Abstract
We have developed a new analyzer for the online measurement of aerosol composition: a particle trap laser desorption mass spectrometer (PT-LDMS). The main components of the instrument include an aerodynamic lens, a particle trap enclosed by a quartz cell, a quadrupole mass spectrometer (QMS), a vacuum chamber incorporating the above components, and a carbon dioxide (CO2) laser (wavelength 10.6 μm). The aerodynamic lens generates a beam of submicron particles, which is focused on a small area on the particle trap. The particle trap consists of custom-made mesh layers, the structure of which was newly designed using engineering techniques for micro electro mechanical systems (MEMS). A large number of mesh frames are well arranged in the trap, and particles can be efficiently captured after multiple impactions on the frames. The CO2 laser is used to vaporize aerosol compounds captured on the particle trap. The evolved gas confined within the quartz cell is analyzed using an electron impact ionization (EI) QMS to quantify the chemical composition of the particles. The concept of the PT-LDMS and first evaluation of its performance are presented, specifically focusing on the structure and performance of the particle trap.
1. INTRODUCTION
Recent advances in online mass spectrometric analyzers have largely improved our understanding of sources and processes of ambient and laboratory-generated aerosols. Analyzer systems include the particle analysis by laser ablation mass spectrometer (PALMS; Murphy and Thomson Citation1995), the aerosol time-of-flight mass spectrometer (ATOFMS; Noble and Prather Citation1996), the single particle laser ablation time-of-flight mass spectrometer (SPLAT; Zelenyuk and Imre Citation2005; Zelenyuk et al. Citation2009a), the two-step laser desorption/ionization mass spectrometer (L2MS; Morrical et al. Citation1998), the thermal desorption particle beam mass spectrometer (TDPBMS; Tobias et al. Citation2000), the Aerodyne aerosol mass spectrometer (AMS; Jayne et al. Citation2000), and the photoionization aerosol mass spectrometer (PIAMS; Öktem et al. Citation2004). These analyzers utilize aerodynamic lenses, which can efficiently deliver an aerosol particle beam into the vacuum chamber. The PALMS, ATOFMS, L2MS, and SPLAT vaporize and ionize in-flight particles by pulsed lasers followed by time-of-flight mass spectrometry (TOFMS). One of the benefits of these instruments is their highly sensitive detection of the elemental composition of single particles, while the hit rate (the ratio of the number of acquired individual particle mass spectra to the number of detected particles) may depend on the particle size (Su et al. Citation2004; Zelenyuk et al. Citation2009b). The TDPBMS, AMS, and PIAMS collect aerosol particles by impaction on solid surfaces and vaporize them using thermal or laser desorption followed by electron impact ionization (EI) or photoionization mass spectrometry. The major benefit of these instruments is the quantitative/semiquantitative analysis of nonrefractory materials. However, the particle collection efficiency is typically less than unity and varies depending on chemical composition (Tobias et al. Citation2000; Matthew et al. Citation2008). This is because liquid particles can be collected at high efficiency, but solid particles tend to bounce off the surface. V-shaped and/or porous surfaces have been used in the TDPBMS and AMS, but bounce still remains a major issue. The bounce of high-velocity particles on a solid surface has been an important issue for impactors (Rao and Whitby Citation1978). Oil or grease is generally used to prevent solid particles from bouncing off the impactor surface, but this is not applicable to the mass spectrometric analyzers listed above. Particle trapping structures without using oil/grease have been proposed for impactors (Biswas and Flagan Citation1988; Chang et al. Citation1999; Mader et al. Citation2001), and these design worked well in some cases. To the best of our knowledge, no studies proposed efficient structures of particle traps that can be used for mass spectrometric analyzers.
We have developed a new analyzer for the online measurement of aerosol composition: a particle trap laser desorption mass spectrometer (PT-LDMS). The major difference compared with preexisting analyzers is a new particle trap that was designed to reduce the loss of particles due to bouncing. The purpose of this article is to describe the principle of the instrument and to evaluate the performance of the particle trap, specifically focusing on the collection efficiency.
2. INSTRUMENT DESIGN
2.1. Hardware Overview
The PT-LDMS has been designed for the online quantification of the masses of sulfate, nitrate, and carbon, which generally contribute major fractions of fine particles. The goal is to collect all particles delivered to the particle trap and also vaporize all compounds containing sulfate, nitrate, and carbon. The PT-LDMS is also intended to be a relatively compact, low-cost instrument in terms of hardware and data processing.
shows a schematic diagram of the instrument. The main components of the PT-LDMS include an inlet assembly (critical orifice, valve, aerodynamic lens, and skimmer), a particle trap enclosed by a quartz cell, a quadrupole mass spectrometer (QMS) with electron impact ionization (EI), and a carbon dioxide (CO2) laser (wavelength 10.6 μm). All of the above components except the CO2 laser are incorporated into a vacuum chamber. The chamber is evacuated using 250 L s−1 turbo molecular pumps (V301, Agilent Technologies, Inc., Santa Clara, CA, USA) with a backup scroll vacuum pump. The vacuum chamber consists of three regions that are differentially pumped. When the sample air is loaded, the pressure in the first chamber (lens and skimmer region) is of the order of 10−3 Torr, the second (particle trap region) is 10−5 Torr, and the third (QMS region) is 10−7 Torr. When the inlet is closed, the pressure in the first chamber is of the order of 10−7 Torr, the second is 10−6 Torr, and the third is 10−8 Torr.
FIG. 1 (a) Schematic diagram of the PT-LDMS. The main components of the PT-LDMS include an inlet assembly, a particle trap enclosed by a quartz cell, a QMS, and a CO2 laser. (b) Structure of the quartz cell and particle trap assembly. One of the exits of the quartz cell (exit 1) is connected to a QMS and the other (exit 2) is connected to the third region of the chamber. See text for details of the components.
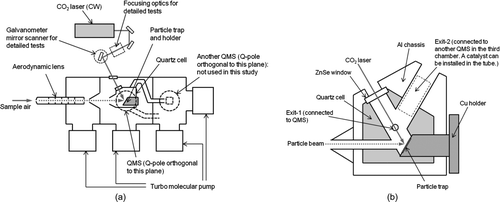
The critical orifice is a 100-μm diameter pinhole, which generates the flow rate of ∼91 cc min−1. The aerodynamic lens produces a well-focused particle beam, which efficiently introduces submicron particles into a small area on the particle trap through the entrance hole of the cell (5 mm diameter). The particle trap consists of custom-made mesh layers (silicon [Si] coated by platinum [Pt]) and a copper (Cu) holder. The structure of the mesh layers was newly designed based on a simplified theoretical calculation to reduce particle loss due to bouncing. Details of the structure of the particle trap are described in section 2.3.
An air-cooled CW CO2 laser with a nominal maximum output power of 10 W (ULR-10, Universal Laser Systems, Inc., Scottsdale, AZ, USA) is used to vaporize the aerosol particles collected on the particle trap. Note that the vaporization process includes not only evaporation and sublimation but also decomposition and reaction. The laser beam irradiates approximately the center of the particle trap through zinc selenide (ZnSe) windows on the walls of the chamber and cell. The laser power was measured by a thermopile sensor (20C-A, Ophir Optronics Ltd., Jerusalem Israel) in front of the ZnSe window on the chamber wall. The laser optics outside the chamber consists of gold (Au)-coated mirrors as the default configuration and a galvanometer mirror scanner (VM500Plus, GSI Group, Inc., Bedford, MA, USA) and ZnSe focusing lens for laboratory tests. The galvanometer mirror scanner consists of electrically driven, two-axis Au-coated mirrors, which enable the systematic change of laser beam position. Without the focusing lens, the beam size (full width at e −2) on the trap is ∼5 mm, which is nearly the same size as the mesh area.
The quartz cell is used to confine the evolved gas within a small volume. Because the mean free path of gas molecules is much longer than the dimensions of the cell, the evolved gas molecules can diffuse in the cell immediately after vaporization. The temperature of the quartz cell (T cell) can be controlled by heating the aluminum chassis enclosing the cell (up to 400°C) to minimize adsorption of the evolved gas on the inner wall of the cell. On the other hand, the temperature of the particle trap (T trap) can be kept lower than T cell using a cooling module. A lower T trap (0°C–10°C) is preferred when sampling semivolatile compounds. The Cu holder for the particle trap is connected to a Cu rod, the other side of which can be cooled by various ways including a Peltier module, water, and liquid nitrogen.
One of the exits of the quartz cell (exit 1) is connected to a QMS (QMS-1, gas-tight ionizer type, PrismaPlus, Pfeiffer Vacuum, GmbH, Asslar, Germany) to quantify the mass concentrations of sulfate and nitrate. The ion detector is a secondary electron multiplier operated at ∼1 kV. The benefit of using the gas-tight ionizer is that it allows relatively high pressures in the ionizer. The conductance of the gas-tight ionizer is only 1 L s−1, and a small fraction of the evolved gas is introduced into the ionizer. The housing for the quadrupole and detector is connected to the third region of the vacuum chamber to maintain the pressure of 10−7 Torr when the sample air is loaded. The other exit of the quartz cell (exit 2) is also connected to the third region of the chamber. A catalyst to convert carbonaceous compounds to CO2 can be installed inside the tube from exit 2, and the processed gas is analyzed by another QMS in the third region of the chamber (QMS-2, cross-beam ionizer type, PrismaPlus, Pfeiffer Vacuum, GmbH, Germany) to quantify the mass concentration of carbon. Not only organic carbon (OC) but also elemental carbon (EC) could be catalytically converted to CO2 depending on the laser power. Although the QMS-2 channel is still under development, the current system is sufficient for demonstrating the proof of concept of the particle trap, which is the major focus of this article.
2.2. Detection Procedure
The collection, vaporization, and detection processes are either continuous or semicontinuous, depending on the aerosol concentration. The data acquisition procedure for the semicontinuous mode is illustrated in . In the semicontinuous mode, the time for accumulating particles on the particle trap is nominally 3–10 min for typical ambient conditions. A longer accumulation time is also possible if the concentrations are very low. Ion signals at some selected mass-to-charge ratios (m/z), im /z , are monitored. For QMS-1, m/z 30 (NO+), 46 (NO2 +), 48 (SO+), and 64 (SO+ 2) are selected for the quantification of nitrate and sulfate. Air signals such as m/z 14 (N+) and 32 (O+ 2) are also monitored to account for temporal (slow) drifts of the ion detection efficiency. Details of the correction are described later in this section. As for QMS-2, m/z 14 (N+), 32 (O+ 2), 44 (CO+ 2), and 57 (C4H+ 9) will be selected for the quantification of carbonaceous compounds. The m/z 57 signal may be used as an indicator of the conversion efficiency to CO2. Although TOFMS can provide more comprehensive mass spectra of the evolved gas, QMS is sufficient for monitoring the limited number of m/z signals listed above (the instrument is intended as a relatively compact, low-cost instrument in terms of hardware and data processing, as mentioned previously). Hereafter, QMS-1 is referred to as QMS for simplicity.
FIG. 2 Data acquisition procedure for the semicontinuous mode. The time for accumulating particles on the particle trap is nominally 3–10 min for typical ambient conditions. Ion signals at some selected mass-to-charge ratios (m/z), im /z , are monitored during the laser desorption.
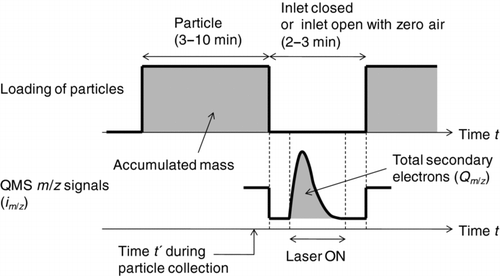
In the temporal evolution of the ion signals, the signal above the background level is integrated to obtain total secondary electrons detected during laser desorption (Qm /z ). The benefits of using Qm /z instead of the peak height of im /z are that the result does not depend on the difference in the time evolution profile of im/z and that a better signal-to-noise (S/N) ratio can be achieved by integrating the signals. The ratio of Qm /z to the accumulated mass represents the instrument sensitivity, Sm /z (pC ng−1). The evolution should take place as fast as possible to concentrate the signal within a short time, while evolution that is too fast is not favorable for the detection by QMS. This point will be discussed in section 5.
The ion signals are obtained under either closed or open inlet conditions. While an open inlet is required for the continuous detection mode, the closed inlet yields a better S/N ratio due to reduced contributions of gas-phase compounds and is also beneficial to the reduction of time exposed to high-intensity signals from air molecules, which may help to slow down the degradation of the QMS detector.
The correction for temporal drift of the ion detection efficiency is given by
2.3. Particle Trap
illustrates the structure of the particle trap. It consists of five mesh layers and a plate at the bottom. The mesh layers were designed and machined using engineering techniques for micro electro mechanical systems (MEMS), which are commonly used to produce microstructure semiconductor devices. To the best of our knowledge, this study is the first to apply the MEMS techniques to produce a particle trap substrate. The MEMS techniques allow us a variety of designs (shape, size of the opening, width and depth of the frame, etc.). In the current configuration, the mesh pattern is a square grid: the side length of the square opening (a) is ∼100 μm, the frame width (r) is ∼5 μm, and the depth (d) is ∼50 μm. Here, we refer to the 5-μm width section as the frame edge and the 50-μm depth section as the frame wall. The distance between the mesh layers is ∼150 μm, resulting in a total depth of ∼1 mm from the top to the bottom of the layers. The area of the mesh pattern (5-mm-diameter circle) is sufficiently large compared to the broadening of the particle beam (∼1 mm).
FIG. 3 Structure of the particle trap. The trap consists of five mesh layers and a plate at the bottom. The mesh pattern is a square grid: the side length of the square opening (a) is ∼100 μm, the frame width (r) is ∼5 μm, and the depth (d) is ∼50 μm. The distance between the mesh layers is ∼150 μm, resulting in the total depth of ∼1 mm from the top to the bottom of the layers. The area of the mesh pattern (5-mm-diameter circle) is sufficiently large compared with the broadening of the particle beam (∼1 mm).
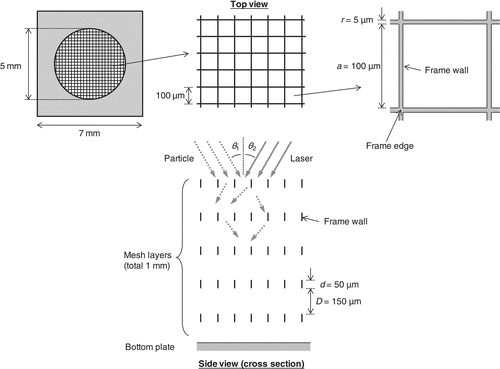
The machining process is briefly described. The main body of the frame and plate is made of Si and can be coated with various metals. In the current configuration, Pt has been chosen as the coating material because of its catalytic properties and corrosion resistance. The coating thickness is set at ∼0.1 μm, but the actual thickness could vary between the frame edge and wall. The particle trap can be heated upon laser irradiation because of the nonunity reflectance of Pt at the wavelength of 10.6 μm (∼0.9 for bulk Pt surface). The mesh patterns are drawn on a Si wafer, coated with Pt, and cut into individual pieces. There could be a few “void grids” due to some physical damage during the machining, but this does not affect the overall performance of the trap considering the large number of grids on a mesh plane. The mesh layers are attached to the trap holder together with the bottom plate. The bottom plate is made of either pure Pt or Pt-coated Si (the former is used in the current configuration). The patterns of the five mesh layers do not completely overlap, which is not very critical.
The mesh layers can be characterized by a large areal porosity from the direction perpendicular to the mesh plane, where the mesh plane is defined as the top surface of the mesh layer. For the square mesh pattern, the areal porosity (p) is defined as
The current configuration (a = 100 μm and r = 5 μm) yields p = 0.91.
The mechanism of the particle trapping is significantly different from traditional particle filters because only straight motion between impactions needs to be considered under high-vacuum conditions. Liquid particles are very likely collected by either the frame or the bottom plate upon a single impaction. The motion of solid particles can be modeled as follows. Let us define the particle beam angle θ 1 and laser beam angle θ 2 as those relative to the direction perpendicular to the mesh plane, respectively. Some particles may go through the first mesh layer without any impaction and the others will collide on the frame. Particles hitting the frame edge of the first mesh layer likely bounce and escape from the mesh layer (i.e., not collected). Particles hitting the frame wall also likely bounce but go through the mesh layer. Considering the large number of mesh frames in the trap, we can assume that particles would lose their kinetic energy after several impactions and eventually be captured by either the frame or the bottom plate. A computational hydrodynamics simulation shows that solid particles can lose a significant portion of their kinetic energy upon impaction, depending on the physical properties of the material. Details of the simulation will be presented elsewhere. An additional benefit of the high-porosity structure of the particle trap is that the laser beam can efficiently irradiate almost all sections of the particle trap by reflection or diffraction of the laser beam. In fact, we can observe the “footprint” of some materials burned by the laser beam on the bottom plate when the plate is not well cleaned.
Based on the geometry of the mesh frame and wall, we conducted a simple calculation to estimate transmission and wall-impaction probabilities of particles with respect to the first mesh layer, Tr and Wi, as follows.
For a > d tan θ 1,
Otherwise
The value of Tr + Wi (= p) represents the overall particle collection efficiency if all the particles passing through the first mesh layer are eventually collected somewhere in the mesh layers or bottom plate. The fraction of particles collected on the mesh layers rather than the bottom plate increases with increasing θ 1. This is preferred to minimize particles that escape from the mesh layers after impaction and bounce from the bottom plate. On the other hand, increasing θ 1 reduces the effective width of the particle trap from the viewpoint of the particle beam. This is more clearly illustrated in , which shows the probability of wall impaction (1 – Tr 5) and the effective width of the mesh plane (5cosθ 1) as a function of the particle beam angle θ 1. Here, we assume a random overlap of the five mesh patterns rather than a complete overlap (the latter is practically difficult). The θ 1 value is set at 30° considering the trade-offs of the two variables.
FIG. 4 Probability of wall impaction (solid) and the effective width of the mesh plane (shaded) as a function of the particle beam angle θ 1. The probability of wall impaction was calculated as 1 – Tr 5 using Equations (Equation2) and (Equation3) and the effective width as 5cosθ 1.
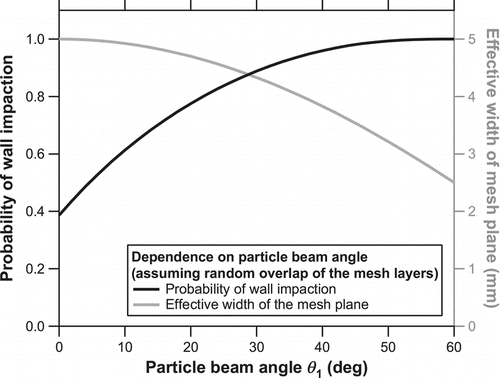
The determination of the laser beam angle is somewhat complicated because the laser beam is quite likely reflected and/or diffracted by the frame. We have chosen θ 2 = 30° rather arbitrarily in the current configuration, which is limited by the geometry of the vacuum chamber.
We should note that the value of 1 – p (= 0.09) is the upper limit of the particle fraction that may bounce on the frame edge of the first mesh layer. Some of the bounced particles escape from the quartz cell and are pumped away, while the others deposit on the wall of the cell. Some compounds in the deposited particles may be vaporized depending on T cell and the dissociation temperatures of the compounds. This issue will be discussed in section 4.4.1.
2.4. Advantages of the Instrument Design
The configuration of the PT-LDMS is a hybrid of thermal desorption/electron impact ionization instruments (e.g., TDPBMS and AMS) and laser desorption/ionization instruments (e.g., L2MS, SPLAT, and PIAMS). It is worthwhile summarizing the scientific basis of the design to clarify the advantages of this instrument.
The benefit of using a CO2 laser instead of thermal desorption is that the former can potentially vaporize all aerosol compounds containing sulfate, nitrate, and carbon within a short time (of the order of seconds). CO2 lasers have been used in some L2MS instruments (Morrical et al. Citation1998; Woods et al. Citation2002). The purpose of using CO2 lasers in these L2MS instruments is the soft vaporization of organic compounds in a single particle. A pulsed CO2 laser with very high power density (106–107 W cm−2) is necessary for this purpose because the duration time of the laser irradiation with respect to a single particle is of the order of μs. In the PT-LDMS, particles collected on the trap are continuously heated by the CW CO2 laser, and the particle compounds can be vaporized with a much lower power density. The vaporization mechanism includes both direct heating of aerosol particles due to light absorption and thermal conduction from the heated surface of the particle trap. Note that the relative contributions of these processes depend on the laser power, surface properties of the trap, and thermodynamic properties of aerosol compounds. This issue has not been optimized for multiple compounds at the present stage, as discussed in section 5.
Apart from the new particle trap, one of the major differences between this instrument and preexisting ones is the use of a quartz cell. While the use of the quartz cell dilutes the evolved gas before introducing it to the ionization region, thus reducing the efficiency of producing ions per unit mass of the accumulated particles (i.e., sensitivity), it can immediately mix the evolved gas well with air molecules, which makes it possible to account for temporal drifts of ion detection efficiencies by simply using the air signals (section 2.2). Heating the quartz cell and cooling the trap may introduce some complications in the hardware, but the use of the quartz cell is critical for the quantification of carbon mass. Conversion of carbonaceous compounds to CO2 can largely simplify the quantification and thus improve the accuracy.
3. APPARATUS FOR THE LABORATORY TESTS
3.1. Particle Generation System
shows the particle generation system and oil coating apparatus. The particle generation system is basically the same as that described in Takegawa et al. (Citation2005), and the oil coating apparatus is similar to the one used in Moteki and Kondo (Citation2007). The particle generation and evaluation system consists of a Collison atomizer (Model 3076, TSI, Inc., Shoreview, MN, USA), a diffusion dryer, a differential mobility analyzer (DMA, Model 3081, TSI, Inc., USA), and a condensation particle counter (CPC, Model 3022, TSI, Inc., USA). Monodisperse aerosols with known chemical compositions were generated and introduced into the PT-LDMS. Potassium nitrate (KNO3) was chosen for the evaluation of the particle trap because the interpretation is simple. As described in section 4.3.4, the evolved gas from KNO3 is almost exclusively nitric oxide (NO). Moderately high melting and dissociation temperatures (333°C and 400°C, respectively) prevent the particles from evaporating during the sampling. Ammonium sulfate [(NH4)2SO4] was also used as an example of the detection of other compounds.
The oil (oleic acid) coating apparatus was used for estimating the particle collection efficiency. The KNO3 seed particles were introduced into an oil bath heated at 90°C, followed by a cooling section to condense oleic acid vapors onto the seed particles. The coated particles were then classified by another DMA (Model 3081) to select particles with known coating thickness. We confirmed that no particles were found after the second DMA without KNO3 seed particles, indicating that homogeneous nucleation of oleic acid did not yield particles in the size range that was selected by the second DMA. Note that the particle number concentrations decreased substantially through the second DMA because the size distribution of the oil-coated particles was rather broad. The sample flow rate through the DMA was 0.4 L min−1 (the sum of the flow rates of the PT-LDMS and CPC). The sheath flow rates were 4.0 and 3.0 L min−1 for the first and second DMA, respectively. Estimates of multiply charged particles were made using the same method as presented by Wang et al. (Citation2010) and Takegawa and Sakurai (Citation2011). The reason for the reduced sheath flow rate of the second DMA is that the corrections for multiply charged particles need to be done by doubling the high voltage without changing the sheath flow rate. The effect of the slight broadening of the transfer function of the second DMA is minor.
3.2. Overview of the Laboratory Experiments
Three types of experiments were performed for testing the performance of the particle trap. The first experiment was the direct observation of collected particles using a scanning electron microscope (SEM). A simple test chamber incorporating an inlet assembly (a critical orifice, valve, aerodynamic lens, and skimmer) and a particle trap was constructed for evaluating the performance of the particle trap. This test chamber did not include other components (a quartz cell, CO2 laser optics, and QMS) and had more flexibility than the PT-LDMS with regard to this specific purpose. KNO3 particles with or without oil coating were used for this experiment. The selected diameters of solid KNO3 particles were dm = 400 nm, and those of oil-coated KNO3 particles were dm = 250 nm for the core and dm = 450 nm for the shell. The core diameter refers to that selected by the first DMA and the shell diameter is that selected by the second DMA. The number concentration and accumulation time were 3 × 103 cm−3 and 10 min, respectively, for solid KNO3 particles, and 4 × 102 cm−3 and 30 min, respectively, for oil-coated particles.
The second experiment was the preliminary tests using the PT-LDMS with a simple optical system (i.e., without the galvanometer mirror scanner or focusing lens). KNO3 particles with or without oil coating were used for the experiment. The selected diameters of solid KNO3 particles were dm = 250 nm, and those of oil-coated KNO3 particles were dm = 250 nm for the core and dm = 450 nm for the shell. The mass concentrations of KNO3 particles in the sample air ranged from 0 to ∼40 μg m−3 (as NO− 3). The accumulation time for the particle collection was 5–30 min. Note that the QMS sensitivity was not sufficient because the QMS ionizer and detector degraded after repeated evacuation and venting under various operating conditions. Before starting the experiments, the position of the aerodynamic lens was adjusted by collecting particles onto a greased target placed at the entrance of the quartz cell. The position of the laser beam was coarsely adjusted by placing a small piece of quartz filter in the trap holder and observing the red-hot spot upon laser irradiation. Further alignment of the laser beam position was performed by manually moving the mirrors while monitoring the ion signals originating from collected particles. The laser irradiation and data acquisition of ion signals were performed with the inlet open.
The third experiment was performed with the galvanometer mirror scanner, focusing lens, and new QMS installed. The particle trap was also replaced with a new one. KNO3 particles were mostly used for the experiment. The selected diameters of solid KNO3 particles were dm = 250 nm, and those of oil-coated KNO3 particles were dm = 250 nm for the core and dm = 450 nm for the shell. The mass concentrations of KNO3 particles in the sample air ranged from 0 to ∼40 μg m−3 (as NO− 3). The accumulation time for the particle collection was typically ∼3 min. (NH4)2SO4 particles with a mobility diameter of 250 nm were also used as another example. Adjustment of the aerodynamic lens and laser position was performed in the same way as the preliminary test. The major difference was that the mirror scanner enabled us to investigate systematically the dependence of the vaporization efficiency on the laser power and position. The focusing lens was temporarily used to examine how the difference in the laser power density affects the detection. As described in section 4.3, the focusing lens did not yield a significant improvement, and thus, the lens was not actually used for the experiments. The laser irradiation and data acquisition of ion signals were conducted with the inlet closed.
The temperature controllers for the quartz cell and the particle trap were not operated during most of the experiments. The T trap was close to room temperature (20°C–25°C), which does not affect the quantification of KNO3 because it is nonvolatile at this temperature. The T cell (40°C–44°C) was somewhat higher than room temperature because the laser beam reflected by the trap gently heated the quartz cell. We assume that adsorption of NO on the quartz cell was not significant under the above T cell conditions. This assumption was tested by altering T cell, as discussed in section 4.4.1.
We measured all possible m/z peaks for KNO3 particles and confirmed that the signals from NO− 3 were dominated by m/z 30. It is rather difficult to obtain full mass spectra of the evolved gas because the duty cycle of the QMS was not sufficiently fast compared with the evolution rate of the signals. Instead, the contributions of the other fragments were obtained separately. Details of the other fragments are presented in section 4.3.4. For most of the following experiments, only m/z 14 (N+), 30 (NO+), and 32 (O+ 2) signals were monitored. The data were acquired every 60 ms, and the dwell times at m/z 14, 30, and 32 were 5, 20, and 5 ms, respectively. A shorter data acquisition interval (10 ms) and dwell time (2 ms) did not show a significant difference.
4. RESULTS
4.1. Microscopic Images of Trapped Particles
Monodisperse, solid (dry) KNO3 aerosols with dm = 400 nm were introduced into the test chamber, and microscopic images of mesh layers were obtained using a moderate-resolution SEM (VE-9800, Keyence, Inc., Osaka, Japan). –d show SEM images of the third layer near the center of the particle beam and ∼1.5 mm away from the center. It appears that particles were mostly collected on the second and third mesh layers and rarely on the bottom plate. The actual frame width was somewhat narrower than that designed (5 μm) because of limitations in the machining. It should be noted that particles were uniformly deposited on both sides of the frame wall, although the particle beam was introduced from a specific side (). This suggests significant bounce of particles (presumably a few times) before deposition. Some particles were hemispherical in shape, indicating deformation upon impaction. Very few particles were found >1.5 mm away from the center. We qualitatively (visually) inspected the horizontal distribution of trapped particles and found that most of the particles were confined within a small area (∼2 mm in vertical and ∼3 mm in horizontal). This indicates that particles were efficiently trapped within a small cylindrical volume of 2–3 mm diameter and ∼1 mm height. It is interesting to note that very few particles coincided together on the trap, even though the particle loadings were quite high. This is favorable to avoid resuspension of trapped particles by collision with newly introduced particles.
FIG. 6 SEM images of the third layer with 400-nm KNO3 particles loaded: (a) near the center of the particle beam viewed from a 10° angle; (b) same as (a) but a closer view; (c) near the center of the particle beam viewed from a 0° angle; (d) ∼1.5 mm away from the center viewed from a 0° angle. SEM images of the mesh layer with oil-coated KNO3 particles loaded (core 250 nm, shell 450 nm): (e) first mesh layer and (f) third mesh layer viewed from the particle beam side.
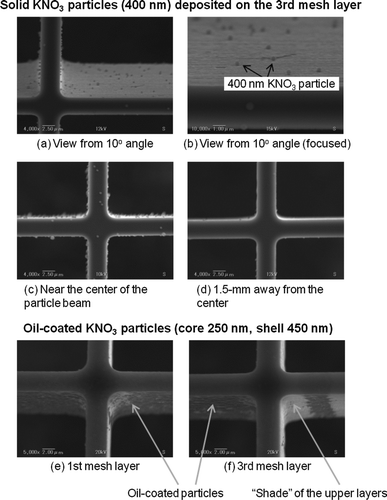
We also obtained SEM images of thickly oil-coated KNO3 particles deposited on the first and third layers ( and f). The core and shell diameters were dm = 250 and 450 nm, respectively. It appeared that particles were mostly collected from the first to third mesh layers. Very few particles deposited on the bottom plate. The oil-coated particles were found deposited specifically on the side of the frame wall facing the particle beam and the area of the particle deposition seemed smaller than the case for solid particles (∼0.2 mm in vertical and ∼0.3 mm in horizontal). Interestingly, we found a nonuniform distribution of particles on the third layer, presumably due to the “shade” of the upper layers. These results support the commonly accepted assumption that liquid particles or solid particles thickly coated by liquid do not bounce upon impaction.
4.2. Preliminary Test
In the preliminary test, the evolution of ion signals upon laser irradiation, the relationship between ion signals and mass loadings, the particle collection efficiency, and the effects of T cell and dm on the detection were evaluated using laboratory-generated KNO3 particles to obtain proof of concept. The results were mostly identical to the detailed tests described in the following sections and are not presented here, to avoid redundancy. The major difference is the evolution profiles of the ion signals. This is potentially important for future improvements and is thus presented here in detail.
and b show the temporal evolution of the ion signal (im /z ) at m/z 30 (NO+) for solid KNO3 particles (dm = 250 nm) and oil-coated KNO3 particles (core: dm = 250 nm, shell: dm = 450 nm), respectively. The accumulated mass of 10 ng corresponds to an NO3 mass concentration of ∼20 μg m−3 for an integration time of 5 min. The laser irradiated the particle trap for 6 s. The laser power irradiating the trap was estimated to be 3.7 W considering the transmittance of the two ZnSe windows. The average power density was estimated to be 17 and 32 W cm−2 within the beam size at e −2 and half maximum, respectively. No systematic tests were made at lower laser power conditions. A large enhancement of the m/z 30 signal above its background level was observed immediately after the laser was turned on. The background level of m/z 30 was estimated by linearly interpolating the signals before and after laser irradiation. A sharp increase in the ion signals was found within 1 s for both solid and oil-coated KNO3 particles. There was a broad peak in the ion signal during the latter stage of the laser irradiation (between 1 and 6 s), although it is somewhat difficult to distinguish the peak from the noise level. For both cases, further irradiation of the laser (additional 6 s) did not generate detectable signals above the background level.
FIG. 7 Temporal evolution of i 30 for (a) solid KNO3 particles (dm = 250 nm) and (b) oil-coated KNO3 particles (core: dm = 250 nm, shell: dm = 450 nm) at the laser power of 3.7 W obtained during the preliminary test. The laser was turned on at time = 0 and irradiated the particle trap for 6 s. The dashed line represents the background level. (c) Temporal evolution of i 30 when particle-free air (zero air) was introduced to the instrument.
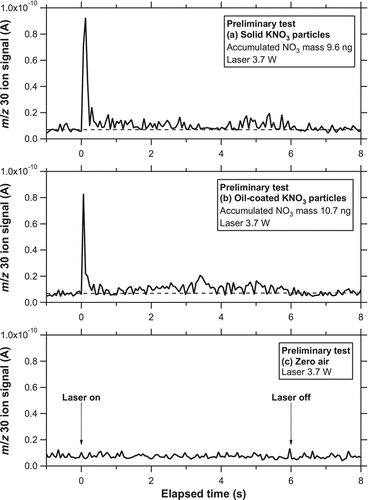
shows the temporal evolution of i 30 when particle-free air was introduced to the instrument (hereafter referred to as zero-air test) for 5 min. The zero-air tests were repeated several times after the loadings of KNO3 particles, and no significant change in the ion signal was observed, indicating that artifacts due to the laser desorption process were below the noise level under these conditions.
4.3. Detailed Tests
In order to investigate the dependence of the ion signals on various parameters including the laser power, beam diameter, and position, we modified the optical system and also replaced the QMS with a new one, as mentioned previously. Unfortunately, the particle trap used for the preliminary test was penetrated by a laser beam (∼1 mm holes in the center of the five mesh layers) when the laser power density was increased by a factor of >2 using the focusing lens. Considering the melting point of Si and Pt (1420°C and 1769°C, respectively), it is estimated that the maximum temperature of the trap irradiated by the center of the laser beam reached >1400°C. We removed the focusing lens from the system and replaced the particle trap with a new one.
4.3.1. Vaporization Efficiency and Linearity
The choice of laser power is an important issue because it is a trade-off for the sensitivity, measurement artifacts, and risk of damage. It is difficult to measure the temperature of the trap irradiated by the laser precisely in the current configuration. We gradually increased the laser power and visually observed the trap, and found a red-hot spot (diameter ∼1 mm) near the center of the trap when the power exceeded ∼3 W. In general, red-hot spots can be visually recognized when the temperature of a material reaches 500°C–1000°C. Although this is a qualitative estimate, it is quite likely that the temperature of the red-hot spot exceeded the dissociation temperature of KNO3 (400°C). This suggests that the vaporization efficiency was ∼100% at least near the center of the laser beam.
shows the temporal evolutions of the ion signals at m/z 30 after solid KNO3 particles (dm = 250 nm) were collected for 3.3 min (NO− 3 mass concentration of ∼30 μg m−3). The actual time for the open inlet was ∼4.5 min including the transition between zero air and particle-loaded air. The laser irradiated the center position with powers of 2.3 and 3.9 W for 1 min. As for the laser power of 3.9 W, the average power density is estimated to be 18 and 33 W cm−2 within the beam size at e −2 and half maximum, respectively. The evolution tended to take place faster with increasing laser power. There were several peaks in the evolution profiles. The appearance of these peaks was reproducible when the mass loadings and laser irradiation procedure were repeated. It appears that the laser duration time of 1 min was sufficient for 3.9 W because no significant signal was observed after 30 s. However, it was not sufficient for 2.3 W considering that the signal still remained even at 1 min. In , the temporal evolutions of the ion signals for the zero-air test at 2.3 and 3.9 W are expanded. The duration time for zero-air sampling was set at 4.5 min to match the time for the particle loadings. We found a small but nonnegligible artifact when the laser was turned on. As for the data obtained at 2.3 W, a substantial fraction of the artifact was due to leftover KNO3 particles from previous measurements. As for the data at 3.9 W, however, the artifact was not due to leftover KNO3 particles from previous measurements. This point will be discussed below in association with the vaporization efficiency of KNO3.
FIG. 8 (a) Temporal evolution of i 30 for solid KNO3 particles (dm = 250 nm) in the modified system. The laser power was 2.3 (shaded) and 3.9 W (solid). The zero-air signal at 3.9 W is also shown with a dashed line. Note that the evolution of the signal was much broader than that in . (b) Temporal evolution of i 30 for zero air at a laser power of 2.3 (shaded) and 3.9 W (solid).
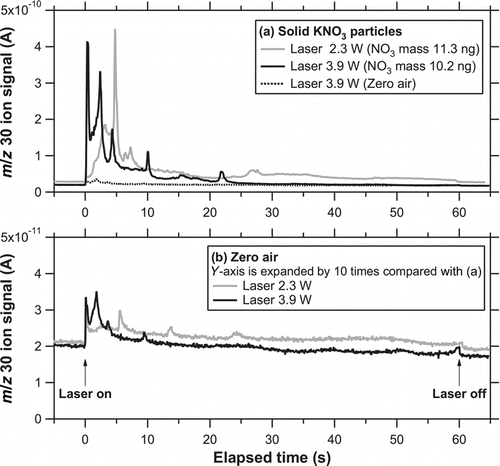
We did not increase the laser power above 3.9 W, to reduce the risk of damaging the trap due to overheating. Instead, we estimated the vaporization efficiency by scanning the laser beam across the trap (). After KNO3 particles were collected on the trap (∼10 ng), the laser irradiated the center of the particle trap for 1 min. Then, the laser was moved to a position 1 mm upward from the center and irradiated the new position for 1 min. The same procedures were repeated at positions 1-mm down, right, and left. No significant signals were observed at all positions, suggesting that the remaining fraction of KNO3 particles was negligible and that the vaporization efficiency can be considered as nearly 100% at a laser power of 3.9 W.
FIG. 9 Schematic drawing of the laser beam scanning across the particle trap (viewed from the laser beam direction). The dashed circle represents the approximate distribution of deposited particles based on the SEM observations. The shaded circles represent the laser beam size at the e −2 and half maximum.
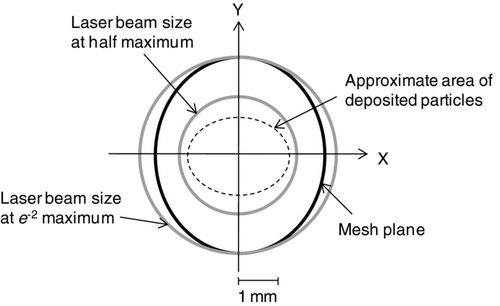
FIG. 10 Temporal evolutions of i 30 for solid KNO3 particles with respect to the different laser positions (): center (dashed), 1-mm up (shaded), and 1-mm left (solid) positions.
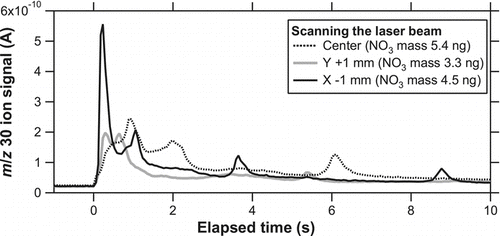
FIG. 11 Correlation plots of total secondary electrons at m/z 30, Q 30, versus the accumulated mass of NO− 3 for 250-nm solid KNO3 particles at different laser powers (2.3, 3.0, 3.4, and 3.9 W). The line represents the regression for a laser power of 3.9 W. The data points obtained at a laser power of 3.9 W with the off-center positions are also shown as triangles.
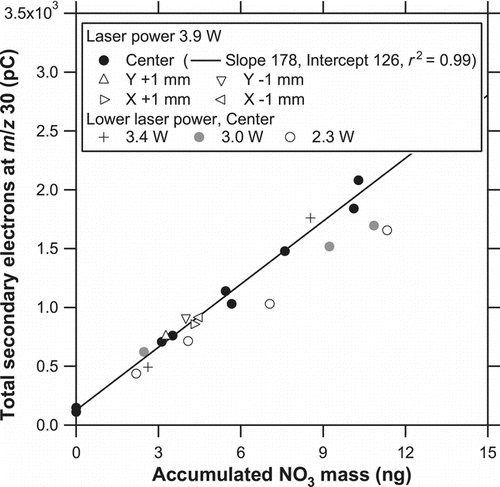
We also investigated the dependence of the vaporization efficiency on the changes in the laser position. This is potentially important for ambient measurements because the distribution of particles on the trap may be slightly different depending on the aerodynamic properties of particles (diameter, density, and shape). After KNO3 particles were collected on the trap (3–6 ng), the laser irradiated the center position for 1 min at a laser power of 3.9 W. The same procedures were repeated at positions 1-mm up, down, right, and left. shows the evolution of the signals obtained at the 1-mm up and left positions, together with that at the center position. The X-axis scale has been expanded compared with for clarity. The shape of the evolution profile showed notable changes with the changes in the laser position. The timing of the peak appearance also changed. These results suggest that the amplitude and timing of the peaks depend on the relative positions of the laser and particles as well as the laser power. Nevertheless, the calculated sensitivity, S 30, at the off-center positions agreed well with that at the center position to within 10% (as quantitatively shown in ). Although the 1-mm left position yielded faster evolution, we have chosen the center position as the default in the following experiments because the slightly slower evolution was more favorable for the detection by QMS.
The dependence of the vaporization efficiency on the laser position and power is summarized here. The data obtained at a laser power of 3.9 W at the center position are used as a reference. shows correlation plots of Q 30 versus the accumulated mass of NO− 3 for solid KNO3 particles at a laser power of 3.9 W with different laser positions and those at the center position with lower laser powers (2.3, 3.0, and 3.4 W). The ion signal was integrated for 1 min to obtain the Q 30 value. Good linearity was found between the detected ions and mass loadings (r 2 = 0.99) for the reference data. The data points at 3.9 W with the off-center positions and those at 3.4 W overlapped the regression line (within the scatter in the data), but those at 2.3 and 3.0 W tended to fall below the regression line. Reproducibility and short-term fluctuation of the laser output power as measured by the thermopile sensor were found to be 2% and 5% (1σ), respectively, at a laser power of 3.9 W. These numbers were much smaller than the difference between 3.9 and 3.4 W. These results suggest that the vaporization efficiency for KNO3 particles would not vary significantly with the possible variability of the laser power or small misalignment of the laser position, at least for a laser power of 3.9 W.
The S 30 value (regression slope) was 178 pC ng−1, in . The number of secondary electrons per NO molecule vaporized is then calculated to be of the order of 10−4. If we assume a multiplier gain of 105, the ionization efficiency (the number of ions per NO molecule vaporized) is estimated to be of the order of 10−9. This number is relatively low because the evolved gas was diluted in the cell, and a substantial fraction of the gas was pumped away from exit 2. While this may be improved by changing the diameter of exit 2, we will retain the cell geometry for the quantification of carbon mass in the future.
4.3.2. Possible Origins of the Artifact
The zero-air test and mapping across the particle trap suggest that the artifact was evident only when zero air was “accumulated” for a certain time. The artifact levels increased with the accumulation time and appeared to be saturated for an accumulation time of more than ∼10 min (not shown). We speculate that dissociative adsorption of O2 molecules on Pt surfaces may have caused catalytic oxidation of a small amount of N2 to form NO upon laser heating. Depletions of N2 and O2 were not observed probably because the reacted fractions of N2 and O2 were very minor compared with their total amounts. Although this issue should be resolved in the future, it is not very critical given the major scope of this article as long as the signal levels for the evaluation are well above the fluctuation of the artifact.
The limit of detection (LOD) for KNO3 is now estimated. Note that this is only a nominal estimate because the LOD may depend significantly on the instrumental conditions. Apart from the artifact, the LOD estimated from the background fluctuation (2σ) prior to the laser irradiation and initial peak height of the evolution of the signal is ∼0.01 ng, which corresponds to an NO− 3 mass concentration of ∼0.01 μg m−3 for an accumulation time of 10 min. This is the best LOD that can be achieved in the current detection system. In order to see the effect of the artifact on LOD, the zero-air test (an accumulation time of 4.5 min) was repeated 8 times, and the average ±2σ was found to be 0.6 ± 0.3 ng (as NO− 3). The fluctuation of 0.3 ng, which corresponds to ∼0.3 μg m−3 for an accumulation time of 10 min, is the nominal LOD for KNO3 particles under the current conditions of the artifact. This LOD value is lower than the nitrate mass concentration of 0.4–8.7 μg m−3 observed in Tokyo (Takegawa et al. Citation2006). For comparison, Ng et al. (Citation2011) reported that the detection limit (3σ) of an Aerodyne Aerosol Chemical Speciation Monitor (ACSM) was 0.024 μg m−3 (0.016 μg m−3 as 2σ) for nitrate at an integration time of 30 min. The LOD of our instrument would be largely improved if optimal conditions for the metal coating of the trap could be identified.
4.3.3. Particle Collection Efficiency
The collection efficiency of the particle trap was evaluated by comparing the evolved gas signals between solid (dm = 250 nm) and oil-coated KNO3 (core diameter: dm = 250 nm, shell diameter: dm = 450 nm) particles. We can estimate the collection efficiency for solid KNO3 particles by assuming that thickly oil-coated KNO3 particles were collected with 100% efficiency. The mobility diameters of solid and oil-coated particles correspond to vacuum aerodynamic diameters (dva ) of 525 and 496 nm, respectively (DeCarlo et al. Citation2004), considering the difference in the density between KNO3 and oleic acid. Because the structure and operating conditions of the aerodynamic lens are the same as those for an Aerodyne AMS, which is based on the design of Liu et al. (Citation1995) and Zhang et al. (Citation2004), the difference in the transmission efficiency for these two dva is likely small (Takegawa et al. Citation2009). This assumption has been confirmed by altering the mobility diameters of dry KNO3 particles, as discussed in section 4.4.2.
shows the temporal evolution of the ion signal at m/z 30 for solid and oil-coated KNO3 particles. The evolution took place faster than solid KNO3 particles. As mentioned in section 4.1, solid particles tended to be scattered compared with oil-coated particles because of bouncing. We interpreted that the faster evolution for oil-coated particles was due to a more concentrated distribution of particles on the trap. shows the temporal evolution of the ion signal at m/z 44 for oil-coated particles and for zero air. The m/z 44 signal was chosen because of its better S/N ratio compared with other peaks such as m/z 57. It should be noted that the m/z 30 and 44 signals were not obtained simultaneously because of the limited duty cycle of the QMS. The presence of m/z 44 was due to either decarboxylation of oleic acid or oxidation of carbon to CO2 on the Pt surface. The yield of m/z 44 signal from oleic acid may depend on the laser power, but a quantitative estimate of the yield has not been performed at the present stage.
FIG. 12 (a) Temporal evolution of i 30 for solid (shaded) and oil-coated (solid) KNO3 particles. (b) Temporal evolution of i 44 for oil-coated particles (solid) and for zero air (dashed). It should be noted that the m/z 30 in (a) and m/z 44 signals in (b) were not simultaneously obtained because of the limited duty cycle of the QMS.
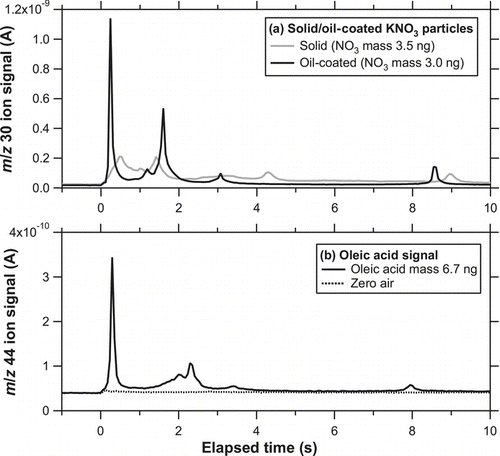
shows correlation plots of Q 30 versus the accumulated mass of NO− 3 for solid and oil-coated KNO3 particles. As for the oil-coated case, the regression line was forced through the data point with an NO− 3 mass of zero because of the relatively small dynamic range. There was no significant difference in the regression slope between particles with and without oil coating. The slope ratio of the solid to oil-coated cases, which represents the collection efficiency for solid KNO3 particles, was found to be 0.96 ± 0.14 (the error is defined as the 95% confidence interval). The same experiments were performed on a different day and also in the preliminary test, and the results were found to be 1.12 ± 0.16 and 1.04 ± 0.10, respectively. These results suggest that the collection efficiency for solid KNO3 particles can be considered as ∼100% with an experimental error of ∼15%. We also performed the same test by removing the five mesh layers (i.e., only the bottom plate remained) and found that the slope for solid KNO3 particles was only <∼10% of that for oil-coated KNO3 particles. This indicates that the mesh layers successfully prevented particles from being lost by bouncing.
FIG. 13 Correlation plots of Q 30 versus the accumulated mass of NO− 3 for solid and oil-coated KNO3 particles. The solid and dashed lines represent the regression for solid and oil-coated particles. As for the oil-coated case, the regression line was forced through the data point with an NO− 3 mass of zero because of the relatively small dynamic range.
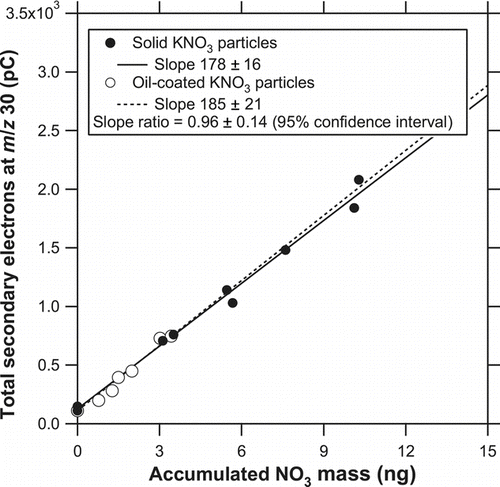
4.3.4. Other Fragments
Effects of other fragments on the evaluation of the collection efficiency are discussed. As mentioned previously, full mass spectra during the evolution of the ion signals were not obtained because of the limited duty cycle of the QMS. We measured all possible nitrogen-containing peaks (m/z 14: N+, 30: NO+, and 46: NO+ 2) and the potassium peak (m/z 39: K+) originating from KNO3. The m/z 14 signal was measured simultaneously with m/z 30, but m/z 46 and 39 were measured separately because the signal levels of these peaks were low and longer dwell times were required. We estimated the fragment ratios of m/z 46 to 30 and m/z 39 to 30 by comparing the sensitivities (S 30, S 46, and S 39). It was found that the m/z 46 and 39 signals were only ∼0.3% and ∼1% of the m/z 30 signal, respectively. The dominance of m/z 30 over m/z 46 was also found in mass spectra of KNO3 particles in an Aerodyne AMS (Takegawa et al. Citation2009), which is based on flash vaporization of particles by impaction on a heated tungsten surface (600°C).
shows the temporal evolution of the ion signals at m/z 14 and 30 for solid KNO3 particles. We can see that the m/z 14 signal nearly followed the m/z 30 signal during the evolution. The m/z 14 signal was found to be ∼6% of the m/z 30 signal. A similar amount of m/z 14 (∼4% of m/z 30) was also assumed in an Aerodyne AMS (based on laboratory experiments). Note that it is somewhat difficult to measure the m/z 14 signal from nitrate in the AMS mass spectra because of the large contribution from N2 in air. There was no significant change in this ratio between dry and oil-coated particles (not shown). The m/z 14 was not included in the preceding results because of its poor S/N ratio, but neglecting m/z 14 does not affect the major conclusions of this study.
FIG. 14 Temporal evolution of i 14 (shaded) and i 30 (solid) for solid KNO3 particles (dm = 250 nm).
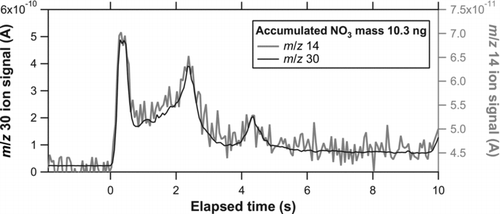
Although the vaporization process is different between the PT-LDMS and AMS, the fragment patterns of KNO3 were similar. This may be due to the simple molecular structure of KNO3. It is possible that the fragment patterns could be significantly different for some organic compounds.
4.4. Error Analysis for the Evaluation of the Collection Efficiency
4.4.1. Uncertainty in the Ion Signals
The uncertainty in Q 30 originates from the overall detection efficiency of the evolved gas, which is governed by three processes: vaporization of KNO3, transport of NO molecules in the cell, and ionization of NO molecules and detection of NO+ ions. The vaporization efficiency was likely close to 100% as discussed in section 4.3.1. The transport efficiency of NO molecules may be affected by the loss of NO in the quartz cell. We compared the data obtained at T cell = 42°C with those at T cell = 120°C and found no significant difference, suggesting that adsorption of NO on the quartz cell was minor. The efficiency of ionization of NO molecules and detection of NO+ ions depends on the condition of the filament and ion detector. We consider that normalization by the m/z 32 signal can account for the drift of the ionization and detection efficiencies. Uncertainty also originates from the fluctuation of the artifact. Considering the fluctuation of the artifact (2σ) of ∼0.3 ng, the Q 30 value may have ∼10% error for the accumulated NO− 3 mass of 3 ng.
We previously mentioned the possible effect of the deposition of bounced particles in the cell. Because T cell of <120°C in this study was far lower than the dissociation temperature of KNO3, the vaporization of KNO3 deposited on the cell was likely negligible. In fact, there was no systematic difference in the artifact level during the experiments associated with repeated accumulation and vaporization process (∼12 cycles with mass loadings of up to ∼11 ng).
4.4.2. Uncertainty in the Accumulated Mass of NO− 3
The uncertainty in the accumulated mass of NO− 3 on the particle trap originates from the uncertainty in the flow rate and the correction for multiply charged particles in the DMA. The uncertainty in the flow rate is estimated to be 4% (2σ). The estimate of multiply charged particles was based on the method presented by Wang et al. (Citation2010) and Takegawa and Sakurai (Citation2011). The vacuum aerodynamic diameters are 525 and 869 nm for solid KNO3 particles with single and double charges, respectively, and 496 and 848 nm for oil-coated KNO3 particles with single and double charges, respectively. We assumed that the transmission efficiency of the aerodynamic lens was nearly 100% (or at least stable at a certain value) at dva < ∼600 nm and gradually reduced at dva > ∼600 nm, following the results of Takegawa et al. (Citation2009). However, some studies have shown that the transmission efficiency may have started to decrease at smaller dva , even though the same type of aerodynamic lens was used (Liu et al. Citation2006). Possible uncertainties associated with the above assumption should be further investigated.
First, the uncertainty due to the stability of the transmission efficiency at dva < ∼600 nm is estimated. We compared the data obtained for dm = 250 nm with those for dm = 300 nm and found no systematic difference. These mobility diameters correspond to dva = 525 and 630 nm, respectively, suggesting that variability of the transmission efficiency at dva < ∼600 nm had a minor effect on the final results. Second, the uncertainty due to reduced transmission efficiency at dva > ∼600 nm is estimated. We did not make any corrections for this effect (i.e., the transmission efficiency at dva = 525 and 869 nm is assumed to be the same) because of insufficient data to support this correction. This effect may lead to a slight overestimation of the accumulated mass of NO− 3: if the actual transmission efficiency at dva = 869 nm is 60% of that at dva = 525 nm, following the results of Takegawa et al. (Citation2009), the mass of NO− 3 could be overestimated by ∼12% for solid KNO3 particles. On the other hand, the effect is negligible for oil-coated KNO3 particles because the ratio of doubly to singly charged particles was very small.
In summary, we currently identified several sources of systematic errors in the quantities shown in , and the combined uncertainty is comparable to the experimental error of 15% (section 4.3.3). We do not exclude the possibility of other missing sources of errors in the experiments. What we can conclude here, however, is that the collection efficiency for solid KNO3 particles agreed with that for oil-coated KNO3 particles to within the experimental error of ∼15%, based on the currently identified sources of uncertainty.
4.5. Detection of (NH4)2SO4 Particles
The response of the instrument to (NH4)2SO4 particles was briefly tested as it is one of the major compounds in ambient aerosol. Possible changes in the fragment patterns of (NH4)2SO4 due to changes in the laser power were not systematically investigated, and only the m/z 14, 32, 48, and 64 signals were monitored. The cell temperature was kept at 120°C to reduce the possible adsorption of sulfur compounds on the quartz cell.
shows the temporal evolution of the ion signals at m/z 48 and 64 for solid (NH4)2SO4 particles (dm = 250 nm) and for zero air at a laser power of 3.9 W. The evolution took place faster than KNO3 probably because (NH4)2SO4 decomposes at a lower temperature (280°C). No significant artifact was observed either at m/z 48 or 64, at least within the experimental uncertainty. shows correlation plots of Q 48 + Q 64 versus the accumulated mass of SO4. The ratio of Q 48 to Q 64 was 0.79 ± 0.28 (average ±1σ). A linear relationship (slope = 4.4 ± 0.6, r 2 = 0.92) was found, suggesting that the instrument can quantify SO4 mass as long as the yields of these m/z signals are reproducible and also an absolute calibration is properly performed. Further investigation is needed to evaluate the detection capability for (NH4)2SO4 in detail.
5. DISCUSSION
Several key points for future improvements are discussed below. The temporal evolutions of the signals during the detailed test ( and ) were systematically slower than those observed in the preliminary test (Figures and ), even though the laser powers were comparable. Considering that the particle trap was replaced after the preliminary test, it is possible that subtle differences in the Pt coating thickness between the traps may have resulted in substantial variability of the surface reflectance and thermal conductivity (and thus the susceptibility to laser heating). The nonnegligible artifact at m/z 30 may also be due to the surface properties of the Pt coating that is currently used. While Pt is useful as a coating material because of its catalytic properties and corrosion resistance, the coating method should be modified to improve the susceptibility to laser heating and artifact.
The laser beam profile is also important for regulating the desorption rate. A higher laser power density would be required for efficient detection of refractory compounds. However, the inhomogeneity of the laser beam intensity of the Gaussian profile, together with the possible variability of the susceptibility to laser heating, can result in evolution that is too fast in some cases (if the distribution of particles on the trap completely overlaps the center of the laser beam). This is not favorable for the detection by QMS. Also, it has a risk of damaging the trap due to overheating in the center of the Gaussian beam profile. Reforming the laser power profile (from Gaussian to flat-top) may mitigate too rapid evolution and also local heating of the trap. Ramping the laser power may also be useful for controlling the desorption rate.
Adsorption of the evolved gas (NO) on the quartz cell was likely minor in this study. However, these effects may be an important issue in some cases, especially for nonvolatile organic compounds. In the current configuration, the cell can be heated up to 400°C. Various T cell conditions (while keeping T trap as low as possible during the sampling) need to be tested for the detection of multiple compounds including both semivolatile and nonvolatile compounds.
The stability of the sensitivity is an important issue, as it affects the frequency of absolute calibrations. As mentioned previously, the m/z 30 signal was normalized by the m/z 32 signal to account for temporal drifts of the ion detection efficiency within a day, and the instrument sensitivity at m/z 30 (S 30) after the corrections showed good reproducibility. Attempts were also made to correct the data obtained on different days, and the correction seemed to work well in some cases. Further experiments are needed to establish absolute calibration procedures.
6. SUMMARY
The development of a PT-LDMS has been described, focusing on the structure and performance of the particle trap. The bounce of solid particles on collection substrates caused uncertainties in the quantitative measurements of aerosol chemical compositions in previously reported mass spectrometric analyzers, including TDPBMS and AMS. The most important advance compared with the previously reported analyzers is a newly designed particle trap, which consists of five mesh layers characterized by a large areal porosity from the direction perpendicular to the mesh plane and a good mesh frame arrangement. Particles colliding on the mesh frame may bounce upon a single impaction, but eventually lose their kinetic energy and be captured in the course several impactions. The mechanism of the particle trapping is significantly different from traditional particle filters.
Laboratory experiments using KNO3 particles have demonstrated the proof of concept of the particle trap. The SEM observations of the particle trap clearly showed the deposition of solid or oil-coated particles on the mesh frames. We systematically measured the dependence of ion signals at m/z 30 (NO+) originating from KNO3 particles on the power, diameter, and position of the CO2 laser beam. The appropriate condition for the evaluation of the particle trap (i.e., the detection of KNO3 particles) was determined based on these results. The collection efficiency for solid KNO3 particles was evaluated by comparing the ion signals originating from oil-coated KNO3 particles. We conclude that the collection efficiency for solid KNO3 particles was ∼100% to within the experimental error of ∼15%, assuming that oil-coated particles are collected with 100% efficiency.
Reliable measurements of ambient aerosol will be possible after some modifications and optimization. They include the choice of coating material of the particle trap, optimization of laser irradiation, and controlling T cell and T trap at appropriate levels. Establishing a methodology for routine calibrations is also important for continuous measurements in the field.
Acknowledgments
This study was funded by the SENTAN program of the Japan Science and Technology Agency (JST).
REFERENCES
- Biswas , P. and Flagan , R. C. 1988 . The Particle Trap Impactor . J. Aerosol Sci. , 19 : 113 – 121 .
- Chang , M. , Kim , S. and Sioutas , C. 1999 . Experimental Studies on Particle Impaction and Bounce: Effects of Substrate Design and Material . Atmos. Environ. , 33 : 2313 – 2322 .
- DeCarlo , P. , Slowik , J. G. , Worsnop , D. R. , Davidovits , P. and Jimenez , J. L. 2004 . Particle Morphology and Density Characterization by Combined Mobility and Aerodynamic Diameter Measurements. Part 1: Theory . Aerosol Sci. Technol. , 38 : 1185 – 1205 .
- Jayne , J. T. , Leard , D. C. , Zhang , X. F. , Davidovits , P. , Smith , K. A. , Kolb , C. E. and Worsnop , D. R. 2000 . Development of an Aerosol Mass Spectrometer for Size and Composition Analysis of Submicron Particles . Aerosol Sci. Technol. , 33 ( 1–2 ) : 49 – 70 .
- Liu , P. S. K. , Deng , R. , Smith , K. A. , Williams , L. R. , Jayne , J. T. , Canagaratna , M. R. , Moore , K. , Onasch , T. B. , Worsnop , D. R. and Deshler , T. 2006 . Transmission Efficiency of an Aerodynamic Focusing Lens System: Comparison of Model Calculations and Laboratory Measurements for the Aerodyne Aerosol Mass Spectrometer . Aerosol Sci. Technol. , 41 ( 8 ) : 721 – 733 .
- Liu , P. , Ziemann , P. J. , Kittelson , D. B. and McMurry , P. H. 1995 . Generating Particle Beams of Controlled Dimensions and Divergence: I. Theory of Particle Motion in Aerodynamic Lenses and Nozzle Expansions . Aerosol Sci. Technol. , 22 : 293 – 313 .
- Mader , B. , Flagan , R. C. and Seinfeld , J. H. 2001 . Sampling Atmospheric Carbonaceous Aerosols Using a Particle Trap Impactor/Denuder Sampler . Environ. Sci. Technol. , 35 : 4857 – 4867 .
- Matthew , B. M. , Onasch , T. B. and Middlebrook , A. M. 2008 . Collection Efficiencies in an Aerodyne Aerosol Mass Spectrometer as a Function of Particle Phase for Laboratory Generated Aerosols . Aerosol Sci. Technol. , 42 : 884 – 898 .
- Morrical , B. D. , Fergeson , D. P. and Prather , K. A. 1998 . Coupling Two-Step Laser Desorption/Ionization with Aerosol Mass Spectrometry for the Analysis of Individual Organic Particles . J. Amer. Soc. Mass Spectr. , 9 : 1068 – 1073 .
- Moteki , N. and Kondo , Y. 2007 . Effects of Mixing State on Black Carbon Measurements by Laser-Induced Incandescence . Aerosol Sci. Technol. , 41 : 398 – 417 .
- Murphy , D. M. and Thomson , D. S. 1995 . Laser Ionization Mass Spectroscopy of Single Aerosol Particles . Aerosol Sci. Technol. , 22 : 237 – 249 .
- Ng , N. L. , Herndon , S. C. , Trimborn , A. , Canagaratna , M. R. , Croteau , P. L. , Onasch , T. B. , Sueper , D. , Worsnop , D. R. , Zhang , Q. , Sun , Y. L. and Jayne , J. T. 2011 . An Aerosol Chemical Speciation Monitor (ACSM) for Routine Monitoring of the Composition and Mass Concentrations of Ambient Aerosol . Aerosol Sci. Technol. , 45 : 770 – 784 .
- Noble , C. A. and Prather , K. A. 1996 . Real-Time Measurement of Correlated Size and Composition Profiles of Individual Atmospheric Aerosol Particles . Environ. Sci. Technol. , 30 : 2667 – 2680 .
- Öktem , B. , Tolocka , M. P. and Johnston , M. V. 2004 . On-Line Analysis of Organic Components in Fine and Ultrafine Particles by Photoionization Aerosol Mass Spectrometry . Anal. Chem. , 76 : 253 – 261 .
- Rao , A. K. and Whitby , K. T. 1978 . Non-Ideal Collection Characteristics of Inertial Impactors—I. Single-Stage Impactors and Solid Particles . J. Aerosol Sci. , 9 : 77 – 86 .
- Su , Y. X. , Sipin , M. F. , Furutani , H. and Prather , K. A. 2004 . Development and Characterization of an Aerosol Time-of-Flight Mass Spectrometer with Increased Detection Efficiency . Anal. Chem. , 76 : 712 – 719 .
- Takegawa , N. , Miyakawa , T. , Kondo , Y. , Jimenez , J. L. , Zhang , Q. , Worsnop , D. R. and Fukuda , M. 2006 . Seasonal and Diurnal Variations of Submicron Organic Aerosol in Tokyo Observed Using the Aerodyne Aerosol Mass Spectrometer . J. Geophys. Res. , 111 : D11206
- Takegawa , N. , Miyakawa , T. , Watanabe , M. , Kondo , Y. , Miyazaki , Y. , Han , S. , Zhao , Y. , van Pinxteren , D. , Bruggemann , E. , Gnauk , T. , Herrmann , H. , Xiao , R. , Deng , Z. , Hu , M. , Zhu , T. and Zhang , Y. 2009 . Performance of an Aerodyne Aerosol Mass Spectrometer (AMS) During Intensive Campaigns in China in the Summer of 2006 . Aerosol Sci. Technol. , 43 : 189 – 204 .
- Takegawa , N. , Miyazaki , Y. , Kondo , Y. , Komazaki , Y. , Miyakawa , T. , Jimenez , J. L. , Jayne , J. T. , Worsnop , D. R. , Allan , J. D. and Weber , R. J. 2005 . Characterization of an Aerodyne Aerosol Mass Spectrometer (AMS): Intercomparison with Other Aerosol Instruments . Aerosol Sci. Technol. , 39 : 760 – 770 .
- Takegawa , N. and Sakurai , H. 2011 . Laboratory Evaluation of a TSI Condensation Particle Counter (Model 3771) Under Airborne Measurement Conditions . Aerosol Sci. Technol. , 45 : 272 – 283 .
- Tobias , H. J. , Kooiman , P. M. , Docherty , K. S. and Ziemann , P. J. 2000 . Real-Time Chemical Analysis of Organic Aerosols Using a Thermal Desorption Particle Beam Mass Spectrometer . Aerosol Sci. Technol. , 33 : 170 – 190 .
- Wang , X. , Caldow , R. , Sem , G. J. , Hama , N. and Sakurai , H. 2010 . Evaluation of a Condensation Particle Counter for Vehicle Emission Measurement: Experimental Procedure and Effects of Calibration Aerosol Material . J. Aerosol Sci. , 41 : 306 – 318 .
- Woods , E. III , Smith , G. D. , Miller , R. E. and Baer , T. 2002 . Depth Profiling of Heterogeneously Mixed Aerosol Particles Using Single-Particle Mass Spectrometry . Anal. Chem. , 74 : 1642 – 1649 .
- Zelenyuk , A. and Imre , D. 2005 . Single Particle Laser Ablation Time-of-Flight Mass Spectrometer: An Introduction to SPLAT . Aerosol Sci. Technol. , 39 : 554 – 568 .
- Zelenyuk , A. , Yang , J. , Choi , E. and Imre , D. 2009a . SPLAT II: An Aircraft Compatible, Ultra-Sensitive, High Precision Instrument for In-Situ Characterization of the Size and Composition of Fine and Ultrafine Particles . Aerosol Sci. Technol. , 43 : 411 – 424 .
- Zelenyuk , A. , Yang , J. , Imre , D. and Choi , E. 2009b . Achieving Size Independent Hit-Rate in Single Particle Mass Spectrometry . Aerosol Sci. Technol. , 43 : 305 – 310 .
- Zhang , X. , Smith , K. A. , Worsnop , D. R. , Jimenez , J. L. , Jayne , J. T. , Kolb , C. E. , Morris , J. and Davidovits , P. 2004 . Characterization of Particle Beam Collimation: Part II. Integrated Aerodynamic Lens-Nozzle System . Aerosol Sci. Technol. , 38 ( 6 ) : 619 – 638 .