Abstract
In this study, the characteristic time scale of black carbon (BC) aging due to condensation of hygroscopic species was parameterized as a function of the condensing species concentration and the size distribution of BC particles. The aging time scale defined based on the BC mass concentration was shown to increase with BC particle size as well as with polydispersity of the size distribution. The effects of the BC particle size distribution on the aging time scale are shown to be considerable in the continuum regime, whereas the aging time scale tends to converge to a constant value in the free-molecule regime. The polydispersity effect was shown to be significant except for extraordinarily small particles. When the aging time scale was defined based on the BC number concentration, the dependency of the aging time scale on polydispersity is much smaller because the effect of slow aging of largest particles is compensated by fast aging of smallest particles. The aging time scale was also shown to be a strong function of the condensing species concentration. The result of this study implies that the aging time scale parameterizations suggested in the previous studies, which did not take into account the effects of condensing species concentration and particle size distribution, cannot be easily generalized for being applied to global model simulations.
Copyright 2012 American Association for Aerosol Research
1. INTRODUCTION
The aerosol effect on climate change has drawn attention of a number of researchers over the last decade. Atmospheric aerosol particles change the amount of solar radiation arriving at the earth surface by scattering or absorbing sunlight, which is called the direct effect. Aerosol particles composed of hygroscopiccomponents can act as cloud condensation nuclei (CCNs). Increase in the number of CCNs under limited amount of atmospheric water vapor results in smaller cloud drop size, larger number of cloud drops, reduced precipitation, higher cloud albedo, and longer cloud lifetime, counteracting global warming, which is called the indirect effect (Albrecht Citation1989; Rosenfeld Citation2000; Ramanathan et al. Citation2001; Kaufman et al. Citation2002; Lohmann and Feichter Citation2005). The direct and indirect effects of aerosol particles depend on their optical properties and hygroscopicity that are determined by the chemical composition. It is crucial to quantify the direct and indirect aerosol effects to predict the future climate accurately. The aerosol effect, however, accounts for the largest uncertainty in assessment of the anthropogenic influences on climate change (IPCC [Intergovernmental Panel on Climate Change] Citation2007).
Black carbon (BC) is a byproduct of combustion of fossil fuels and biomass burning and is an indicator of diesel particulate matter in urban areas (Bae et al. Citation2007). BC is the component accounting for a significant part of the uncertainty of the aerosol climate impact. BC imposes positive radiative forcing by absorbing sunlight (Ech et al. Citation1998; Satheesh and Ramanathan Citation2000), resulting in increase of atmospheric temperature (direct effect), contrary to the negative radiative forcing caused by hygroscopic aerosol species such as sulfate that scatter sunlight. The optical properties of a BC-containing aerosol, which determine the direct effect of BC, are dependent on its mixing state. According to computations based on Mie theory, BC reveals much larger absorbance when it is mixed internally with other aerosol species than when it exists in an external mixture (Ackerman and Toon Citation1981; Chylek et al. Citation1995; Martins et al. Citation1998; Haywood and Boucher Citation2000; Jacobson Citation2001; Lesins et al. Citation2002; Riemer et al. Citation2003; Bond et al. Citation2006). Based on his global modeling, Jacobson (Citation2000) argued that the positive radiative forcing of BC might offset the total negative radiative forcing caused by all other anthropogenic aerosol species.
BC particles acting as CCNs exert indirect effect similar to other aerosol species (Kaufman and Nakajima Citation1993; Kaufman and Fraser Citation1997; Rosenfeld Citation2000). However, there is an additional influence of BC on climate. Absorption of sunlight by in-cloud BC increases the cloud temperature and reduces the cloud cover by evaporating cloud drops (Hansen et al. Citation1997; Ackerman et al. Citation2000; Lohmann and Feichter Citation2001), which leads to additional positive radiative forcing. BC also enhances the atmospheric stability and hence suppresses cloud formation by reducing the lapse rate (Hansen et al. Citation1997; Ackerman et al. Citation2000), which may lead to changes in atmospheric circulation pattern and regional climate (Menon et al. Citation2002). On the other hand, BC existing above or below cloud layer can either increase or decrease cloud cover depending on circumstance. For example, below-cloud heating by BC can enhance vertical motions and increase cloud cover (McFarquhar and Wang Citation2006). When absorption of solar radiation by BC affects the cloud cover, it is collectively referred to as the semi-direct effect. Refer to Koch and Del Genio (Citation2010) for detailed review on the semi-direct effect of BC. The strength of indirect and semi-direct effects of BC is determined by the hygroscopicity of BC-containing particles. Fresh BC particles, which are externally mixed and hydrophobic (Weingartner et al. Citation1997), cannot participate in the cloud forming process. By the interactions with other species in the atmosphere, i.e., condensation of hygroscopic species molecules on BC particle surface, coagulation with hydrophilic particles, and heterogeneous oxidation reaction taking place on BC particle surface, BC transforms into internally mixed state and eventually takes part in cloud formation (Zuberi et al. Citation2005). Therefore, it is required to estimate the mixing state of BC to quantify the climate impact of BC.
The process in which externally mixed hydrophobic BC-containing particles are transformed into internally mixed hygroscopic state is called aging. In this paper, “aging of BC-containing particles” will be referred to as “aging of BC” for simplicity. Aging of BC has been investigated theoretically (Croft et al. Citation2005; Ramanathan and Carmichael Citation2008; Riemer et al. Citation2009; Ramana et al. Citation2010; Riemer et al. Citation2010; Zaveri et al. Citation2010) as well as experimentally (Saathoff et al. Citation2003; Slowik et al. Citation2007; Zhang et al. Citation2008; Khalizov et al. Citation2009; Pagels et al. Citation2009; Xue et al. Citation2009) or with observations (Johnson et al. Citation2005; Moteki et al. Citation2007; Shiraiwa et al. Citation2007; Wehner et al. Citation2009).
It should be noted that there is no unanimous agreement on the definition of the aging criterion in the literature. Aging criterion can be defined either as being capable of acting as a CCN (cloud-activity-based definition) or as containing enough soluble material to enhance absorption substantially (mixing-state-based definition). The former definition is suitable for modeling in-cloud scavenging, while the latter for quantifying mixing state and corresponding optical properties. These two definitions do not necessarily coincide: internally mixed BC may not always be cloud-active and, similarly, externally mixed BC is not always non-cloud-active because the cloud-activity is not determined solely by hygroscopicity but it is dependent also on particle size. It was shown in laboratory that smaller soot particles require a thicker coating of soluble material to be cloud-active than larger particles (Khalizov et al. Citation2009) because larger particles do not need to be as hygroscopic as smaller ones to be cloud-active. Both cloud-activity-based (Riemer et al. Citation2010) and mixing-state-based (Mann et al. Citation2010; Pringle et al. Citation2010) criteria have been used in recent studies. In this study, we use the mixing-state-based criterion because in most global atmospheric models incorporating the concept of aging, to which the result of this study is supposed to be applied, aging is used to move particles from an externally mixed group (mode or section) to an internally mixed group.
Inherently, aging of BC particles involves aerosol dynamics mechanisms such as condensation and coagulation. In most global atmospheric models, however, BC aging is simply neglected, i.e., BC is assumed to be either externally or internally mixed always, or is accounted for by simple parameterization instead of tracking the aerosol dynamics processes to reduce the computational cost (Textor et al. Citation2006). One method that is commonly used to take into account BC aging in global atmospheric modeling is to define the aging time scale as the characteristic time taken for externally mixed BC particles to transform into internally mixed ones and parameterize it appropriately. Because BC aging is the result of aerosol dynamics processes, the aging time scale should be dependent on the size distribution of BC particles. In the parameterization methods that are currently used, however, the size-distribution-dependency of the aging time scale is not taken into account explicitly (Riemer et al. (Citation2004; Park Citation2009). For a review on the global atmospheric models, refer to Textor et al. (Citation2006) and references therein.
In this paper, a parameterization for the aging time scale of BC particles due to condensation of hygroscopic molecules is derived as a function of the size distribution of BC particles as well as of the concentration of condensing species. The new parameterization is compared with previous ones and the implications of the new formula are discussed.
2. DERIVATION OF ANALYTICAL PARAMETERIZATION
2.1. Rate of BC Aging due to Condensation
Externally mixed BC particles age into internally mixed ones when they are “covered” by sufficient amount of hygroscopic species. Sulfuric acid, nitric acid, and oxidized organic species may be the three most important condensing species causing aging of BC particles (Riemer et al. Citation2004).
Vignati et al. (Citation2004), based on a comparison of their box model simulations with sophisticated sectional model simulations, suggested that the mass of a condensing species required to age an insoluble particle corresponds to a monolayer of the condensing species. They took into account only condensation of sulfuric acid, however, which could have significantly underestimated the number of coating layers if there were other condensable species as well. Most recent models assume that several monolayers of coating are required to fully age a particle. For example, Pringle et al. (Citation2010) used 5 monolayers for aging threshold, while Mann et al. (Citation2010) adopted 10-monolayer scheme. There is no firm agreement yet on exactly how many monolayers of coating are adequate as the criterion for the completion of aging. It may depend on the particle size and on the condensing material. Additional laboratory and field studies are needed on this issue. Therefore, we do not fix the number of monolayers at a constant value in our formulation in order that one can use it with whatever value one wants to.
Defining n(d BC) as the number size distribution of externally mixed BC particles where d BC is the BC particle diameter, the rate of aging due to condensation of a hygroscopic species can be expressed by
Under the assumption that Nc monolayers of coating are needed to age a BC particle, mth is
2.2. Aging Time Scale
Many models use globally constant aging time scale independent of time, location, atmospheric state, and BC particle size distribution (Cooke et al. Citation1999; Lohmann et al. Citation1999; Chung and Seinfeld Citation2002). Based on mesoscale modeling results, Riemer et al. (Citation2004) suggested aging time scales that depend on time and location. The aging time scale due to condensation of hygroscopic species was proposed to be 2 h above 250 m height from the surface and 8 h below. Park (Citation2009) derived the particle-size-dependent aging time scale under the assumption that all BC particles are of the same size, which is not applicable to realistic situations where particle size distribution is polydisperse. Mann et al. (Citation2010) and Pringle et al. (Citation2010) incorporated the particle-size-dependent aging process into their aerosol dynamics module, but they did not use the concept of “aging time scale.” To our knowledge, there has been no work that suggests the aging time scale as an explicit function of BC particle size distribution.
The aging time scale of BC particles with a polydisperse size distribution is defined as (Riemer et al. Citation2004)
In order to derive analytical expression for the numerator and the denominator of Equation (5), it is assumed in this study that the particle size distribution can be represented with a log-normal function. In general, this approach has been widely employed for representing the size distribution of aerosol particles both experimentally and theoretically. The log-normal size distribution function is given as
Then, Equation (5) can be rewritten as
Several semi-empirical methodologies, which are based on the so-called flux-matching theory, can be used to obtain the transition correction factor β. The general formula of the transition correction factor by the flux-matching theory is given as a function of Knudsen number, Kn (= 2λV/d BC), where λV is the mean free path of the condensing vapor. Among different flux-matching approaches, the harmonic mean method is the easiest one and has been widely used (Pratsinis Citation1988; Park and Lee Citation2000). It was shown by Park and Lee (Citation2000) that the harmonic mean is in good agreement with the approach of Fuchs and Sutugin (Citation1971), which is known to be almost universally valid (Monchick and Blackmore Citation1988), over the entire particle size range. Therefore, the harmonic mean method has been used in this study for the transition correction factor. For the details of the harmonic mean method and other flux-matching approaches, one can refer to Park and Lee (Citation2000).
In the harmonic mean method, the transition correction factor β is given by
For particles which are much smaller than the mean free path of the condensing vapor (d BC<<λV), i.e., in the free-molecule regime, Equation (12) is reduced into β fm =3αd BC/8λV and Equation (10) is converted into
On the other hand, for very large particles (d BC>>λV), i.e., in the continuum regime, β co =1 and Equation (10) is converted into
Using Equation (8), Equation (14) can be rewritten in terms of M 3, dg , and σ g as the following:
One can easily see that Equation (12) can be obtained again by taking harmonic mean of β
fm
and β
co
(). By applying the harmonic mean method to X, we obtain
3. DISCUSSION
shows the aging time scale due to condensation of a hygroscopic species calculated as a function of geometric mean BC particle diameter for three different polydispersity levels and two different accommodation coefficients. Throughout this section, Nc is set at 5 following the suggestion of Pringle et al. (Citation2010). Sulfuric acid is assumed to be the condensing species throughout this section. Although other condensable gaseous species such as nitric acid may also contribute to aging of BC as well as to aerosol burden, these are neglected in many global models. If their concentrations are available, however, inclusion of these species for calculating BC aging time scale is straightforward because the formulation presented in the previous section is applicable to any nonvolatile condensable species including nitrate. Also, many recent studies have shown that a large fraction of secondary organic aerosol is nonvolatile once condensed. Therefore, the approach of this study may potentially be applicable to secondary organic aerosol species, too. Condensation of semi-volatile organics may also contribute to BC aging to a certain extent. However, the result of this study may not be applicable to aging due to condensation of semi-volatile organics without knowledge on their saturation vapor pressure distribution, which is not obviously negligible. There is still a high uncertainty in the estimation of saturation vapor pressure distribution of semi-volatile organics and further intensive effort will be required to have a better estimation on it, which is not the scope of this study. Thus, the effect of semi-volatile organics was excluded from our consideration in this paper. The sulfuric acid concentration used in the calculations for was 1 ppt (2.58 × 107 molecules cm−3). It is shown that the aging time scale increases with BC particle size. The aging time scale also increases with increasing polydispersity (geometric standard deviation) and with decreasing accommodation coefficient. The effects of the BC particle size distribution, represented by dg and σg , on the aging time scale are shown to be considerable when dg > 0.1 μm. When dg is smaller than 0.01 μm, however, the aging time scale tends to converge to a constant value. This result can easily be predicted from Equation (17). When dg is much smaller than λV, Kn g is much larger than exp(2.5 ln2σ g ) and τ in Equation (17) reduces to a constant: τ=8λV/3αC. This phenomenon is explained by the characteristics of condensation process. When a BC particle is very small (in the free-molecule regime), the condensing flux is proportional to the surface area of the BC particle, which means that under a fixed-thickness coating criterion, the rate of aging does not depend on particle size. When BC particle is very large (in the continuum regime), however, the condensing flux is proportional to the BC particle size. Thus, as particle size increases, the condensing flux cannot catch up with particle surface area, leading to a decrease in the aging rate and hence an increase in the aging time scale. In contrast, the effect of accommodation coefficient is more pronounced when dg < 0.1 μm because the accommodation coefficient is important for free-molecule regime condensation.
FIG. 1 Aging time scale due to condensation of hygroscopic vapors as a function of geometric mean BC particle diameter for different polydispersity levels and accommodation coefficients.
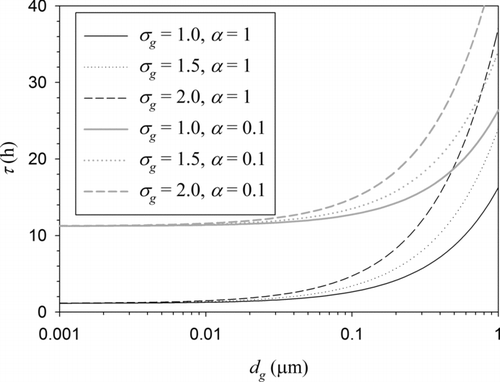
It is interesting to look into the polydispersity effect on the aging time scale in more detail. Let us define the polydispersity factor Fp as the ratio between the aging time scale of polydisperse BC particles and that of monodisperse particles with the same geometric mean particle size. Then, from Equation (17), one can easily derive the following equation for Fp :
For the free-molecule regime (Kn g >>1) and the continuum regime (Kn g =0), Equation (18) reduces to Fp =1 and Fp =exp(2.5ln2σ g ), respectively.
compares the polydispersity factors for different geometric mean BC particle sizes. The limiting case of the continuum regime is also compared. The accommodation coefficient was set at 1 for this figure. For dg = 0.001 μm, which is well within the free-molecule regime, Fp remains very close to 1, whereas for dg = 1 μm, the curve is very close to the limiting case of the continuum regime. It is clearly shown in this figure that the polydispersity effect is not negligible except for the particles well within the free-molecule regime (a few nanometer in diameter under atmospheric pressure) when the particle size is represented by an average value.
FIG. 2 Polydispersity factor as a function of geometric standard deviation for different geometric mean BC particle diameters. The limiting case of the continuum regime is also compared. For the computations, α = 1 was used.
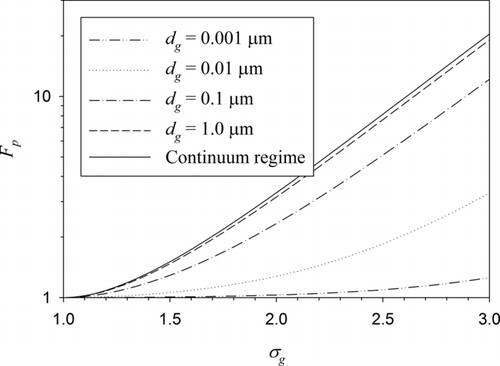
shows the aging time scale as a function of condensing vapor concentration for three different geometric mean BC particle diameters (0.01, 0.1, and 1 μm). It was assumed for the computations that σg = 1.5 and α = 1. The aging time scale is shown to vary a lot depending on the condensing vapor concentration as well as on BC particle diameter.
FIG. 3 Aging time scale as a function of condensing vapor concentration for different geometric mean BC particle diameters. For the computations, σg = 1.5 and α = 1 were used.
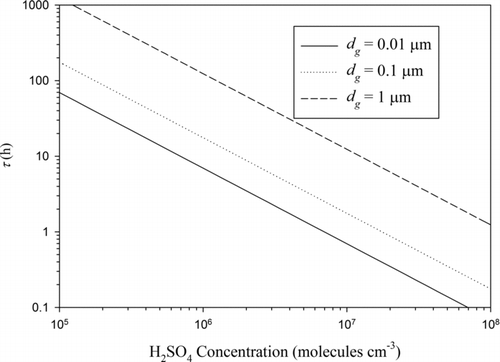
The results shown in through demonstrate that the aging time scale due to condensation is dependent to a large extent on the condensing species concentration as well as on BC particle size distribution. The condensing species concentration and the BC particle size distribution vary considerably depending on time and location, which implies that the aging time scale values suggested by Riemer et al. (Citation2004) based on a regional scale modeling, 2 h above 250 m height from the surface and 8 h below, cannot be easily generalized for being applied to global modeling, although their method was based on averaging over the whole mesoscale model domain, which contained many different conditions in terms of gas phase and particle size distributions, so the information of condensing species concentration and particle size distribution is implicitly contained. The size-dependent aging time scale suggested by Park (Citation2009) is not sufficient either because the polydispersity effect was not taken into account.
In the formulation of this paper, the change of the condensing vapor concentration as a result of condensation was not accounted for explicitly because it was assumed that the incorporation of the aging process into a global model using the characteristic time scale suggested in this study would be done on top of the model's own gas-to-particle conversion process. In the case where the global model's own gas-to-particle conversion process is different from that used in this study, the time evolution of condensing species concentration needed for calculating the aging time scale will not be perfectly provided by the global model. Nevertheless, we believe that it is still superior to using characteristic time scale that does not depend at all on particle size distribution and condensable species concentration.
The formulation of this study is based on a unimodal log-normal size distribution. In reality, however, atmospheric aerosol is comprised of three modes, i.e., nuclei, accumulation, and coarse modes, although externally mixed BC is expected to reside mostly in nuclei and accumulation modes, while coarse mode BC is considered to have been aged. In the case of multimodal size distribution, one can apply Equation (17) separately to calculate the characteristic aging time for each mode. The average aging time for total particle size distribution can then be obtained by mass-weighted averaging if it is needed. For example, the average aging time of all BC particles in nuclei and accumulation modes is calculated by
Thus far, all the parameterization has been based on the mass concentration of BC particles following the approach of Riemer et al. (Citation2004). This approach is particularly relevant when the optical properties of BC particles are of concern because the parameterizations for the radiative forcing of BC are usually based on BC particle mass burden (Chylek and Wong Citation1995; Bond and Bergstrom Citation2006; Park et al. Citation2011). However, if one is interested in the aging of BC particles not for their optical properties but for their effect on CCN, an approach based on BC particle number concentration may be more adequate because CCN is a number concentration. The aging time scale based on BC particle number concentration is defined as
By repeating the same procedure shown in Section 2.2 but with Equation (20) instead of Equation (5), one can derive the following equation for the aging time scale based on BC particle number concentration:
The corresponding polydispersity factor is given as
FIG. 4 Aging time scale due to condensation of hygroscopic vapors as a function of geometric mean BC particle diameter for different polydispersity levels and accommodation coefficients but for the number-based parameterization.
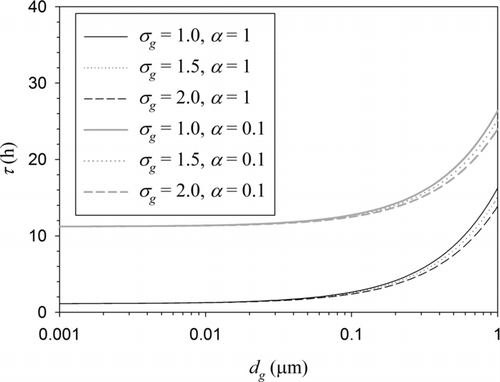
FIG. 5 Polydispersity factor as a function of geometric standard deviation for different geometric mean BC particle diameters but for the number-based parameterization. The limiting case of the continuum regime is also compared. For the computations, α = 1 was used.
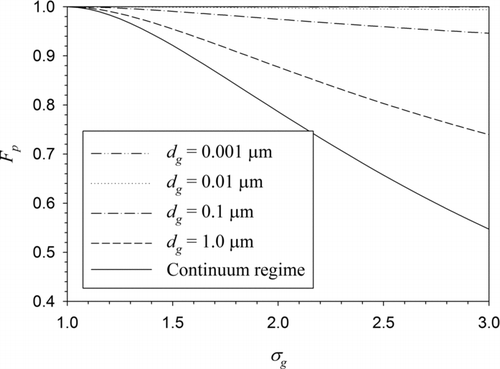
Note that the coefficient 2.5 appearing in the exponential term of Equations (17) and (18) was replaced by −0.5 in Equations (21) and (22), respectively.
shows the aging time scale based on BC particle number concentration. All the conditions used for preparing this figure are identical to those used for except for the definition of BC aging. Contrary to , the aging time scale decreases with increasing polydispersity (geometric standard deviation) and the effect is much smaller. shows the polydispersity factors for the number-based parameterization. Again, the effect of polydispersity is opposite to that shown in and much less significant. The results shown in and can be easily understood by comparing the effects of aging of small and large particles on the overall aging rate. The largest particles, which have the largest aging time scale, contain a large fraction of the mass but a small fraction of the number. Thus, the predicted aging time scale based on BC mass increases greatly when the width of the size distribution increases.On the other hand, the predicted aging time scale based on BC number does not depend as mush on the width of the size distribution because the effect of slow aging of largest particles is compensated by fast aging of smallest particles. Initially, the aging will occur faster with a broader size distribution as is indicated by Equation (22) and because the smallest particles will be aged first. As the aging proceeds, however, the average size of fresh BC particles will increase due to aging of smallest particles and thus the aging time will become larger than that for a narrower particle size distribution.
4. CONCLUSIONS
In this study, the aging time scale of BC aerosol particles due to condensation of hygroscopic species was parameterized as a function of the condensing vapor concentration and the BC size distribution. When the parameterization was based on the BC mass concentration, the aging time scale was shown to increase with BC particle size as well as with polydispersity of the size distribution. The effects of the BC particle size distribution on the aging time scale were shown to be considerable when dg > 0.1 μm. When dg is smaller than 0.01 μm, however, the aging time scale tends to converge to a constant value. This phenomenon was attributed to the relationship between the condensing flux and BC particle size: the condensing flux is proportional to the particle surface area in the free-molecule regime, whereas it is proportional to the particle diameter in the continuum regime. The polydispersity effect was shown to be non-negligible in most situations in the atmosphere. When the parameterization was based on the BC number concentration, the aging time scale is only slightly dependent on polydispersity because the effect of slow aging of largest particles is compensated by fast aging of smallest particles. It was shown in this study that the aging time scale is dependent on the condensing species concentration as well as on BC particle size distribution. This result implies that the simple aging time scale parameterizations suggested in the previous studies, which did not take into account the effects of condensing species concentration and particle size distribution, cannot be easily generalized for being applied to global modeling.
Acknowledgments
This study was supported by the CEFV (Center for Environmentally Friendly Vehicle) of Eco-STAR project from MOE (Ministry of Environment, Republic of Korea).
REFERENCES
- Ackerman , A. S. , Toon , O. B. , Stevens , D. E. , Heymsfield , A. J. , Ramanathan , V. and Welton , E. J. 2000 . Reduction of Tropical Cloudiness by Soot . Science , 288 : 1042 – 1047 .
- Ackerman , T. P. and Toon , O. B. 1981 . Absorption of Visible Radiation in Atmosphere Containing Mixtures of Absorbing and Non-Absorbing Particles . Appl. Optics, , 20 : 3661 – 3668 .
- Albrecht , B. A. 1989 . Aerosols, Cloud Microphysics, and Fractional Cloudiness . Science , 245 : 1227 – 1230 .
- Bae , G.-N. , Lee , S.-B. and Park , S.-M. 2007 . Vehicle-Related Fine Particulate Air Pollution in Seoul, Korea . Asian J. Atmos. Environ., , 1 : 1 – 8 .
- Bond , T. C. and Bergstrom , R. W. 2006 . Light Absorption by Carbonaceous Particles: An Investigative Review . Aerosol Sci. Technol., , 40 : 27 – 67 .
- Bond , T. C. , Habib , G. and Bergstrom , R. W. 2006 . Limitations in the Enhancement of Visible Light Absorption Due to Mixing State . J. Geophys. Res., , 111 : D20211 doi: 20210.21029/22006JD007315
- Chung , S. H. and Seinfeld , J. H. 2002 . Global Distribution and Climate Forcing of Carbonaceous Aerosols . J. Geophys. Res., , 107 : D19407 doi: 19410.11029/12001JD001397
- Chylek , P. , Videen , G. , Ngo , D. , Pinnek , R. G. and Klett , J. D. 1995 . Effect of Black Carbon on the Optical Properties and Climate Forcing of Sulfate Aerosols . J. Geophys. Res., , 100 ( 16 ) 325–316, 332
- Chylek , P. and Wong , J. 1995 . Effect of Absorbing Aerosols on Global Radiation Budget . Geophys. Res. Lett., , 22 : 929 – 931 .
- Cooke , W. F. , Liousse , C. , Cachier , H. and Feichter , J. 1999 . Construction of a 1×1 Fossil Fuel Emission Data Set for Carbonaceous Aerosol and Implementation and Radiative Impact in the ECHAM4 Model . J. Geophys. Res., , 104 ( 22 ) 137–122, 162
- Croft , B. , Lohmann , U. and von Salzen , K. 2005 . Black Carbon Ageing in the Canadian Centre for Climate modelling and Analysis Atmospheric General Circulation Model . Atmos. Chem. Phys., , 5 : 1931 – 1949 .
- Ech , T. F. , Holben , B. N. , Slutsker , I. and Setzer , A. 1998 . Measurements of Irradiance Attenuation and Estimation of the Aerosol Single Scattering Albedo for Biomass Burning in Amazonia . J. Geophys. Res., , 103 : 31865 – 31878 .
- Fuchs , N. A. and Sutugin , A. G. 1971 . “ High-Dispersed Aerosols ” . In Topics in Current Aerosol Research , Edited by: Hidy , G. M. and Brock , J. R. 1 – 60 . New York : Pergamon Press .
- Hansen , J. , Sato , M. and Ruedy , R. 1997 . Radiative Forcing and Climate Response . J. Geophys. Res., , 102 : 6831 – 6864 .
- Haywood , J. and Boucher , O. 2000 . Estimates of the Direct and Indirect Radiative Forcing Due to Tropospheric Aerosols . Rev. Geophys., , 38 : 513 – 543 .
- IPCC . 2007 . “ Climate Change 2007: The Physical Science Basis ” . In Contribution of Working Group I to the Fourth Assessment Report of the Intergovernmental Panel on Climate Change , Edited by: Solomon , S. , Qin , D. , Manning , M. , Chen , Z. , Marquis , M. , Averyt , K. B. , Tignor , M. and Miller , H. L. 996 Cambridge, UK and New York : Cambridge University Press .
- Jacobson , M. Z. 2000 . A Physically-Based Treatment of Elemental Carbon Optics: Implications for Global Direct Forcing of Aerosols . Geophys. Res. Lett., , 27 : 217 – 220 .
- Jacobson , M. Z. 2001 . Strong Radiative Heating due to the Mixing State of Black Carbon in Atmospheric Aerosols . Nature , 409 : 695 – 697 .
- Johnson , K. S. , Zuberi , B. , Molina , L. T. , Molina , M. J. , Iedema , M. J. Cowin , J. P. 2005 . Processing of Soot in an Urban Environment: Case Study from the Mexico City Metropolitan Area . Atmos. Chem. Phys., , 5 : 3033 – 3043 .
- Kaufman , Y. J. and Fraser , R. S. 1997 . The Effect of Smoke Particles on Cloud and Climate Forcing . Science, , 277 : 1636 – 1639 .
- Kaufman , Y. J. and Nakajima , T. 1993 . Effect of Amazon Smoke on Cloud Microphysics and Albedo . J. Appl. Meteor., , 32 : 729 – 744 .
- Kaufman , Y. J. , Tanre , D. and Boucher , O. 2002 . A Satellite View of Aerosols in the Climate System . Nature, , 419 : 215 – 223 .
- Khalizov , A. F. , Zhang , R. , Zhang , D. , Xue , H. , Pagels , J. and McMurry , P. H. 2009 . Formation of Highly Hygroscopic Soot Aerosols Upon Internal Mixing with Sulfuric Acid Vapor . J. Geophys. Res., , 114 : D05208 doi: 05210.01029/02008JD010595
- Koch , D. and Del Genio , A. D. 2010 . Black Carbon Semi-Direct Effects on Cloud Cover: Review and Synthesis . Atmos. Chem. Phys., , 10 : 7685 – 7696 .
- Lesins , G. , Chylek , P. and Lohmann , U. 2002 . A Study of Internal and External Mixing Scenarios and Its Effect on Aerosol Optical Properties and Direct Radiative Forcing . J. Geophys. Res., , 107 : 4094 doi: 4010.1029/2001JD000973
- Lohmann , U. and Feichter , J. 2001 . Can the Direct and Semidirect Aerosol Effect Compete with the Indirect Effect on a Global Scale? . Geophys. Res. Lett., , 28 : 159 – 161 .
- Lohmann , U. and Feichter , J. 2005 . Global Indirect Aerosol Effects: A Review . Atmos. Chem. Phys., , 5 : 715 – 737 .
- Lohmann , U. , Feichter , J. , Chuang , C. C. and Penner , J. E. 1999 . Prediction of the Number of Cloud Droplets in the ECHAM GCM . J. Geophys. Res., , 104 : 9169 – 9198 .
- Mann , G. W. , Carslaw , K. S. , Spracklen , D. V. , Ridley , D. A. , Manktelow , P. T. Chipperfield , M. P. 2010 . Description and Evaluation of GLOMAP-mode: A Modal Global Aerosol Microphysics Model for the UKCA Composition-Climate Model . Geosci. Model Dev., , 3 : 519 – 551 .
- Martins , J. V. , Artaxo , P. , Liousse , C. , Reid , J. S. , Hobbs , P. V. and Kaufman , Y. J. 1998 . Effects of Black Carbon Content, Particle Size, and Mixing on Light Absorption by Aerosol Particles from Biomass Burning in Brazil . J. Geophys. Res., , 103 : 32041 – 32050 .
- McFarquhar , G. M. and Wang , H. 2006 . Effects of Aerosols on Trade Wind Cumuli over the Indian Ocean: Model Simulations . Q. J. Roy. Meteor. Soc., , 132 : 821 – 843 .
- Menon , S. , Hansen , J. , Nazarenko , L. and Luo , Y. 2002 . Climate Effects of Black Carbon Aerosols in China and India . Science , 297 : 2250 – 2253 .
- Monchick , L. and Blackmore , R. 1988 . A Variation Calculation of the Rate of Evaporation of Small Droplets . J. Aerosol Sci., , 19 : 273 – 286 .
- Moteki , N. , Kondo , Y. , Miyazaki , Y. , Takegawa , N. , Komazaki , Y. Kurata , G. 2007 . Evolution of Mixing State of Black Carbon Particles: Aircraft Measurements Over the Western Pacific in March 2004 . Geophys. Res. Lett., , 34 : L11803 doi: 11810.11029/12006GL028943
- Pagels , J. , Khalizov , A. F. , McMurry , P. H. and Zhang , R. Y. 2009 . Processing of Soot by Controlled Sulphuric Acid and Water Condensation-Mass and Mobility Relationship . Aerosol Sci. Technol., , 43 : 629 – 640 .
- Park , S. H. 2009 . Particle-Size-Dependent Aging Time Scale of Atmospheric Black Carbon (in Korean) . Part. Aerosol Res., , 5 : 45 – 52 .
- Park , S. H. , Gong , S. L. , Bouchet , V. S. , Gong , W. , Makar , P. A. Moran , M. D. 2011 . Effects of Black Carbon Aging on Air Quality Predictions and Direct Radiative Forcing Estimation . Tellus B, , 63 : 1026 – 1039 .
- Park , S. H. and Lee , K. W. 2000 . Condensational Growth of Polydisperse Aerosol for the Entire Particle Size Range . Aerosol Sci. Technol., , 33 : 222 – 227 .
- Pratsinis , S. E. 1988 . Simultaneous Nucleation, Condensation, and Coagulation in Aerosol Reactors . J. Colloid Interf. Sci., , 124 : 416 – 427 .
- Pringle , K. J. , Tost , H. , Message , S. , Steil , B. , Giannadaki , D. Nenes , A. 2010 . Description and Evaluation of GMXe: A New Aerosol Submodel for Global Simulations (v1) . Geosci. Model Dev., , 3 : 391 – 412 .
- Ramana , M. V. , Ramanathan , V. , Feng , Y. , Yoon , S.-C. , Kim , S.-W. Carmichael , G. R. 2010 . Warming Influenced by the Ratio of Black Carbon to Sulphate and the Black-Carbon Source . Nature Geosci. , doi: 10.1038/NGEO1918
- Ramanathan , V. and Carmichael , G. 2008 . Global and Regional Climate Changes due to Black Carbon . Nature Geosci., , 1 : 221 – 227 .
- Ramanathan , V. , Crutzen , P. , Kiehl , J. and Rosenfeld , D. 2001 . Aerosols, Climate, and the Hydrological Cycle . Science , 294 : 2119 – 2124 .
- Riemer , N. , Vogel , H. and Vogel , B. 2004 . Soot Aging Time Scales in Polluted Regions During Day and Night . Atmos. Chem. Phys., , 4 : 1885 – 1893 .
- Riemer , N. , Vogel , H. , Vogel , B. and Fiedler , F. 2003 . Modeling Aerosols on the Mesoscale-γ: Treatment of Soot Aerosol and its Radiative Effects . J. Geophys. Res., , 108 : 4601 doi: 4610.1029/2003JD003448
- Riemer , N. , West , M. , Zaveri , R. and Easter , R. 2010 . Estimating Black Carbon Aging Time-Scales with a Particle-Resolved Aerosol Model . J. Aerosol Sci., , 41 : 143 – 158 .
- Riemer , N. , West , M. , Zaveri , R. A. and Easter , R. C. 2009 . Simulating the Evolution of Soot Mixing State with a Particle-Resolved Aerosol Model . J. Geophys. Res., , 114 : D09202 doi: 09210.01029/02008JD011073
- Rosenfeld , D. 2000 . Suppression of Rain and Snow by Urban and Industrial Air Pollution . Science, , 287 : 1793 – 1796 .
- Saathoff , H. , Naumann , K. H. , Schnaiter , M. , Schock , W. , Mohler , O. Schurath , U. 2003 . Coating of Soot and (NH4)2SO4 Particles by Ozonolysis Products of α-Pinene . J. Aerosol Sci., , 34 : 1297 – 1321 .
- Satheesh , S. K. and Ramanathan , V. 2000 . Large Differences in Tropical Aerosol Forcing at the Top of the Atmosphere and Earth's Surface . Nature, , 405 : 60 – 63 .
- Shiraiwa , M. , Kondo , Y. , Moteki , N. , Takegawa , N. , Miyazaki , Y. and Blake , D. R. 2007 . Evolution of Mixing State of Black Carbon in Polluted Air from Tokyo . Geophys. Res. Lett., , 34 : L16803 doi: 16810.11029/12007GL029819
- Slowik , J. G. , Cross , E. S. , Han , J.-H. , Kolucki , J. , Davidovits , P. Williams , L. R. 2007 . Measurements of Morphology Changes of Fractal Soot Particles Using Coating and Denuding Experiments: Implications for Optical Absorption and Atmospheric Lifetime . Aerosol Sci. Technol., , 41 : 734 – 750 .
- Textor , C. , Schulz , M. , Guibert , S. , Kinne , S. , Balkanski , Y. Bauer , S. 2006 . Analysis and Quantification of the Diversities of Aerosol Life Cycles Within AeroCom . Atmos. Chem. Phys., , 6 : 1777 – 1813 .
- Vignati , E. , Wilson , J. and Stier , P. 2004 . M7: An Efficient Size-Resolved Aerosol Microphysics Module for Large-Scale Aerosol Transport Models . J. Geophys. Res., , 109 : D22202 doi: 22210.21029/22003JD004485
- Wehner , B. , Berghof , M. , Cheng , Y. F. , Achtert , P. , Birmili , W. Nowak , A. 2009 . Mixing State of Nonvolatile Aerosol Particle Fractions and Comparison with Light Absorption in the Polluted Beijing Region . J. Geophys. Res. , 114 : D00G17 doi: 10.1029/2008JD010923
- Weingartner , E. , Burtscher , H. and Baltensperger , U. 1997 . Hygroscopic Properties of Carbon and Diesel Soot Particles . Atmos. Environ., , 31 : 2311 – 2327 .
- Xue , H. , Khalizov , A. F. , Wang , L. , Zheng , J. and Zhang , R. 2009 . Effects of Coating of Dicarboxylic Acids on the Mass-Mobility Relationship of Soot Particles . Environ. Sci. Technol., , 43 : 2787 – 2792 .
- Zaveri , R. A. , Barnard , J. C. , Easter , R. C. , Riemer , N. and West , M. 2010 . Particle-Resolved Simulation of Aerosol Size, Composition, Mixing State, and the Associated Optical and Cloud Condensation Nuclei Activation Properties in an Evolving Urban Plume . J. Geophys. Res., , 115 : D17210 doi: 17210.11029/12009JD013616
- Zhang , R. , Khalizov , A. F. , Pagels , J. , Zhang , D. , Xue , H. and McMurry , P. H. 2008 . Variability in Morphology, Hygroscopicity, and Optical Properties of Soot Aerosols During Atmospheric Processing . Proc. Natl. Acad. Sci. USA, , 105 : 10291 – 10296 .
- Zuberi , B. , Johnson , K. S. , Aleks , G. K. , Molina , L. T. and Molina , M. J. 2005 . Hydrophilic Properties of Aged Soot . Geophys. Res. Lett., , 32 : L01807 doi: 01810.01029/02004GL021496