Abstract
Copyright 2012 American Association for Aerosol Research
Superaggregates (SAs) are formed due to late stage aggregation occurring near the gelling point of an aggregating system (Dhaubhadel et al. Citation2006). Percolation theory best describes their formation mechanism (Sorensen and Chakrabarti Citation2011). SAs have a long-range overall structure described by a fractal dimension Df ≈ 2.6 and a short-range local structure described by Df ≈ 1.8 (Sorensen et al. Citation1998; Sorensen and Chakrabarti Citation2011). Recently, based on the mechanism of SA formation in an aggregating system, Dhaubhadel and co-workers (Dhaubhadel et al. Citation2007) carried out enclosed-chamber aerosol aggregation to create “aerosol gels”—a novel material similar in properties to aerogels (Akimov Citation2003; Lerner Citation2004). Flame synthesis—which has been shown to be a cost-effective, scalable, and versatile route for large-scale production of nanoscale materials (Ulrich Citation1984)—is yet to be established as a viable method for production of aerosol gels. An upward-pointing flame system cannot facilitate steady and consistent formation of SAs in its product outflow upstream because of the aggregate fragmentation effects associated with buoyancy-driven flickering and shear stress of the flame's “tipping” front (Shaddix and Smyth Citation1996).
Here, we report our observations of steady outflow of SAs from a nontipping, reversed gravity (-g, up-side-down configuration) laminar methane–air diffusion flame. shows a schematic drawing of our up-side-down diffusion burner that is fed with methane through the inner tube and coflow air through the outer tubes, as in a Burke–Schumann arrangement (Stipe et al. Citation2005; Kirchstetter and Novakov Citation2007). The flame is cylindrical in shape, and the tip of the flame remains open. No flame flickering was observed past a few seconds after ignition. A portion of the exhaust was extracted using an ejector pump through the downstream port. Particle flow exiting the ejector pump was split isokinetically for characterization of particle morphology through two parallel setups—a large-angle static light scattering (SLS) setup (Kim et al. Citation2006), and a scanning electron microscopy (SEM) sampling unit (Chakrabarty et al. Citation2009). Additional information regarding the experimental setup and analysis methods used in this study can be found in the accompanying supplemental files.*
FIG. 1 Left, schematic diagram of the experimental setup used for aggregate generation and characterization. Right, photograph of the up-side-down diffusion flame. (Color figure available online).
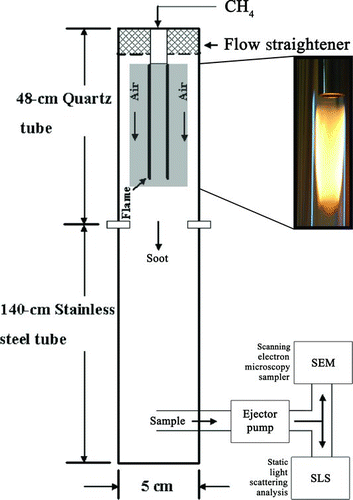
SEM image of a typical SA observed during this study is shown in a. The partial fragmentation of the fragile network SA seen in could be due to the effects of impaction during the sampling process. To confirm the morphology of SAs to be consistent with previously reported observations (Chakrabarty et al. Citation2006; Kim et al. Citation2006), we quantitatively analyzed the SA micrographs using the perimeter method image analysis technique (Dhaubhadel et al. Citation2006; Chakrabarty et al. Citation2011). Analyses were performed on approximately 25 micrographs for investigating SA morphology. Past experimental and simulation studies (Jullien et al. Citation1994; Dhaubhadel et al. Citation2006) have confirmed the perimeter fractal dimension Dp of an SA to be well defined and related to its mass fractal dimension Df using the following empirical relationship:
FIG. 2 (a) SEM image of a typical superaggregate observed in the outflow of the up-side-down diffusion flame used in our study. (b) Perimeter fractal analysis of part (a). The negative perimeter analysis slope equals the perimeter fractal dimension, Dp = 1.4. The mass fractal dimension, Df is found via Equation (1a) to be 2.46.
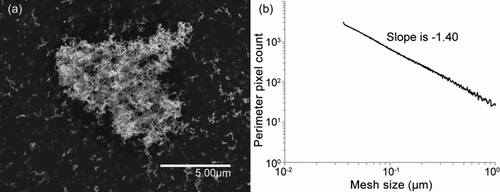
Using the large-angle SLS setup, we quantitatively characterized the morphology of aggregates, which constituted the SAs. Our SLS apparatus facilitated measurements of aggregate morphologies with length scales between 150 nm and 1 μm. Aggregates constituting the repeating units of the SAs were calculated to exhibit Df = 1.82 ± .05 ( a,b). These values confirm the growth mechanism of the aggregates in the dilute regime of the flame system to be consistent with the diffusion limited cluster agglomeration (DLCA) mechanism (Jullien and Botet Citation1987), and its subsequent transition into the percolation regime.
Our observation of superaggregation of DLCA-grown aggregates in an up-side-down flame configuration is explained by the following hypothesis. A “stagnation plane” is formed at the nontipping, lower end of the diffusion flame which confines the DLCA-grown Df ≈ 1.8 aggregates. By enhancing the aggregate residence time within the stagnation plane, aggregates evolve from dilute to percolation regime to form SAs. b depicts a schematic of this hypothesis. When a flame is inverted, the effects of buoyancy on the flow-field are quite drastic. Buoyancy provides an upstream feedback mechanism that balances the opposing flow forces to create a zero-acceleration plane of stagnation downstream from the flame zone (Bédat and Cheng Citation1996). Creation of this plane pushes the flame front wide open and makes it curl outward. This mechanism is contrary to what takes place in a normal gravity upright flame, where buoyant forces accelerate the flow forces, causing a narrowing of the flame front to tip (). For a low-sooting fuel such as methane, a narrowing and accelerating flame front cannot provide the combination of high number density and aggregating time for aggregates to cross over into the percolation regime, while we have shown here that this is possible for the reversed gravity flame. Additionally, buoyant forces in upright flames cause flickering, which results in aggregate structure fragmentation.
FIG. 3 (a) SEM image of a single aggregate. These aggregates constitute the repeating units of the superaggregates observed in this study. (b) Large-angle structure analysis measured an ensemble of the aggregates in part (a) to have a negative slope (Df ) ≈ 1.8, consistent with the DLCA growth mechanism.
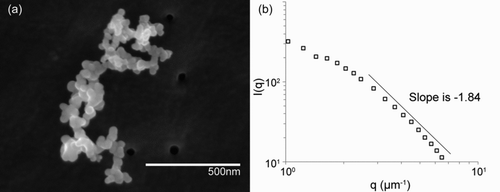
FIG. 4 Schematic depiction of our hypothesis explaining superaggregate formation in an up-side-down diffusion flame system. (a) In an upward-rising diffusion flame system, a narrowing and accelerating flame front cannot provide the threshold aggregating time for DLCA-grown Df ≈ 1.8 aggregates to cross over into the percolation regime. (b) In an up-side-down flame system, a “stagnation plane” is formed at the nontipping end of the flame, which confines the aggregates and their residence time within the plane to facilitate their evolution from dilute to percolation regime to form superaggregates.
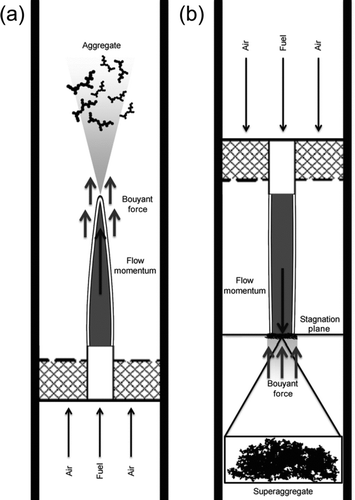
Further investigation of our hypothesis and/or any other mechanism that might be involved in the production of SAs from an inverted diffusion flame is needed. Future research needs to be directed toward characterization and optimization of our inverted flame system using different fuels and operating conditions (e.g., temperature, flow parameters, equivalent ratios), and toward identifying its limitations. In situ aerosol growth analysis, using a small-angle SLS apparatus at different heights in the flame (Beaucage et al. Citation2004), might provide further insights into the growth kinetics of gelation, which is currently an unresolved question (Sorensen and Chakrabarti Citation2011).
uast_a_608389_sup_20738248.zip
Download Zip (21 KB)Acknowledgments
*Supplementary materials are available for this article. Go to the publisher's online edition of Aerosol Science and Technology to view the free supplementary files.
This material is based upon work supported by NASA EPSCoR under Cooperative Agreement No. NNX10AR89A, by NASA ROSES under grant NNX11AB79G and by the Desert Research Institute.
REFERENCES
- Akimov , Y. K. 2003 . Fields of Application of Aerogels (Review) . Instrum. Exp. Tech. , 46 : 287 – 299 .
- Beaucage , G. , Kammler , H. K. , Mueller , R. , Strobel , R. , Agashe , N. , Pratsinis , S. E., and Narayanan , T. 2004 . Probing the Dynamics of Nanoparticle Growth in a Flame Using Synchrotron Radiation . Nat. Mater. , 3 : 370 – 373 .
- Bédat , B. and Cheng , R. K. 1996 . Effects of Buoyancy on Premixed Flame Stabilization . Combust. Flame , 107 : 13 – 26 .
- Chakrabarty , R. K. , Garro , M. A. , Garro , B. A. , Chancellor , S. , Moosmüller , H. and Herald , C. M. 2011 . Simulation of Aggregates with Point-Contacting Monomers in the Cluster-Dilute Regime. Part 1: Determining the Most Reliable Technique for Obtaining Three-Dimensional Fractal Dimension from Two-Dimensional Images . Aerosol Sci. Technol. , 45 : 75 – 80 .
- Chakrabarty , R. K. , Moosmüller , H. , Arnott , W. P. , Garro , M. A. , Tian , G. , Slowik , J. G. , Cross , E. S. , Han , J. H. , Davidovits , P. and Onasch , T. B. 2009 . Low Fractal Dimension Cluster-Dilute Soot Aggregates from a Premixed Flame . Phy. Rev. Lett. , 102 : 235504
- Chakrabarty , R. K. , Moosmüller , H. , Garro , M. A. , Arnott , W. P. , Walker , J. , Susott , R. A. , Babbitt , R. E. , Wold , C. E. , Lincoln , E. N. and Hao , W. M. 2006 . Emissions from the Laboratory Combustion of Wildland Fuels: Particle Morphology and Size . J. Geophys. Res. , 111 : 7204 – 7220 .
- Dhaubhadel , R. , Gerving , C. S. , Chakrabarti , A. and Sorensen , C. M. 2007 . Aerosol Gelation: Synthesis of a Novel, Lightweight, High Specific Surface Area Material . Aerosol Sci. Techol. , 41 : 804 – 810 .
- Dhaubhadel , R. , Pierce , F. , Chakrabarti , A. and Sorensen , C. M. 2006 . Hybrid Superaggregate Morphology as a Result of Aggregation in a Cluster-Dense Aerosol . Phy. Rev. E , 73 : 011404
- Fry , D. , Chakrabarti , A. , Kim , W. and Sorensen , C. 2004 . Structural Crossover in Dense Irreversibly Aggregating Particulate Systems . Phy. Rev. E , 69 : 061401
- Jullien , R. and Botet , R. 1987 . Aggregation and Fractal Aggregates , Singapore : World Scientific .
- Jullien , R. , Thouy , R. and Ehrburger-Dolle , F. 1994 . Numerical Investigation of Two-Dimensional Projections of Random Fractal Aggregates . Phy. Rev. E , 50 : 3878 – 3882 .
- Kim , W. , Sorensen , C. , Fry , D. and Chakrabarti , A. 2006 . Soot Aggregates, Superaggregates and Gel-Like Networks in Laminar Diffusion Flames . J. Aerosol Sci. , 37 : 386 – 401 .
- Kirchstetter , T. W. and Novakov , T. 2007 . Controlled Generation of Black Carbon Particles from a Diffusion Flame and Applications in Evaluating Black Carbon Measurement Methods . Atmos. Environ. , 41 : 1874 – 1888 .
- Lerner , E. J. 2004 . Less Is More with Aerogels . Ind. Physicist , 10 : 26 – 32 .
- Shaddix , C. R. and Smyth , K. C. 1996 . Laser-Induced Incandescence Measurements of Soot Production in Steady and Flickering Methane, Propane, and Ethylene Diffusion Flames . Combust. Flame , 107 : 418 – 452 .
- Sorensen , C. and Chakrabarti , A. 2011 . The Sol to Gel Transition in Irreversible Particulate Systems . Soft Matter , 7 : 2284 – 2296 .
- Sorensen , C. M. , Hageman , B. , Rush , T. J. , Huang , H. and Oh , C. 1998 . Aerogelation in a Flame Soot Aerosol . Phy. Rev. Lett. , 80 : 1782 – 1785 .
- Stipe , C. B. , Higgins , B. S. , Lucas , D. , Koshland , C. P. and Sawyer , R. F. 2005 . Inverted Co-Flow Diffusion Flame for Producing Soot . Rev. Sci. Instrum. , 76 : 023908 – 023908 .
- Ulrich , G. 1984 . Flame Synthesis of Fine Particles . Chem. Eng. News , 62 : 22 – 29 .