Abstract
This paper describes the design and performance of a photo-acoustic aerosol absorption spectrometer (PAS) built for operation on a research aircraft platform. The PAS instrument is capable of measuring dry absorption at 659 nm, 532 nm, and 404 nm, and absorption enhancement due to coatings at 532 nm and 404 nm. The measurement accuracy for all channels is < = 10% and in flight 1 Hz sensitivities lie within the range of 0.5–1.5 Mm−1. PAS measurements of calibrated absorbing aerosol samples are shown to be consistent with measurements made by a previous generation single channel photo-acoustic instrument. Aircraft data collected during a recent field campaign in California are used to demonstrate the capabilities of the PAS. In combination with an aircraft cavity ring down aerosol extinction spectrometer described in a companion paper, the new PAS instrument provides a sensitive airborne in-situ characterization of aerosol optics.
Copyright 2012 American Association for Aerosol Research
1. INTRODUCTION
Black carbon (BC) is estimated to contribute about 20–50% of the present-day warming of CO2 (IPCC 2007; Ramanathan and Carmichael Citation2008) and can also perturb atmospheric heating profiles, cloud lifetimes and the development of regional precipitation patterns (Ramanathan and Carmichael Citation2008). Where major sources of anthropogenic emissions and biomass burning exist, BC aerosol can contribute 1.2–1.8 K of heating per day (Ramanathan et al. Citation2007). Evidence is also emerging that the climate impact of BC evolves throughout its emission to deposition lifecycle, including changes to mixing state (Jacobson Citation2001), incorporation into clouds (Koch and Del Genio Citation2010) and impact based on geographic location (i.e., above clouds or snow, Flanner et al. Citation2007; Doherty et al. Citation2010; Zarzycki and Bond Citation2010). Ramanathan and Carmichael (Citation2008) identified four major uncertainties in the understanding of the climate impact of BC: vertical distributions, mixing state, emissions inventories, and the indirect effect on clouds.
It is apparent that for most of its atmospheric lifetime, BC is coated in inorganic and organic materials (Quinn et al. Citation2006; Zhang et al. Citation2007; Schwarz et al. Citation2008a). These coatings can result in (1) collapse of the fractal BC cores emitted from efficient combustion (Zhang et al. Citation2008) and (2) enhanced absorption of BC by lensing radiation through the coating to the BC core (Schnaiter et al. Citation2005; Bond et al. Citation2006). This lensing can increase total absorption by 50 to 100%. In addition, recent studies have shown that a significant amount of organic aerosol, which can coat BC cores, also absorbs radiation at wavelengths from mid visible (532 nm) to the near UV (350 nm) (Barnard et al. Citation2008). Not only does this so-called brown carbon (BrC) absorb radiation itself, it too can generate a lensing effect upon coating BC (Lack and Cappa Citation2010).
Given the potential climate impacts of the phenomenon outlined above, accurate in-situ measurements of the optical properties of BC and BrC are needed (Moosmuller et al. Citation2009). Traditional thermal methods of measuring the mass of BC have significant uncertainties or biases (Boparai et al. Citation2008). More recently, measurements of BC through laser-induced incandescence have provided powerful insight into the distribution of BC from local to global scales (Schwarz et al. Citation2010) and on the potential for BC particles to be coated (Schwarz et al. Citation2008b). These mass-based methods, however, do not directly measure the radiative impact of BC or BrC absorption or the magnitude of the absorption enhancement. Traditional filter-based methods of measuring in-situ absorption also have been shown to exhibit significant variability (Muller et al. Citation2011) and biases (Lack et al. Citation2008; Lack et al. Citation2009; Nakayama et al. Citation2010). Remote sensing instrumentation, like sun photometers and satellites (Schmid et al. Citation2000) provide column integrated estimates of ambient aerosol optical properties but cannot easily deconvolve the mixing state or contributions from non-BC absorbers. Photo-acoustic absorption spectroscopy (PAS) has been shown to have high accuracy (Lack et al. Citation2006), the ability to measure absorption enhancements from clear coatings (Lack et al. Citation2009; Havey et al. Citation2010), be deployable on aircraft platforms (Arnott et al. Citation2006), show immunity to biases observed for filter based methods (Lack et al. Citation2009), and to be able to deduce the contributions of BrC absorption (Lewis et al. Citation2008; Chakrabarty et al. Citation2010).
As the understanding of the climate impact of BC and BrC evolves, it is apparent that some important progress in absorption measurement techniques must also occur. These include (1) accurate, sensitive vertical profiling on airborne platforms, (2) identification of the contribution of absorption via enhancements (from organic and water coatings), (3) determining the wavelength dependence of absorption in the visible/near UV spectrum, and (4) characterizing absorption related to BrC. We developed a high sensitivity aircraft PAS system that addresses many of these issues. This PAS operates five independent cavities; three sampling dry aerosol at 404 nm, 532 nm, or 659 nm, and two sampling thermo-denuded aerosol at 404 nm and 532 nm. Here, we focus on operation of this new instrument as regards to the first three points and provide performance examples from in-field deployment during the 2010 NOAA CalNex campaign (http://www.esrl.noaa.gov/csd/calnex/). Details related to BrC absorption as well as absorption enhancements attributable to water uptake will be covered in future manuscripts.
2. INSTRUMENT DESIGN
Photo-acoustic spectroscopy utilizes a resonant cavity and a laser that is amplitude-modulated at the cavity resonant frequency (FR ). Absorption by constituents of the sampled air leads to generation of a pressure wave that is amplified by the resonator. If the absorbed energy (in the form of a pressure wave) reaches the sensitive microphones within the acoustic period (1/FR ) the detected sound is directly related to the total light absorption via a calibration constant. Delays in energy return within the acoustic period result from energy investment into evaporation, molecular dissociation or fluorescence or thermal relaxation delays in large particles (Raspet et al. Citation2003; Murphy Citation2009; Gillis et al. Citation2010). In the system described here, the RH (<20%) and particle sizes (2 μm size cut) are sufficiently low that these issues negligibly affect the measured signal. The technique has been widely applied to gas and particle phase absorption measurements. Arnott et al. (Citation1999) and Nägele and Sigrist (Citation2000) provide comprehensive descriptions of the PAS technique which the reader is referred to for further details.
The PAS described here expands on the fundamental PAS design by focusing on sensitivity, accuracy, and noise immunity under field conditions. The instrument employs five cells; (1) 404 nm dry, (2) 404 nm thermo-denuded (i.e., heated), (3) 532 nm dry, (4) 532 nm thermo-denuded, and (5) 659 nm dry. Alternatively, the 532 nm thermo-denuded channel can be replaced with a 532 nm elevated RH channel. The operating conditions chosen for these cells simultaneously provide for the ability to explore the wavelength dependence of absorption and the effects of coatings.
The fundamental design principles to address the needs identified above and provide guidance for instrument development (and described in detail in this manuscript) were identified as:
1. | [Sensitivity] High laser power with low incident energy per particle: PAS instrument sensitivity is directly related to laser power, and yet particle properties can be altered (via incandescence or evaporation) if too much energy is introduced. | ||||
2. | [Sensitivity + Airborne Deployment] Noise isolation: For successful deployment on aircraft platforms physical isolation from vibration and noise sources is essential. Other noise rejection/cancellation/minimization design features are also required to optimize operation. | ||||
3. | [Accuracy] Regular calibrations /stable operating conditions: Rapid temperature and pressure changes in an aircraft can quickly de-tune a resonant PAS cavity. Temperature stabilization, rapid pressure calibrations and accurate microphone calibrations are essential to maintain high quality data collection. |
2.1. General Resonator Design
shows a schematic of the PAS cell design used in the instrument. The cell design has changed considerably with respect to a previous generation version described in Lack et al. (Citation2006). The cell is machined from aluminum and employs dual half-wavelength resonators (11 cm long, 1.9 cm diameter) capped on either end with ¼ wavelength acoustic notches. Each cell has a total sample volume of 185 cm3. Cell length was optimized to produce resonant frequencies (FR ) within a range least affected by NOAA WP-3D aircraft propeller noise and other peaks in the acoustic noise spectrum of the aircraft operating under common flight conditions. The dimensions produce an FR in the range of 1320–1360 Hz and cell quality factors (Q) of 50–90 over the pressure and temperature ranges of 20–90 kPa and 285–295 K (Section 3.2 and Figure 3). While both resonators are open to sample flow, only one is exposed to power modulated laser light; the other provides for noise cancellation (Section 2.7). Knowles AcousticsFootnote 1 (Itasca, IL, USA) EK-3132 Electret microphones are placed at the antinode of the sound wave at the center of each resonator (). A Knowles Acoustics EP-7369 speaker is placed in the background resonator and used to measure the cell FR and measure Q (Section 3.1). All cell connections are sealed with O-rings.
2.2. Lasers and Optics
The system uses two 60 mw, 403.6 nm diode laser modules, one 60 mw 659.1 nm diode laser module (all from Diode Laser Concepts1, Central Point, OR, USA) and two 200 mw 532.1 nm diode pumped solid state lasers (Snake Creek Laser1, Halstead, PA, USA). Each laser's wavelength was measured to ensure accurate reference to absorption cross-sections of NO2 and O3 used for calibration (Section 3.3). Here, we refer to each channel as 404 nm, 532 nm, and 659 nm. Each laser is fitted with beam collimation optics producing a <1.5 mm diameter circular beam. Laser power is modulated at the FR of the cell. The lasers are mounted directly into a 5-axis optic mount (Newport1 LPV-1, Irvine, CA, USA). This optical mount is placed directly behind the entrance mirror of the astigmatic multipass cell (McManus et al. Citation1995).
The astigmatic cell consists of two mirrors (ARW Optical1, Wilmington, NC, USA) spaced 35 cm apart, with the “entrance” mirror having a cylindrical radius of curvature of 43 cm and a 2 mm hole drilled in the center. The second (or back) mirror is also cylindrical with a radius of curvature of 47 cm, which is rotated 90° to the radius of curvature of the entrance mirror. Each mirror is coated with a dielectric coating (R > 99.5%) specific to the wavelength of light in use. With appropriate alignment this multipass configuration theoretically produces 182 passes before laser light exits the entrance hole. The PAS cell is mounted within the path of the laser multipass. The multipath light passes through the PAS cell via two 1 mm thick windows (CVI Laser1, Albuquerque, NM, USA), each with a high-transmission antireflective coating specific to the wavelength of light in use. Each window has transmission of > 99.5%. The effective laser power in each multipass cell can be calculated using Equationequation 1 (Nägele and Sigrist Citation2000), and is shown in .
TABLE 1 Details of lasers, laser power, and effective PAS cell power. The PAS cell circulating power calculations are estimates based upon alignment of the multipass cells for 182 passes
2.3. Flow Control and Conditioning
Air is sampled into the PAS instrument through a single inlet at a total flow rate of up to 10 LPM. The total flow is dried to ∼10% RH using a nafion multitube drier (Perma Pure PD-100T, 25 cm length, stainless steel housing) and is split into five separate lines using a custom built manifold. A mass flow controller (MC-5LPM-D; Alicat Scientific Tuscon, AZ, USA) located at the outlet of each cell is used to control sample flow and to monitor cell pressure. Suction is provided by a single, compact scroll pump (Air Squared V11H12N2.5, Broomfield, CO, USA). Flow in each cell cannot exceed 2 LPM before flow noise increases to prohibitive levels. At 2 LPM, the sample residence time within each cell is approximately 5.5 s, which is sufficient for rapid sampling on an aircraft platform. RH and temperature are monitored in each cell using a thermo hygrometer probe (HMP50; Vaisala, Helsinki, Finland) positioned through one of the acoustic notch end caps. An electronically actuated 3-way valve allows sample flow to be directed through a filter (United Filtration Systems DIF-LK50-1/4”, Sterling Heights, MI, USA) to provide particle free measurement. A Mercury pen-ray lamp (UVP1 90-0004-01, Upland, CA, USA) is positioned within the filtered flow line to provide ozone (O3) for calibrations. The flow system is constructed primarily of aluminum to avoid particle, O3 and NO2 losses (the latter absorbing gases being used for calibration). Sample flow can optionally be directed through a thermo-denuder (Section 2.5).
2.4. Temperature Control
The FR of PAS cells are sensitive to changes in operating conditions and particularly temperature. We therefore pay particular attention to controlling the temperature of each cell. The easiest temperature control is usually to heat an instrument to a fixed temperature that is higher than usually encountered. However, water evaporation artifacts on the PAS signal rapidly worsen as temperature increases because of the increased vapor pressure of water (Murphy Citation2009). In addition, semi-volatile species may start to evaporate. We therefore chose to control the 5 PAS cells (housed within an acoustically and thermally isolated box) at 290 (± 1) K using a thermo electric cooler (TEC) and controller (TE Technology1 LC-200, TC-36-25 RS232, Traverse City, MI, USA). In addition, each cell is mounted on an individual TEC (TE Technology1 HP-199-1.4-1.5, Traverse City, MI, USA) that makes contact with the optical table through thermally conductive foam pads, the use of which minimizes vibration transfer to the cells. These individual TECs maintain the individual cell temperatures to within ± 0.1°C (Wavelength Electronics WTC3243, Bozeman, MT, USA). This finer temperature control limits FR shifts associated with changes in temperature to less than ± 0.3 Hz.
2.5. Thermo-Denuder
A custom thermo-denuder was built following the design of Fierz et al. (Citation2007). The denuder consists of a 35 cm long 6.35 mm OD stainless steel tube heated to 448 K followed by a 25 cm section of 6.35mm ID perforated stainless steel tubing which is surrounded in activated carbon felt (Kynol CAN-305-15, American Technical Trading Inc, NY, USA) heated to 523 K. At a flow rate of 1 lpm, the effective residence time in the thermo-denuder is 1.5 s. The activated carbon section absorbs volatile species that evaporate from the heated aerosol. It is periodically regenerated by heating to >773 K. In the work by Fierz et al. (Citation2007) this denuder configuration was shown to have almost complete removal of coatings of a semi-volatile organic compound described as representing a “worst case scenario” (Di-Ethyl-Hexyl-Sebecate, boiling point of 300°c) coating sodium chloride cores. In addition, they showed minimal losses >20 nm particle diameter at temperatures ranging from 298 K to 473 K (see Section 2.8 for details of particle loss within the denuder used for this instrument).
2.6. Physical Noise Isolation
Multiple layers of physical noise isolation have been built into this instrument. Each PAS cell is ‘soft’ mounted to the optical table via four neoprene mounts. The optical table, on which the five cells are mounted, is itself mounted to the PAS enclosure using wire rope isolators (Enidine WR3-400-10-D, Orchard Park, NY, USA.) The PAS enclosure is lined with thermally and acoustically isolating foam rubber and is also mounted to the instrument rack using wire rope isolators (Enidine WR3-100-10-D). Finally, the instrument rack itself is mounted on wire rope isolators (Enidine WR6-600-10-D). All wire rope isolators are specified to have frequency damping at the natural frequency of the components being supported. All aluminum sample lines are ‘soft’ connected to junction points using short collars of conductive silicone tubing. At each connection point less than 5 mm of conductive tubing is exposed to the sample flow to minimize any potential issues that have been shown to arise with the use of conductive silicone tubing (Timko et al. Citation2009). All sample lines are also externally shrouded in soft neoprene rubber to minimize vibration transfer from the ambient environment. Noise buffers (in the form of in-line particle filters) positioned between the PAS cells and pumps minimize the introduction of pump noise into the sample volume.
2.7. Electronics/Software and Noise Isolation
In addition to mechanical noise isolation, attention has also been given to acoustic and electronic noise isolation in the present system. As mentioned in Section 2.1, the resonators are capped on either end with acoustic notches. In theory these notches cancel out any noise at the cell FR . However, in practice their effectiveness does not reach theoretical limits, in part due to physical limitations in machining purely symmetrical resonators and notches. Any noise that is present at the FR within a PAS cell will be detected equally by the microphones in both resonators. Differential amplification electronics subtract the signal in both resonators to remove this background noise. The amplifier chain also incorporates a band pass filter that limits detectable signals to those with frequencies between 300 Hz and 10 KHz. The differential amplification does not remove noise that is not common to both resonators, such as that associated with absorption of the modulated laser beam by the cell walls or windows. Such remaining sources of noise can therefore contribute to the measured signal.
2.8. Particle Losses
Particle losses through the PAS cells from gravitational settling and inertial deposition were evaluated using the Max Planck Institute Particle Loss Calculator (von der Weiden et al. 2009). For a 1 LPM flow rate, estimated loss within each cell and through the inlet tubing is <1.5% for sub-1 μm particles, ∼6% for 2 μm particles and ∼30% for 5 μm particles. The predicted loss of sub-1 μm aerosol was confirmed experimentally by measuring extinction (using CRDS, Langridge et al. 2011) of mono-disperse polystyrene spheres before and after a PAS cell. Particle losses through the thermo-denuder were calculated to be < = 1% (1 μm diameter) and were measured experimentally (using CRDS) to not exceed 2% for particles < = 1 μm diameter. Particle losses through the drier were measured to not exceed 10% for all aerosol diameters below 2 μm (Langridge et al. Citation2011). We do not correct data for these losses and, therefore, reported data are a lower limit.
3. INSTRUMENT CALIBRATION AND CHARACTERIZATION
Measured photoacoustic absorption is acquired by modulating the laser in the cavity at the current FR and then acquiring 1 s of microphone signal. That signal is then converted to the frequency domain via a fast Fourier transform (FFT). All signals that can not be distinguished from noise are then removed from the signal of interest (Hildebrand and Sekhon Citation1974). After the initial background is removed, the area of the residual signal is integrated in the region of interest (i.e., about the FR ) and the resulting integrated area (IA) is then converted to absorption via a calibration based on the process described in the following sections.
3.1. Resonant Frequency (FR ) and Cell Quality Factor (Q) Characterization
During aircraft operation, cell FR characterization is essential given that temperature and pressure change as the aircraft changes altitude (Arnott et al. Citation2006). Characterization is commonly performed by scanning across the expected region of resonance with a noise generator (e.g., speaker), which can take several to tens of seconds to complete. Keeping the laser modulated at FR is critical in order to maintain calibration, and so we have implemented a fast method for determining FR (similar to Arnott et al. Citation1999) A 1 s constant-power acoustic ‘chirp’ covering a broad range of frequencies in the region of interest (expected FR ± 200 Hz) is introduced via the speaker. In the frequency domain, the microphone-detected chirp appears as a Gaussian that can be fitted to extract the cell FR and Q (). For a 1 s calibration, the resolution of FR is 1 Hz. This determination is automatically done at the beginning of every filter cycle. During flight operation a speaker duty cycle of 320 s is typically used, although this parameter can be adjusted or alternatively the user can initiate calibrations manually at any time. After characterization, the modulation frequency of the laser power is adjusted to the measured FR .
3.2. Pressure and Temperature Dependencies
Laboratory tests were performed to determine the sensitivity of this system to pressure and temperature changes. The system pressure and temperature were adjusted to cover ranges likely to be observed on a research aircraft. Figure 3 shows the FR , Q, resonator time constant (Q/FR ), and the speaker amplitude as a function of pressure and temperature for one cell. As expected, FR is most sensitive to temperature changes (at ∼3 Hz K−1) illustrating the importance of the PAS cell temperature stabilization. In contrast, the sensitivity of FR to pressure changes is relatively small at ∼7.5×10−2 Hz kPa−1. The sensitivity of FR to operating conditions shown here indicates the importance of performing regular FR characterizations during operation on an aircraft.
3.3. Measurement Calibration
Absorption (b Abs ) can be determined from PAS measurements via a first principles calibration using Equationequation 2 (Arnott et al. Citation2006; Havey et al. Citation2010; Ajtai et al. Citation2010):
FIG. 4 Steps involved in the calibration of PAS flight data. (a) Pressure dependence of PAS cell time constant (Q/FR) measured during preflight (diamonds), in-flight (dots), polynomial fit to preflight data (black dashed line), and pressure correction factor relative to calibration pressure (circles). (b) Removal of background contributions from the measured PAS microphone signal. (c) Calibration regression for a gas phase calibration at 532 nm using ozone. (d) Final 1 Hz resolution, pressure-corrected absorption data (babs ) at STP and 532 nm wavelength.
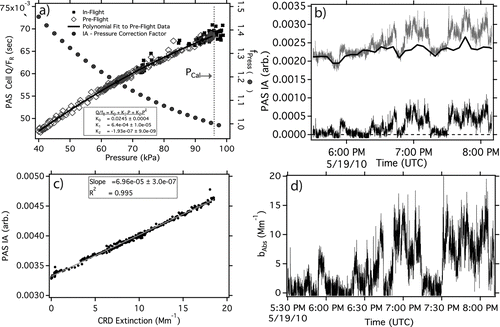
3.3.1. Microphone Calibration
The microphones are calibrated by correlating the microphone response (IA) to known absorption levels of ozone (Lack et al. Citation2006) quantified through concurrent CRDS extinction measurements (Langridge et al. Citation2011). O3 is introduced simultaneously into the CRDS and PAS cells in a parallel flow configuration. Gas phase absorption of O3 is measured by the CRDS at 662 nm, 532 nm, and 405 nm, and is used to calibrate the PAS 659 nm, 532 nm, and 404 nm channels. O3 cross-section differences for the 662 nm/659 nm (405.5 nm/403.6 nm) pairs differ by 0.5% (4%) (Burrows et al. Citation1999; Axson et al. Citation2011) and, therefore, no correction (small correction) is applied to the CRDS 662 nm (405.5 nm) measurements to account for wavelength differences. Photolysis measurements were made by running O3 in series through a 532 nm CRDS cell, 404 nm PAS cell and finally another 532 nm CRDS cell. No photolysis was observed as P Laser was cycled on and off and reduced.
NO2 can also be used to calibrate the 404 nm channels (although were not used for the 404 nm data presented here). The CRDS data must be scaled by 10% to account for the differences in the NO2 cross section at the CRDS and PAS wavelengths (Vandaele et al. Citation1998; Langridge et al. Citation2011). Photolysis of NO2 at 404 nm does occur within the PAS cell, due to the high density of 404 nm photons available. Photolysis is insignificant in the CRDS cells due to very low power levels. Photolysis is dependent on the exposure of NO2 to 404 nm photons, which is dependent on P Laser and residence time in the PAS cell. The photolysis factor is linear with P Laser at a specific flow rate (the same flow rate is always used for calibration). The CRDS measured extinction during NO2 calibrations is reduced by this photolysis factor. The photolysis factor for NO2 introduces an additional 5% uncertainty to the 404 nm measurements.
This calibration approach relies on the CRDS and PAS cells sampling equal concentrations of O3 or NO2. This assumption was validated through laboratory experiments which showed losses of <1% for both species when flowed through an assembly consisting of two CRDS cells arranged either side of a single PAS cell. To further test losses, a single PAS cell was filled with NO2 or O3 and then sealed while the temporal decay in IA at 532 nm was monitored. Analysis of the decay rate suggested losses of <1% for both species for flow rates used in the current system. gives an example of an O3 calibration at 532 nm. It must be noted that absorption cross-sections of NO2 at 404 nm, 532 nm, and 659 nm, (Vandaele et al. Citation1998) and by NO3 (Orphal et al. Citation2003) at 659 nm are significant (5.69e-19 cm2, 1.5×10−19 cm2, 6.81×10−21 cm2, and 3.43×10−18 cm2, respectively) and can cause significant interference. It is, therefore, critical that O3 calibrations are performed using high-grade zero-air or oxygen to eliminate the potential for interference.
3.3.2. Pressure Dependency Calibration
During preflight zero air is introduced into the sample line while the speakers in each cell are activated to provide continuous measurement of FR and Q as the pressure of the system is reduced from ambient (∼100 kPa) to about 40 kPa using a needle valve. These measurements are used to correct the pressure dependencies of the PAS cells.
Equivalent b Abs levels at a reference pressure (ref-P) and any other pressure (P) are related by EquationEquation (3):
We fit a third order polynomial function to preflight Q/FR data (, grey diamonds), which is validated by in-flight Q/FR data (, black stars), which is collected when the speaker calibration is activated at the start of each of the particle filter periods (every 5 min). The pressure at which the microphone calibration is performed is used as a reference pressure (P Cal) and f Press is calculated using the polynomial fit information (, black dots). PAS measured IA across the flight is then corrected to P Cal using f Press.
3.3.3. Postprocessing
The procedure used for converting in-flight IA measurements to aerosol absorption values incorporates information from each of the calibration steps described above. It proceeds as follows:
1. | IA measured in flight is normalized to P Cal using the f Press function measured preflight (Section 3.3.2). The standard deviation in this fit is propagated through to the final calculated absorption. | ||||
2. | IA measured during filter periods is smoothed to 10-s, linearly interpolated across the duration of the flight (black line, ) and subtracted from the sample IA (grey data, ) to produce the background corrected IA signal (black data, ). This background subtraction accounts for long-term changes in gas-phase absorbers. Short-term changes are removed in step 4. | ||||
3. | The microphone calibration slope from gas-phase measurement data (Section 3.3.1) is used to calculate absorption from the background-subtracted IA data, which are then converted to STP (100 kPa, 273 K) (). The standard deviation in this calibration is used for uncertainty propagation. | ||||
4. | Gas phase extinction data measured by the CRDS (Langridge et al. Citation2011) between filter periods are convolved to the cell residence time of the PAS and subtracted from the PAS absorption. This step is only undertaken for gas phase absorption exceeding 0.5 Mm−1. For all flights during the CalNex field mission, gas phase interference at 659 nm was insignificant and PAS data did not require short-term correction. In addition, the thermo-denuder successfully removed all gas phase interference from the thermo-denuded 532 nm and 404 nm signals. Dry 532 nm and 404 nm PAS absorption sometimes required between-filter period gas phase corrections, mostly during urban pollution events. During these events, gas phase absorption at 532 nm (404 nm) contributed on average ∼10% (∼35%) of PAS-measured absorption. Future development will incorporate a NO2 and O3 scrubber to avoid the need for gas phase subtraction. |
3.4. Systematic Uncertainties
Systematic uncertainties in the measured absorption include (a) the fit to the pressure dependency of IA () and (b) the fit to the microphone calibration slope (). The calibration slope uncertainty is affected by the magnitude of the measured absorption. In the laboratory, O3 and NO2 can be introduced at high enough concentrations to minimize the microphone calibration uncertainty. During the CalNex field campaign this was not always the case due to limitations of in-field resources. Fit uncertainties are added point by point in the data processing steps. Ground-based data have a systematic uncertainty, of no more than ±5% for all wavelengths and channels. This uncertainty is confirmed experimentally (for 532 nm only) in Section 4.1. For aircraft-based measurement the average of point-by-point uncertainties from over 20 flights of data were less than ±10%, due to addition of the pressure dependency of IA. An additional absolute uncertainty of ∼±0.5 Mm−1 is generated through the background subtraction although this is dependent on the signal to noise ratio of each flight.
TABLE 2 1 Hz sensitivity and uncertainties of the five PAS channels
3.5. Sensitivity
The sensitivity of each wavelength was calculated by measuring the 2-sigma deviation around the mean for IA measured on zero air (or free troposphere filtered air for in-flight) (). For laboratory (surface) conditions the sensitivities at 659 nm, 532 nm, and 404 nm (including thermo-denuded channels) were 0.4, 0.15, and 0.2 Mm−1, respectively for 1 Hz data; the best sensitivity performance under flight conditions for 1 Hz data was 1.5, 0.75, and 0.5 Mm−1, respectively. Average sensitivity performance, for dry absorption at 659 nm, 532 nm, and 404 nm under flight conditions for 1 Hz data was 2.0, 1.2, and 1.1 Mm−1, respectively. The thermo-denuder appeared to introduce added noise in flight, possibly due to inadequate mechanical isolation. For thermo-denuded 532 nm and 404 nm channels the average sensitivity was 1.5 Mm−1. Deviations from sensitivities reported above resulted periodically from extreme aircraft noise, most often brought about by sharp turns and steep ascents of the aircraft.
TABLE 3 A comparison of the measured (σAbs-meas ) and calibrated (σAbs-cal ) values of absorption cross section at 532 nm for APSS
4. RESULTS
4.1. Laboratory Comparisons—Calibrated Absorbing Spheres
The absorption cross-sections (σAbs ) of absorbing polystyrene spheres (APSS) were measured (σAbs-Meas ) and compared to the σAbs calibrated (σAbs-Cal ) using our previous PAS instrument (Lack et al. Citation2006; Lack et al. Citation2009). Two sizes of APSS were run through the new PAS system and results were in very good agreement with previous measurements (). The APSS were calibrated at 532 nm only and, therefore, no comparison at other wavelengths was possible.
4.2. Field Deployment
4.2.1. Example Temporal and Vertical Profiles
Here we show 1 Hz PAS data from a research flight to illustrate the in-flight capabilities. On the 19th of May 2010, during the CalNex field campaign, the 5-channel PAS instrument flew onboard the NOAA WP-3D research aircraft over Los Angeles. shows ∼90 min of 1 Hz data for the gas-phase corrected dry absorption at each of the measurement wavelengths. This figure also shows the wavelength dependent absorption angstrom exponent (AAE) smoothed to 10 s resolution (and only where absolute absorption was above background levels). The AAE is calculated as the slope of the regression between the plot of log(absorption) versus log(wavelength) (Moosmuller et al. Citation2011). Here we use all three absorption wavelengths to calculate AAE404-532-659. Uncertainty in AAE404-532-659 is calculated as the measurement accuracy propagated in quadrature. and c show 3-wavelength absorption and AAE, respectively, for a vertical profile during the flight, while shows the PAS comparison to particle soot absorption photometer (PSAP) measured absorption for that flight. PSAP to PAS regressions for 659 nm, 532 nm, and 404 nm are 1.02, 1.03, and 1.06, respectively. PSAP data were corrected using the procedures of Virkkula (Citation2010) and Virkkula et al. (Citation2005), while PSAP data at 469 nm was converted to 404 nm using an AAE of 1. We did not use the PSAP-measured AAE for conversion to avoid introducing a significant noise source into the 404 nm PSAP data. An AAE of 1 does introduce some uncertainty that may account for some of the difference between the PSAP and PAS regression. However, for brief comparison purposes, an AAE of 1 is sufficient.
FIG. 5 Example of 1 Hz (a) absorption time series (90 min) [absorption Angstrom exponent (AAE404–532–659) smoothed to 1 min also shown]. (b) Vertical profile absorption data for 659 nm (red), 532 nm (green), and 404 nm (blue). (c) Vertical profile AAE404–532–659 (1 Hz). (d) Comparison of PSAP to PAS measured absorption. 467 nm PSAP absorption converted to 404 nm using AAE = 1.
![FIG. 5 Example of 1 Hz (a) absorption time series (90 min) [absorption Angstrom exponent (AAE404–532–659) smoothed to 1 min also shown]. (b) Vertical profile absorption data for 659 nm (red), 532 nm (green), and 404 nm (blue). (c) Vertical profile AAE404–532–659 (1 Hz). (d) Comparison of PSAP to PAS measured absorption. 467 nm PSAP absorption converted to 404 nm using AAE = 1.](/cms/asset/437ffb7d-79a3-454c-8cbc-83320bbe34c2/uast_a_645955_o_f0005g.jpg)
4.2.2. Example Absorption Enhancement Due to Coatings
Here we demonstrate the capabilities of the PAS to measure absorption enhancement (EAbs ) created by clear coatings on BC cores, or added absorption due to BrC and EAbs on BC cores (Lack and Cappa Citation2010). EAbs can be calculated from the ratio of the dry absorption and the thermo-denuded absorption assuming a complete internal mixture. This process assumes that the non-BC coatings are entirely removed from the particles and that pyrolysis of non-BC material does not occur; therefore the measured EAbs will be a lower bound on the actual EAbs . During the CalNex field campaign dry ambient air was passed through the thermo-denuder on multiple flights. shows the measured absorption (1 Hz data) and the calculated EAbs for 532 nm and 404 nm (smoothed to 1 min). Due to the low signal-to-noise at the absorption levels measured the smoothed calculated EAbs contains significant variability. In periods that led to an EAbs < 0 were removed for clarity of the figure. The EAbs for 532 nm shows values ranging from ∼0.7 and up to ∼1.2. Considering the signal to noise and uncertainties of the measurements this indicates minimal enhancement due to coatings and possibly small issues regarding the removal of gas phase interference (creating EAbs < 1). At 404 nm, however, EAbs values of up to 2.5 were observed. As discussed in Lack and Cappa (Citation2010) this EABS value may include contributions from either an enhancement from clear coatings or absorption of BrC. Utilizing these, and other measurements to elucidate the contributions of BrC to absorption will be the subject of a subsequent manuscript.
FIG. 6 Dry and thermo-denuded 532 nm and 404 nm 1 Hz absorption (left axis). Calculated absorption enhancement (EAbs ) at 532 nm and 405 nm shown in red on the right axis (smoothed to 1 min). Uncertainties in EAbs calculated through propagation of measurement accuracy. EAbs of 1 (i.e., no enhancement) shown as gray line.
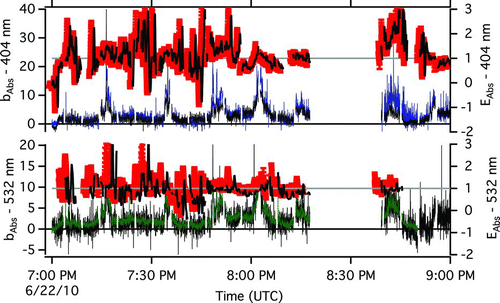
FIG. 7 1 Hz extinction, absorption, and derived parameters from the combination of the PAS and CRDS systems from the companion paper of Langridge et al. (Citation2011).
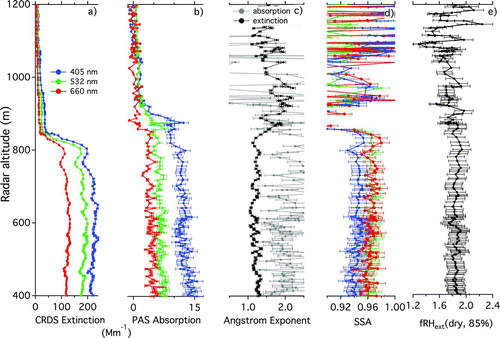
4.2.3. Data Summary from Combined PAS Absorption and CRDS Extinction Instruments
A companion paper (Langridge et al. Citation2011) describes the development of a CRDS system for measuring multiwavelength and multi-RH aerosol extinction. shows a vertical profile of a selection of data measured and derived from the CRDS and PAS instruments during a Calnex flight. During this vertical profile, aerosol extinction was high (170–200 Mm−1 @ 532nm) ( panel a) while absorption was relatively low at 6–8 Mm−1 ( panel b), yielding aerosol single scatter albedos (SSA = [extinction - absorption]/extinction) of 0.96–0.97 ( panel d). The wavelength dependence (or Angstrom exponents) for extinction and absorption are also shown in panel c. The SSA as a function of wavelength is one of the primary determinants for the direct radiative impact of aerosols. Aerosol extinction RH dependence (fRHExt) is an additional parameter required to determine the change in optical extinction as a particle swells from dry to elevated RH (in this case ∼15% to 85%). panel e shows fRHExt of 1.6–1.8 for the example vertical profile, indicating 60–80% increase in extinction from 15–85% RH. Above 825 meters (top of the boundary layer) the pollution layer was substantially reduced, pushing the PAS near its signal-to-noise limit for 1 Hz data, causing the significant variability in derived AAE and SSA observed. In regions where these low signal level parameters are required with better certainty, averaging of the 1 Hz data would be necessary. These profiles show the utility of the multiwavelength, multi-RH capability of the combined instruments. Further assessment of the data from both instruments will be the subject of future manuscripts.
5. SUMMARY
The radiative impact of absorbing black carbon (BC) aerosol is complicated by the addition of coatings of organic and inorganic material on BC and an emerging understanding that some organic aerosol may also absorb light (brown carbon, BrC). Inaccuracies and biases in traditional measurement techniques and lack of high quality spatial and temporal profiles contribute to the uncertainty in the radiative impacts of absorbing aerosol. Here we have described and demonstrated the utility of a three wavelength, 5-channel photo-acoustic absorption spectrometer (PAS) that is capable of sensitive measurement of absorption on a research aircraft. This instrument will contribute to reducing the uncertainties in the measurement of the absorption of BC, BrC, and the effect of coatings on absorption of BC. The instrument is capable of measuring vertical profiles of 659 nm, 532 nm, and 404 nm dry absorption and the absorption enhancement due to semivolatile coatings on BC at 532 nm and 404 nm. In-flight sensitivity of dry absorption for 1 Hz resolution was determined to be, at best, 1.5 Mm−1, 0.75 Mm−1, and 0.5 Mm−1 for 659 nm, 532 nm, and 404 nm, respectively. This sensitivity is adequate for most measurements in a research aircraft environment. However, future development goals of 0.1 Mm−1 sensitivity at 1 Hz have been set to obtain absorption measurements comparable to the sensitivity of the laser incandescence-based measurement of BC mass. Laser power is likely the most productive path toward achieving this goal. The estimated in-flight accuracy was determined to be ≤±10% for all channels. The combination of high laser power (via an astigmatic mirror multipass cell) and mechanical vibration isolation allowed for such good sensitivity. The use of the matched wavelength aerosol extinction from a new cavity ring down spectrometer (CRDS, Langridge et al. 2011) for calibration purposes provides accurate empirical calibrations. The instrument was compared to calibrated absorbing polystyrene spheres and was found to be within ±4% of the calibrated standard. In-flight data compared to filter-based absorption showed acceptable correlation and absolute agreement when uncertainties of both instruments are considered. The instrument design does not allow for absolute laser power measurement thus restricting the use of the first principles theoretical PAS calibration. However, future development of this instrument will include application of the first principles calibration, in addition to higher power lasers, reduced flow noise and additional vibration/noise isolation. The choice of laser wavelengths and sample treatment within the five channels (a restriction due to weight and space restrictions) will also be investigated for optimal information retrieval (e.g., thermal-denuded 659 nm). The combination of the high sensitivity, high time resolution PAS absorption and CRDS extinction will allow for unprecedented 4-dimensional analysis (altitude latitude, longitude, and time) of the direct radiative impact of atmospheric aerosol.
Acknowledgments
This research was funded by NOAAs Climate Program. The authors thank the NOAA Air Operations Center and crews of the WP-3D aircraft for the aircraft frequency measurements and operation during the CalNex campaign.
Notes
1. Certain commercial equipment, instruments, or materials are identified in this article in order to adequately specify the experimental procedure. Such identification does not imply recognition or endorsement by the National Oceanic and Atmospheric Administration, nor does it imply that the material or equipment identified is necessarily the best available for the purpose.
REFERENCES
- Ajtai , T. , Filep , A. , Schnaiter , M. , Linke , C. , Vragel , M. Bozóki , Z. 2010 . A Novel Multi-wavelength Photoacoustic Spectrometer for the Measurement of the UV-Vis-NIR Spectral Absorption Coefficient of Atmospheric Aerosols . J. Aerosol. Sci. , 41 : 1020 – 1029 . doi:10.1016/j.jaerosci.2010.07.008
- Arnott , W. P. , Moosmuller , H. , Rogers , C. F. , Jin , T. F. and Bruch , R. 1999 . Photoacoustic Spectrometer for Measuring Light Absorption by Aerosol: Instrument Description . Atmos. Environ. , 33 : 2845 – 2852 .
- Arnott , W. P. , Walker , J. W. , Moosmuller , H. , Elleman , R. A. , Jonsson , H. H. Buzorius , G. 2006 . Photoacoustic Insight for Aerosol Light Absorption Aloft from Meteorological Aircraft and Comparison with Particle Soot Absorption Photometer Measurements: DOE Southern Great Plains Climate Research Facility and the Coastal Stratocumulus Imposed Perturbation Experiments . J. Geophys. Res. , 111 : D05S02 10.1029/2005jd005964
- Axson , J. L. , Washenfelder , R. A. , Kahan , T. F. , Young , C. J. , Vaida , V. and Brown , S. S. 2011 . Absolute Ozone Absorption Cross Section in the Huggins Chappuis Minimum (350Äì470 nm) at 296 K . Atmos. Chem. Phys. Discuss. , 11 : 21655 – 21676 . 10.5194/acpd-11-21655-2011
- Barnard , J. C. , Volkamer , R. and Kassianov , E. I. 2008 . Estimation of the Mass Absorption Cross Section of the Organic Carbon Component of Aerosols in the Mexico City Metropolitan Area . Atmos. Chem. Phys. , 8 : 6665 – 6679 .
- Bond , T. , Habib , G. and Bergstrom , R. W. 2006 . Limitations in the Enhancement of Visible Light Absorption Due to Mixing State . J. Geophys. Res. , 111 10.1080/02786820500421521
- Boparai , P. , Lee , J. and Bond , T. C. 2008 . Revisiting Thermal-Optical Analyses of Carbonaceous Aerosol Using a Physical Model . Aer. Sci. Tech. , 42 : 930 – 948 . doi: 10.1080/02786820802360690
- Burrows , J. P. , Richter , A. , Dehn , A. , Deters , B. , Himmelmann , S. , Voigt , S. and Orphal , J. 1999 . Atmospheric Remote-sensing Reference Data from GOME - 2. Temperature-dependent Absorption Cross Sections of O-3 in the 231-794 nm Range . J. Quant. Spectrosc. Ra. , 61 : 509 – 517 .
- Chakrabarty , R. K. , Moosmuller , H. , Chen , L. W. A. , Lewis , K. , Arnott , W. P. Mazzoleni , C. 2010 . Brown Carbon in Tar Balls from Smoldering Biomass Combustion . Atmos. Chem. Phys. , 10 : 6363 – 6370 . 10.5194/acp-10-6363-2010
- Doherty , S. J. , Warren , S. G. , Grenfell , T. C. , Clarke , A. D. and Brandt , R E . 2010 . Light-absorbing Impurities in Arctic Snow . Atmos. Chem. Phys. , 10 : 11647 – 11680 . 10.5194/acp-10-11647-2010
- Fierz , M. , Vernooij , M. G. C. and Burtscher , H. 2007 . An Improved Low-flow Thermodenuder . J. Aerosol. Sci. , 38 : 1163 – 1168 . doi:10.1016/j.jaerosci.2007.08.006
- Flanner , M. G. , Zender , C. S. , Randerson , J. T. and Rasch , P. J. 2007 . Present-day Climate Forcing and Response from Black Carbon in Snow . J. Geophys. Res. , 112 doi:10.1029/2006JD008003
- Gillis , K. A. , Havey , D. K. and Hodges , J. T. 2010 . Standard Photoacoustic Spectrometer: Model and Validation Using O2 A-band Spectra . Rev. Sci. Inst. , 81 doi:10.1063/1061.3436660
- Havey , D. K. , Bueno , P. A. , Gillis , K. A. , Hodges , J. T. , Mulholland , G. W. van Zee , R. D. 2010 . Photoacoustic Spectrometer with a Calculable Ccell Constant for Measurements of Gases and Aerosols . Anal. Chem. , 82 : 7935 – 7942 . 10.1021/ac101366e
- Hildebrand , P. H. and Sekhon , R. S. 1974 . Objective Determination of the Noise Level of Doppler Spectra . J. Applied Meteor , 13 : 808 – 811 .
- IPCC . 2007 . Climate Change 2007: The Physical Science Basis. Contribution of Working Group 1 to the Fourth Assessment Report . Report of the intergovernmental panel on climate change (Assesment Report 4)
- Jacobson , M. Z. 2001 . Strong Radiative Heating Due to the Mixing State of Black Carbon in Atmospheric Aerosols . Nature , 409 : 695 – 697 .
- Koch , D. and Del Genio , A. D. 2010 . Black Carbon Semi-direct Effects on Cloud Cover: Review and Synthesis . Atmos. Chem. Phys. , 10 : 7685 – 7696 . 10.5194/acp-10-7685-2010
- Lack , D. , Lovejoy , E. , Baynard , T. , Pettersson , A. and Ravishankara , A. 2006 . Aerosol Absorption Measurement Using Photoacoustic Spectroscopy: Sensitivity, Calibration, and Uncertainty Developments . Aerosol. Sci. Tech. , 40 : 697 – 708 .
- Lack , D. A. and Cappa , C. D. 2010 . Impact of Brown and Clear Carbon on Light Absorption Enhancement, Single Scatter Albedo and Absorption Wavelength Dependence of Black Carbon . Atmos. Chem. Phys. , 10 : 4207 – 4220 .
- Lack , D. A. , Cappa , C. D. , Covert , D. S. , Baynard , T. , Massoli , P. Sierau , B. 2008 . Bias in Filter Based Aerosol Light Absorption Measurements Due to Organic Aerosol Loading: Evidence from Ambient Measurements . Aerosol. Sci. Tech. , 42 : 1033 – 1041 .
- Lack , D. A. , Cappa , C. D. , Cross , E. S. , Massoli , P. , Ahern , A. Davidovits , P. 2009 . Absorption Enhancement of Coated Absorbing Aerosols: Validation of the Photo-Acoustic Technique for Measuring the Enhancement . Aerosol. Sci. Tech. , 43 : 1006 – 1012 .
- Langridge , J. , Richardson , M. , Law , D. , Lack , D. A. and Murphy , D. M. 2011 . Aircraft Instrument for Comprehensive Characterisation of Aerosol Optical Properties, Part I: Wavelength Dependent Optical Extinction and Its Relative Humidity dependence Measured Using Cavity Ringdown Spectroscopy . Aerosol. Sci. Tech , 45 : 1305 – 1318 .
- Lewis , K. , Arnott , W. P. , Moosmuller , H. and Wold , C. E. 2008 . Strong Spectral Variation of Biomass Smoke Light Absorption and Single Scattering Albedo Observed with a Novel Dual-wavelength Photoacoustic Instrument . J. Geophys. Res. , 113 : D16203 10.1029/2007jd009699
- McManus , J. B. , Kebabian , P. L. and Zahniser , M. S. 1995 . Astigmatic Mirror Multipass Absorption Cells for Long-path-length Spectroscopy . Appl. Optics , 34 : 3336 – 3348 .
- Moosmuller , H. , Chakrabarty , R. K. and Arnott , W. P. 2009 . Aerosol Light Absorption and Its Measurement: A Review . J. Quant. Spectros. Radiat. Transfer , 110 : 844 – 878 .
- Moosmuller , H. , Chakrabarty , R. K. , Ehlers , K. M. and Arnott , W. P. 2011 . Absorption Angstrom Coefficient, Brown Carbon, and Aerosols: Basic Concepts, Bulk Matter, and Spherical Particles . Atmos. Chem. Phys. , 11 : 1217 – 1225 . 10.5194/acp-11-1217-2011
- Muller , T. , Henzing , J. S. , de Leeuw , G. , Wiedensohler , A. , Alastuey , A. and Angelov , H. 2011 . Characterization and Intercomparison of Aerosol Absorption Photometers: Result of Two Intercomparison Workshops . Atmos. Meas. Tech. , 4 : 245 – 268 . 10.5194/amt-4-245-2011
- Murphy , D. M. 2009 . The Effect of Water Evaporation on Photoacoustic Signals in Transition and Molecular Flow . Aerosol. Sci. Tech. , 43 : 356 – 363 .
- Nägele , M. and Sigrist , M. W. 2000 . Mobile Laser Spectrometer with Novel Resonant Multipass Photoacoustic Cell for Trace-gas Sensing . Appl. Phys. B-Lasers O , 70 : 895 – 901 . 10.1007/s003400050026
- Nakayama , T. , Kondo , Y. , Moteki , N. , Sahu , L. K. , Kinase , T. and Kita , T. 2010 . Size-dependent Correction Factors for Absorption Measurements Using Filter-based Photometers: PSAP and COSMOS . J. Aerosol. Sci. , 41 : 333 – 343 .
- Orphal , J. , Fellows , C. E. and Flaud , P. M. 2003 . The Visible Absorption Spectrum of NO3 Measured by High-resolution Fourier Transform Spectroscopy . J. Geophys. Res. , 108 : 4077
- Quinn , P. K. , Bates , T. S. , Coffman , D. , Onasch , T. B. , Worsnop , D. Baynard , T. 2006 . Impacts of Sources and Aging on Submicrometer Aerosol Properties in the Marine Boundary Layer Across the Gulf of Maine . J. Geophys. Res. , 111 10.1029/2006jd007582
- Ramanathan , V. and Carmichael , G. 2008 . Global and Regional Climate Changes Due to Black Carbon . Nature Geosci. , 1 : 221 – 227 .
- Ramanathan , V. , Ramana , M. V. , Roberts , G. , Kim , D. , Corrigan , C. and Chung , C. 2007 . Warming Trends in Asia Amplified by Brown Cloud Solar Absorption . Nature , 448 : 575 – 578 .
- Raspet , R. , Slaton , W. , Arnott , W. P. and Moosmuller , H. 2003 . Evaporation-Condenstion Effects on Resonant Photoacoustics of Volatile Aerosols . Journal of Atmospheric and Oceanic Technology , 20 : 685
- Schmid , B. , Livingston , J. M. , Russell , P. B. , Durkee , P. A. , Jonsson , H. H. Collins , R. C. F. 2000 . Clear-sky Closure Studies of Lower Tropospheric Aerosol and Water Vapor During ACE-2 Using Airborne Sunphotometer, Airborne In-Situ, Space-Borne, and Ground-Based Measurements . Tellus B , 52 : 568 – 593 .
- Schnaiter , M. , Linke , M. , Möhler , O. , Naumann , K.-H. , Saathoff , H. R. Wagner , R. 2005 . Absorption Amplification of Black Carbon Internally Mixed with Secondary Organic Aerosol . J. Geophys. Res. , 110 doi:10.1029/2005JD006046
- Schwarz , J. P. , Gao , R. S. , Spackman , J. R. , Watts , L. A. , Thomson , D. S. Fahey , D. W. 2008a . Measurement of the Mixing State, Mass, and Optical Size of Individual Black Carbon Particles in Urban and Biomass Burning Emissions . Geophys. Res. Lett. , 35 doi:10.1029/2008GL033968
- Schwarz , J. P. , Spackman , J. R. , Fahey , D. W. , Gao , R. S. , Lohmann , U. Watts , L. A. 2008b . Coatings and Their Enhancement of Black Carbon Light Absorption in the Tropical Atmosphere . J. Geophys. Res. , 113 doi:10.1029/2007JD009042
- Schwarz , J. P. , Spackman , J. R. , Gao , R. S. , Watts , L. A. , Stier , P. Schulz , M. 2010 . Global-scale Black Carbon Profiles Observed in the Remote Atmosphere and Compared to Models . Geophys. Res. Lett. , 37 : L18812 10.1029/2010gl044372
- Timko , M. T. , Yu , Z. , Kroll , J. , Jayne , J. T. , Worsnop , D. R. Miake-Lye , R. C. 2009 . Sampling Artifacts from Conductive Silicone Tubing . Aerosol. Sci. Tech. , 43 : 855 – 865 .
- Vandaele , A. C. , Hermans , C. , Simon , P. C. , Carleer , M. , Colin , R. Fally , S. 1998 . Measurements of the NO2 Absorption Cross-section from 42 000 cm−1 to 10 000 cm−1 (238–1000 nm) at 220 K and 294 K . J. Quant. Spectrosc. Ra. , 59 : 171 – 184 .
- Virkkula , A. 2010 . Correction of the Calibration of the 3-wavelength Particle Soot Absorption Photometer (3Œa PSAP) . Aerosol Sci. Technol. , 44 : 706 – 712 .
- Virkkula , A. , Ahlquist , N. C. , Covert , D. S. , Arnott , W. P. , Sheridan , P. J. Quinn , P. K. 2005 . Modification, Calibration and a Field Test of an Instrument for Measuring Light Absorption by Particles . Aerosol. Sci. Tech. , 39 : 68 – 83 .
- von der Weiden , S. L. , Drewnick , F. and Borrmann , S. 2009 . Particle Loss Calculator - A New Software Tool for the Assessment of the Performance of Aerosol Inlet Systems . Atmos. Meas. Tech. , 2 : 479 – 494 .
- Zarzycki , C. M. and Bond , T. C. 2010 . How Much can the Vertical Distribution of Black Carbon Affect Its Global Direct Radiative Forcing? . Geophys. Res. Lett. , 37 : L20807 10.1029/2010gl044555
- Zhang , Q. , Jimenez , J. L. , Canagaratan , M. R. , Allan , J. D. , Coe , H. Ulbrich , I. 2007 . Ubiquity and Dominance of Oxygenated Species in Organic Aerosols in Anthropogenically-influenced Northern Hemisphere Midlatitudes . Geophys. Res. Lett. , 34 doi:10.1029/2007GL029979
- Zhang , R. , Khalizov , A. F. , Pagels , J. , Zhang , D. , Xue , H. and McMurry , P. H. 2008 . Variability in Morphology, Hygroscopicity, and Optical Properties of Soot Aerosols During Atmospheric Processing . PNAS , 105 : 10291 – 10296 .