 262 – 268 .
- Japuntich , D. , Stenhouse , J. and Liu , B. 1997 . Effective Pore Diameter and Monodisperse Particle Clogging of Fibrous Filters . J. Aerosol Sci. , 28 : 147 – 158 .
- Jung , J. , Lee , J. , Kim , S. and Bae , G. 2010 . Size Reduction of Aspergillus Versicolor Fungal Bioaerosols During a Thermal Heating Process in Continuous-Flow System . J. Aerosol Sci. , 41 : 602 – 610 .
- Jung , J. H. , Lee , J. E. and Kim , S. S. 2009a . Thermal Effects on Bacterial Bioaerosols in Continuous Air Flow . Sci. Total Environ. , 407 : 301 – 317 .
- Jung , J. H. , Lee , J. E. and Kim , S. S. 2009b . Generation of Nonagglomerated Airborne Bacteriophage Particles Using an Electrospray Technique . Anal. Chem. , 81 : 2985 – 2990 .
- Jung , J. H. , Lee , J. E. , Lee , C. H. , Kim , S. S. and Lee , B. U. 2009c . Treatment of Fungal Bioaerosols by a High-Temperature, Short-Time Process in a Continuous-Flow System . Appl. Environ. Microbiol. , 75 : 2742 – 2749 .
- Jung , J. H. , Oh , H. C. , Ji , J. H. and Kim , S. S. 2007 . In-Situ Gold Nanoparticle Generation Using a Small-Sized Ceramic Heater with a Local Heating Area . Mater. Sci. Forum , 544–545 : 1001 – 1004 .
- Jung , J. H. , Oh , H. C. , Noh , H. S. , Ji , J. H. and Kim , S. S. 2006 . Metal Nanoparticle Generation Using a Small Ceramic Heater With a Local Heating Area . J. Aerosol Sci. , 37 : 1662 – 1670 .
- Kang , T. H. , Jeong , S. J. , Ko , W. G. , Kim , N. Y. , Lee , B. H. , Inagaki , M. , Miyamoto , T. , Higuchi , R. and Kim , Y. C. 2000 . Cytotoxic Lavandulyl Flavanones from Sophora flavescens . J. Nat. Prod. , 63 : 680 – 681 .
- Kim , D. W. , Chi , Y. S. , Son , K. H. , Chang , H. W. , Kim , J. S. , Kang , S. S. and Kim , H. P. 2002 . Effects of Sophoraflavanone G, a Prenylated Flavonoid from Sophora flavescens, on Cyclooxygenase-2 and In Vivo Inflammatory Response . Arch. Pharm. Res. , 25 : 329 – 335 .
- Kuroyanagi , M. , Arakawa , T. , Hirayama , Y. and Hayashis , T. 1999 . Antibacterial and Antiandrogen Flavonoids from Sophora flavescens . J. Nat. Prod. , 62 : 1595 – 1599 .
- Lane , H. C. , La Montagne , J. and Fauci , A. S. 2001 . Bioterrorism: A Clear and Present Danger . Nat. Med. , 7 : 1271 – 1273 .
- Lefebvre , A. H. 1989 . Atomization and Sprays , New York : Hemisphere .
- Lin , C. Y. and Li , C. S. 2002 . Control Effectiveness of Ultraviolet Germicidal Irradiation on Bioaerosols . Aerosol Sci. Technol. , 36 : 474 – 478 .
- Liu , B. Y. , Whitby , K. T. and Yu , H. H. 1967 . Electrostatic Aerosol Sampler for Light and Electron Microscopy . Rev. Sci. Instrum. , 38 : 100 – 102 .
- Main , C. 2003 . Aerobiological, Ecological, and Health Linkages . Environ. Int. , 29 : 347 – 349 .
- Maus , R. , Goppelsroder , A. and Umhauer , H. 2001 . Survival of Bacterial and Mold Spores in Air Filter Media . Atmos. Environ. , 35 : 105 – 113 .
- May , K. R. 1973 . The Collison Nebulizer: Description, Performance and Application . J. Aerosol Sci. , 4 : 235 – 243 .
- Naimi , T. S. , LeDell , K. H. , Como-Sabetti , K. , Borchardt , S. M. , Boxrud , D. J. , Etienne , J. , Johnson , S. K. , Vandenesch , F. , Fridkin , S. and O’Boyle , C. 2003 . Comparison of Community-and Health Care-Associated Methicillin-Resistant Staphylococcus aureus Infection . JAMA , 290 : 2976 – 2984 .
- Papo , N. and Shai , Y. 2005 . A Molecular Mechanism for Lipopolysaccharide Protection of Gram-Negative Bacteria from Antimicrobial Peptides . J. Biol. Chem. , 280 : 10378 – 10387 .
- Piao , X. L. , Piao , X. S. , Kim , S. W. , Park , J. H. , Kim , H. Y. and Cai , S. Q. 2006 . Identification and Characterization of Antioxidants from Sophora flavescens . Biol. Pharm. Bull. , 29 : 1911 – 1915 .
- Price , D. , Simmons , R. , Ezeonu , I. , Crow , S. and Ahearn , D. 1994 . Colonization of Fiberglass Insulation Used in Heating, Ventilation and Air Conditioning Systems . J. Ind. Microbiol. Biotechnol. , 13 : 154 – 158 .
- Pyankov , O. , Agranovski , I. , Huang , R. and Mullins , B. 2008 . Removal of Biological Aerosols by Oil Coated Filters . CLEAN—Soil, Air, Water , 36 : 609 – 614 .
- Reineccius , G. 2004 . The Spray Drying of Food Flavors . Dry. Technol. , 22 : 1289 – 1324 .
- Samet , J. 1993 . Indoor Air Pollution: A Public Health Perspective . Indoor Air , 3 : 219 – 226 .
- Spengler , J. and Sexton , K. 1983 . Indoor Air Pollution: A Public Health Perspective . Science , 221 : 9 – 17 .
- Stewart , S. L. , Grinshpun , S. A. , Willeke , K. , Terzieva , S. , Ulevicius , V. and Donnelly , J. 1995 . Effect of Impact Stress on Microbial Recovery on an Agar Surface . Appl. Environ. Microbiol. , 61 : 1232 – 1239 .
- Thomas , D. , Penicot , P. , Contal , P. , Leclerc , D. and Vendel , J. 2001 . Clogging of Fibrous Filters by Solid Aerosol Particles Experimental and Modelling Study . Chem. Eng. Sci. , 56 : 3549 – 3561 .
- Verdenelli , M. , Cecchini , C. , Orpianesi , C. , Dadea , G. and Cresci , A. 2003 . Efficacy of Antimicrobial Filter Treatments on Microbial Colonization of Air Panel Filters . J. Appl. Microbiol. , 94 : 9 – 15 .
- Wagner , L. A. and Warthesen , J. J. 1995 . Stability of Spray Dried Encapsulated Carrot Carotenes . J. Food Sci. , 60 : 1048 – 1053 .
- Walker , C. and Ko , G. 2007 . Effect of Ultraviolet Germicidal Irradiation on Viral Aerosols . Environ. Sci. Technol. , 41 : 5460 – 5465 .
- Wang , S. C. and Flagan , R. C. 1990 . Scanning Electrical Mobility Spectrometer . Aerosol Sci. Technol. , 13 : 230 – 240 .
- Wang , Z. , Reponen , T. , Grinshpun , S. A. , Gorny , R. L. and Willeke , K. 2001 . Effect of Sampling Time and Air Humidity on the Bioefficiency of Filter Samplers for Bioaerosol Collection . J. Aerosol Sci. , 32 : 661 – 674 .
- Yagi , A. , Fukunaga , M. , Okuzako , N. , Mifuchi , I. and Kawamoto , F. 1989 . Antifungal Substances from Sophora flavescens . Shoyakugaku Zasshi , 43 : 343 – 347 .
- Yamaki , M. , Kashihara , M. and Takagi , S. 2006 . Activity of Ku Shen Compounds Against Staphylococcus aureus and Streptococcus mutans . Phytother. Res. , 4 : 235 – 236 .
- Yixiang , H. , Shenghui , Z. , Jianbo , W. , Kang , Y. , Yu , Z. , Lihui , Y. and Laixi , B. 2010 . Matrine Induces Apoptosis of Human Multiple Myeloma Cells via Activation of the Mitochondrial Pathway . Leuk. Lymphoma. , 51 : 1337 – 1346 .