Abstract
The degree of crystallinity of nanometer size lipid matrices governs drug loading and release rates. Recently, droplet-phase aerosol synthesis was used to prepare lipid nanoparticles of stearic acid and achieve control over their crystallinity using precursor solvents with differing vapor pressures. The present work aims at examining relationships between solvent evaporation rate and extent of evaporative cooling, during drop evaporation, on the crystallinity of the resulting lipid nanoparticles. A stationary drop model was developed to study evaporation of submicron-sized solution drops, of stearic acid in organic solvents, by including mechanisms of solvent vapor pressure depression by the solute, heat and mass transfer between the drop ensemble and suspending gas, Kelvin (curvature) effect, noncontinuum vapor transfer effects, and changes in activity coefficients of solute and solvent with changing concentrations. It was found that increasing estimated evaporation rates correlated with decreasing measured crystallinity. Higher evaporation rates also led to greater evaporative cooling and lower drop temperatures. The rate of change of supersaturation in solution drops under fast evaporation was shown to be an order of magnitude higher than that for slow evaporation. The modeled evaporation rate and drop temperature depend primarily on vapor pressure and enthalpy of vaporization of the precursor solvent. This suggests that selection of precursor solvents, with desired physical properties, can be used to control crystallinity, and related drug release behavior of lipid nanoparticles made through aerosol synthesis routes.
Copyright 2012 American Association for Aerosol Research
1. INTRODUCTION
Droplet-phase aerosol synthesis of biocompatible nanoparticles for drug delivery, with diameters of 50–200 nm, has recently been explored using pure drugs, lipids, and polymers (Eerikainen and Kauppinen Citation2003; Raula et al. Citation2004; Pawar and Venkataraman Citation2011). Lipid nanoparticles for drug delivery are typically prepared from lipids like stearic acid and glycerides of lauric, stearic, and behenic acid (zur Muhlen et al. Citation1998; Mehnert and Mader Citation2001; Hou et al. Citation2003). The rate of drug release from lipid nanoparticles has been related to particle properties like size, morphology, and crystallinity (Brannon-Peppas Citation1995; Westesen et al. Citation1997; zur Muhlen et al. Citation1998; das Neves et al. Citation2010). Lower release rates of encapsulated drugs could result from lower degradation rates of lipid particles by enzymes like lipases (Olbrich et al. Citation2002). It has also been suggested that release rates of encapsulated drugs could be lower from lipid matrices of higher crystallinity, through reduction in solid–solid diffusion (Muller et al. Citation2000).
The effect of solvent evaporation rates on the crystallization kinetics and, consequently, on the degree of crystallinity of resulting nanoparticles is of interest in droplet-phase aerosol synthesis. Recently, stearic acid lipid nanoparticles of differing crystallinity were synthesized through the droplet-phase aerosol route, at temperatures below 300 K (Pawar and Venkataraman Citation2011). Precursor solvents of differing vapor pressures were used to control evaporation rates of solution drops leading to formation of lipid nanoparticles. Nanoparticles synthesized using lower vapor pressure solvents, resulting in slower evaporation, exhibited higher crystallinity than those synthesized using higher vapor pressure solvents, with faster evaporation rates. The observed influence of vapor pressure or evaporation rates on the extent of crystallinity could result from several mechanisms, including the effect of evaporation-mediated drop temperature depression on the kinetics of crystallization, including nucleation and/or ordering of nuclei.
The quantitative connection between the evaporation rate of nanometer-sized solution drops and crystallinity of nanoparticles produced has not been studied in literature. In bulk solution, slow evaporation has been linked to large grains, long-range order, and fewer defects in thin film formation from pentacene and gold nanocrystals (Gundlach et al. Citation1997; Shah et al. Citation2003). While some studies had a qualitative measure of evaporation rate, others included measured evaporation rate of the solvent (Shah et al. Citation2003). In millimeter-sized solution drops, slower evaporation led to the formation of fewer, larger crystals in proteins like egg white lysozyme (Wilson et al. Citation1991; Wilson and Suddath Citation1992). In micrometer-sized aqueous inorganic zirconium hydrochloride and magnesium sulfate solution drops, slower evaporation rates were linked to higher crystallinity (Eslamian and Ashgriz Citation2006). The relative difference in crystallinity of the particles, measured using X-ray diffraction (XRD), was related to the evaporation rate estimated from a model. On the computational side, studies have evaluated conditions leading to different size and morphology of the particles, through prediction of internal solute concentration gradients (Eslamian et al. Citation2009).
In the present work, a computational model for stationary nanodrop evaporation has been developed to explore the relationship among solvent physical properties, resulting drop evaporation rate, and measured crystallinity of lipid nanoparticles produced during droplet-phase aerosol synthesis as reported in our previous work (Pawar and Venkataraman Citation2011). Evaporation rates are estimated from modeling because the very small time scales involved make measurements difficult. The effect of evaporative cooling and nanodrop curvature on solution equilibria, noncontinuum effect on vapor transfer, and mass exchange with the suspending gas applicable to nanodrops, changing activity of solvents and vapor pressure depression, have been considered.
2. MODEL DEVELOPMENT
2.1. Simultaneous Mass and Heat Transfer
During evaporation, the drop shrinks in size and the expression for drop diameter can be written considering the rate at which the solvent vapor diffuses away from the drop as follows:
Equation (1) also assumes that the drop is “stationary” in the suspending medium i.e., the Sherwood number for mass transport is equal to 2. The vapor pressure, at the surface of a solution drop (Pd ), is affected by the activity of solvent and the drop curvature (Kelvin effect). The concentration of the nonvolatile species (solute) in the solution drop increases as the solvent evaporates from the drop surface. A decrease in the solvent mole fraction in the evaporating drop decreases the solvent activity. To account for the decrease in solvent activity, the activity coefficient of the solvent was calculated by the UNIFAC (Universal Functional Activity Coefficient) group contribution method. Equation (3) was used to compute the vapor pressure at the surface of a drop (Pd ) relative to solvent vapor pressure (Ps ), taking into account the effect of activity of the solvent (as ) and drop curvature (Pruppacher and Klett Citation1997),
As evaporation continues, the solvent vapor accumulates in the suspending gas resulting in an increase of solvent partial pressure (P ∞), which reduces the driving force for drop evaporation (Pd −P ∞). Using the ideal gas law and the solvent mass transfer rate from solution drops to suspending gas yields, for the change in P ∞,
During evaporation, there is continuous loss of sensible heat from the drop due to energy being useful for latent heat of vaporization, leading to evaporative cooling. Some of this heat is recovered by heat transfer from the suspending gas to the drops. The heat balance on a single stationary drop is given by
TABLE 1 Physical properties (vapor pressure, surface tension, diffusivity, density, and specific heat capacity) of precursor solvents cyclohexane, chloroform, dichloromethane, and diethyl ether at 298 K.
The temperature of the surrounding gas was obtained by considering heat transfer between the gas and drops, as follows:
2.2. Solute Solubility as a Function of Temperature
The solute solubility is a function of temperature (Gmehling et al. Citation1978). The solubility, in mole fraction (xs ), can be estimated at different drop temperatures if the heat of fusion (ΔHf ) and melting point temperature (Tm ) of the solute are known. This is described by
Equations (1)–(7), along with UNIFAC activity correlations, were solved using MATLAB (Version 7.0, The MathWorks, Inc., Natick, MA, USA). The physical properties used as input to the numerical calculations are listed in . The drop diameter (dp ) and temperature (Td ), suspending gas temperature (T ∞), solvent partial pressure in the suspending gas (P ∞), and solute solubility (xs ) are the output variables. The instantaneous evaporation rate was also computed, using
Further, the mean evaporation rate was calculated from the mass balance of the solvent or from the time average of the instantaneous rate over the evaporation time scale of the drop and is written as follows:
The supersaturation ratio (S) is defined as the ratio between solute concentration (c) and solubility (cs ) (S=c/cs ). The rate of change of supersaturation at equilibrium saturation (ES) is the time rate of change of supersaturation ratio when the solute in the drop is just saturated, i.e., S = 1 and is given by
The calculation was terminated when the computed drop diameter reached the experimentally obtained dry particle mean mobility diameter (Pawar and Venkataraman, Citation2011).
3. RESULTS AND DISCUSSION
3.1. Evaporation History of the Solution Drop
The evaporation history of a solution drop is shown for the system of stearic acid solution in CHL (–b). A monodisperse drop aerosol, of number concentration 2 × 1012/m3, and an initial solution concentration of 10 kgm−3 was considered. The initial drop and suspending gas temperatures were set at 25°C. Solvent vapor content in the suspending gas, at the start of evaporation, was set to zero.
FIG. 1 (a) Solvent vapor pressure over drop and instantaneous evaporation rate and (b) drop temperature and temperature of suspending gas, with time for a stearic acid solution in chloroform drop of initial diameter 350 nm with stearic acid concentration = 10 mgmL−1, number concentration = 2 × 1012/m3, initial drop temperature = 25°C, and carrier gas temperature = 25°C.
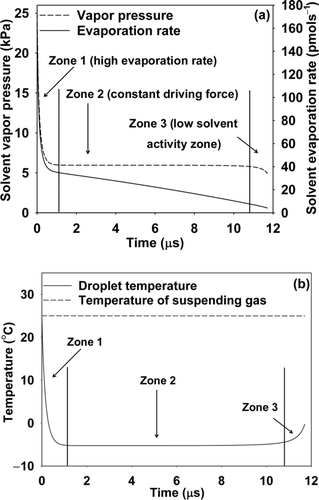
Three distinct zones of evaporation can be seen (–b). The first zone is a short transient zone with high evaporation rate. During this time, the high initial drop temperature provides a high driving force for evaporation. The resulting evaporative cooling leads to a sharp drop in the drop temperature. The second zone is relatively longer, with both drop temperature and vapor pressure driving force nearly constant. As evaporation proceeds, a decrease in the drop size increases the intensity of the curvature (Kelvin) effect. However, at the same time, the solvent activity decreases because of increase in the solute concentration. Thus, by the end of second zone, the competing Kelvin and the solvent activity effects lead to an overall decrease in vapor pressure over the drop. Solvent activity is low in the third zone and heat gained from the suspending gas is larger than the latent heat losses consumption to an increase in temperature of the drop.
The present observations are consistent with the reported literature on evaporation of micron-sized solution drops of inorganic salts. Eslamian and Ashgriz (2006) also showed three zones with initial rapid cool-down, followed by a relatively constant temperature zone, and increase in temperature during final stages of evaporation, for the case of evaporation of micron-sized zirconium hydrochloride solution drops.
FIG. 2 Measured percent crystallinity of stearic acid lipid nanoparticles synthesized by evaporating 10 mgmL−1 stearic acid solution drops of mean diameter 350 nm in cyclohexane, chloroform, dichloromethane, and diethyl ether (Pawar and Venkataraman Citation2011) compared with the average solvent evaporation rates for the respective solvents.
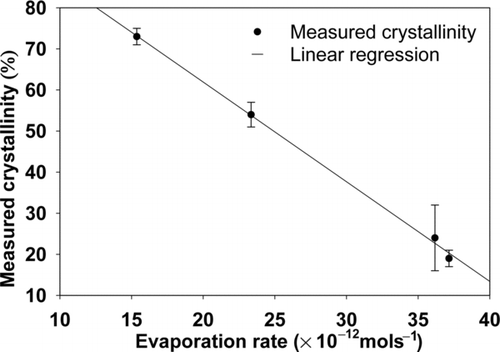
3.2. Relation Between Predicted Evaporation Rate and Measured Crystallinity
In order to investigate the relation between drop evaporation rate and crystallinity, the computed evaporation rates were correlated with the measured crystallinity of stearic acid nanoparticles (Pawar and Venkataraman Citation2011). The experimental conditions used for synthesis of stearic acid lipid nanoparticles of varying crystallinity were used as inputs to the present model (). Atomized stearic acid solution (10 kgm−3 concentration) drops of mean diameter 350 nm, at a drop number concentration of 2 × 1012/m3, in suspending gas maintained at 25°C were considered. The initial drop temperature was assumed to be at 25°C. The selected precursor solvents included CYC, CHL, DCM, and DEE with vapor pressures differing by a factor of five. The predicted drop temperatures, for precursor solvents with higher vapor pressures, DCM and DEE, at the second evaporation zone, were −17.6°C and −17.9°C, respectively. In contrast, the predicted drop temperatures, for precursor solvents with lower vapor pressures, CYC and CHL, were 1.95°C and −5.25°C, respectively. This leads to corresponding reductions in saturation vapor pressure at the drop surface. Despite larger reductions in the relative saturation vapor pressures, drops containing higher vapor pressure solvents were subjected to a larger driving force, leading to a smaller evaporation time. Thus, the average evaporation rate was higher for drops of higher vapor pressure solvents ().
TABLE 2 Measured particle crystallinity of lipid nanoparticles synthesized using 10 mgmL−1 stearic acid solutions in cyclohexane, chloroform, dichloromethane, and diethyl ether and estimated average evaporation rate, drop temperature in constant driving force zone, and rate of supersaturation at saturation.
The mean evaporation rates (Equation (9)) show a negative linear correlation with measured crystallinity of stearic acid nanoparticles synthesized (). Evaporation rate influences the drop temperature, the resulting solubility, and rate of change of super-saturation, thereby governing crystallization kinetics (i.e., the rate of self-assembly by nucleation and growth into periodical, regular structures). Thus, it can be argued that crystal structure formation is governed by the evaporation rate. For a CHL drop containing stearic acid, the change in solubility and concentration of solute during evaporation () indicates that saturation concentration was reached near 0.25 μs after the start of evaporation. At this time, the drop temperature (, CHL line) had dropped to 1.5°C, close to its constant value. Consequently, reduction in the drop temperature resulted in reduced vapor pressures (). Similar behavior was observed with other solvents as well. Stearic acid nanoparticles synthesized at higher mean evaporation rates exhibited lower crystallinity, which also correlated with the predicted lower drop temperatures (). The predicted behavior is consistent with crystallization studies of other systems, polymer blends, nanometer fibers, and inorganic particles, in which crystallization was observed to depend on solvent vapor pressure and evaporation rate (Leong Citation1981; Runt and Rim Citation1982; Palermo et al. Citation2006).
FIG. 3 Solubility and solute concentration (mg/mL) inside an evaporating drop of initial concentration of 10 mgmL−1 stearic acid in chloroform of mean diameter 350 nm till 6 μs of drop evaporation, number concentration = 2 × 1012/m3, initial drop temperature = 25°C, and carrier gas temperature = 25°C.
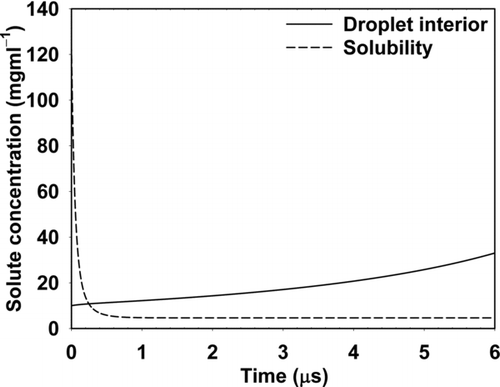
FIG. 4 (a) Drop temperature and (b) solvent vapor pressure of solvent with time for drops of diameter 350 nm of stearic acid solution in cyclohexane, chloroform, dichloromethane, and diethyl ether with stearic acid concentration = 10 mgmL−1, number concentration = 2 × 1012/m3, initial drop temperature = 25°C, and carrier gas temperature = 25°C.
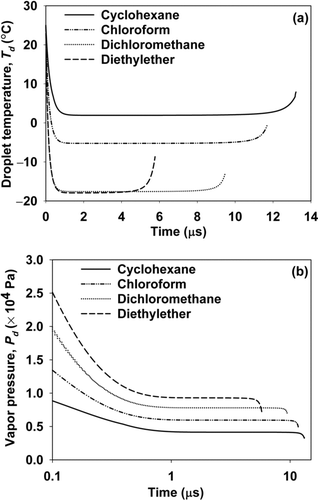
3.3. Effect of Precursor Solvent Physical Properties on Evaporation Rate
To evaluate the extent of control that could be achieved on evaporation rate and drop temperature, through precursor solvent selection, the proposed model was used to predict the influence of vapor pressure and enthalpy of vaporization, on the evaporation rate. Vapor pressures, in the range 875–66,528 Pa and enthalpy of vaporization from 27.39–52.66 kJmol−1(at 25°C), were selected, being representative of typical organic solvents permitted for use in the drug industry (ICH [International Conference on Harmonisation] Citation1997). These include 1-butanol, ethanol, CYC, CHL, acetone, DCM, and DEE. The average evaporation rate and drop temperature in constant temperature zone are shown as a function of these two physical properties (–b). Two-dimensional plots of average evaporation rate (Figure S1a) and drop temperature (Figure S1b) as a function of solvent vapor pressure, at three specific enthalpies, are included in the supplemental information, which can be used for quantitative extraction of data.
FIG. 5 (a) Average evaporation rate and (b) drop temperature in constant driving force zone with different initial solvent vapor pressures and enthalpy of vaporization for an evaporating drop of 350 nm with initial stearic acid concentration 10 mgmL−1. Drop number concentration = 2 × 1012/m3, initial drop temperature = 25°C, and carrier gas temperature = 25°C.
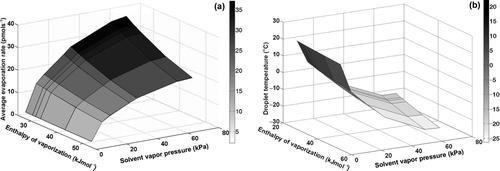
As can be seen, the mean evaporation rate is more strongly influenced by solvent vapor pressure than the enthalpy of vaporization. However, enthalpy of vaporization has a significant influence on evaporation rate at higher vapor pressures. The resulting drop temperature in the constant driving force region is also more strongly influenced by vapor pressure than enthalpy of vaporization; however, the influence of the latter does not change much over the chosen vapor pressure range. Thus, control of crystallinity of lipid nanoparticles can be achieved through selection of precursors based on both these physical properties.
The magnitude of the evaporation rate also governs the rate of change of supersaturation, another parameter that has been linked to crystallization kinetics (Wilson et al. Citation1991; Wilson and Suddath Citation1992; Foks and Luszczek Citation1993; Martins et al. Citation2008). An overall effect of evaporation rates on crystallization process is considered; nucleation and growth have not been considered separately. A higher rate of supersaturation will cause larger peaks of supersaturation. A higher evaporation rate was observed to lead to larger supersaturation in polymer systems like polyethylene adipate (Foks and Luszczek Citation1993). Higher supersaturation led to the formation of amorphous solids or solids with lower degree of ordering (Wilson et al. Citation1991; Martins et al. Citation2008). From the present model, the rate of change of supersaturation was computed using Equation (10). The calculated rate of change of supersaturation at ES was an order of magnitude lower for drops containing precursor solvents having lower vapor pressure (). Investigations on crystallization kinetics and transport processes of solute within an evaporating drop need to be carried out for a better understanding of mechanisms influencing the crystallization process.
4. CONCLUSIONS
A model to estimate solvent evaporation rates, and consequent evaporative cooling, for nanodrops is developed and applied to lipid–organic solvent systems, deployed for the production of lipid nanoparticles. The evaporation history of the nanodrop shows three zones: a high evaporation rate zone, a constant driving force zone, and a low solvent activity zone. A higher evaporation rate leads to lower drop temperatures and correlates with lower measured crystallinity. Further, the rate of change of supersaturation, at ES, is an order of magnitude greater for solvents with higher vapor pressure. This is likely to lead to a relatively faster nucleation than the rate of crystal lattice formation. Significant control over both evaporation rate and drop temperature can be achieved through selection of solvents based on both vapor pressure and enthalpy of vaporization.
GLOSSARY
as | = |
activity of solvent in the solution drop |
c | = |
solute concentration within a drop (kgm−3) |
cs | = |
solute solubility within a drop (kgm−3) |
Cpg | = |
heat conductivity of suspending gas (Jkg−1K−1) |
Cpl | = |
heat conductivity of solution drop (Jkg−1K−1) |
CSS | = |
critical supersaturation |
dp | = |
diameter of evaporating drop (m) |
dpo | = |
initial diameter of evaporating drop (m) |
dpe | = |
final diameter of evaporating drop (m) |
D | = |
diffusivity of solvent vapor in suspending gas (m2s−1) |
ES | = |
equilibrium saturation or solubility |
Fi | = |
instantaneous evaporation rate (mols−1) |
![]() | = |
mean evaporation rate (mols−1) |
H | = |
enthalpy of vaporization (Jkg−1) |
k | = |
thermal conductivity of suspending gas (Wm−1K−1) |
M | = |
molecular weight of solvent (kgmol−1) |
N | = |
number of solution drops |
Pd | = |
solvent pressure over the drop (Pa) |
Ps | = |
solvent saturation vapor pressure (Pa) |
P ∞ | = |
partial pressure of solvent in the suspending gas (Pa) |
R | = |
gas constant (Jmol−1K−1) |
Rs | = |
rate of supersaturation at ES |
S | = |
supersaturation ratio |
t | = |
time (s) |
te | = |
time for evaporation of drop (s) |
Td | = |
temperature of the drop (K) |
T ∞ | = |
temperature of the suspending gas (K) |
V | = |
volume of suspending gas (m3) |
xs | = |
solubility of stearic acid in solvent |
Greek Symbols
γ s | = |
solute activity coefficient in the drop |
λ | = |
mean free path of suspending gas (m) |
φ | = |
Fuch's correction factor |
ρ | = |
density of solution (kgm−3) |
ρg | = |
density of suspending gas (kgm−3) |
σ | = |
surface tension of drop-air interface (Nm−1) |
uast_a_648287_sup_23232375.zip
Download Zip (1.2 MB)Acknowledgments
[Supplementary materials are available for this article. Go to the publisher's online edition of Aerosol Science and Technology to view the free supplementary files.]
REFERENCES
- Brandreth , D. A. and Johnson , R. E. 1971 . Solubility of Stearic Acid in Some Halofluorocarbons, Chlorocarbons, Ethanol, and Their Azeotropes . J. Chem. Eng. Data, , 16 : 325 – 327 .
- Brannon-Peppas , L. 1995 . Recent Advances on the Use of Biodegradable Microparticles and Nanoparticles in Controlled Drug Delivery . Int. J. Pharm., , 116 : 1 – 9 .
- das Neves , J. , Amiji , M. M. , Bahia , M. F. and Sarmento , B. 2010 . Nanotechnology-Based Systems for the Treatment and Prevention of HIV/AIDS . Adv. Drug Deliver. Rev., , 62 : 458 – 477 .
- Eerikainen , H. and Kauppinen , E. I. 2003 . Preparation of Polymeric Nanoparticles Containing Corticosteroids by a Novel Aerosol Flow Reactor Method . Int. J. Pharm., , 263 : 69 – 83 .
- Eslamian , M. , Ahmed , M. and Ashgriz , N. 2009 . Modeling of Solution Droplet Evaporation and Particle Evolution in Droplet-to-Particle Spray Methods . Drying Technol., , 27 : 3 – 13 .
- Eslamian , M. and Ashgriz , N. 2006 . Effect of Precursor, Ambient Pressure, and Temperature on the Morphology, Crystallinity, and Decomposition of Powders Prepared by Spray Pyrolysis and Drying . Powder Technol., , 167 : 149 – 159 .
- Foks , J. and Luszczek , M. 1993 . Effect of Temperature and Supersaturation on the Crystallization of Polyethylene Adipate from Dioxane Solutions . J. Cryst. Growth, , 134 : 347 – 352 .
- Gmehling , J. G. , Anderson , T. F. and Prausnitz , J. M. 1978 . Solid-Liquid Equilibria Using UNIFAC . Ind. Eng. Chem. Fund., , 17 : 269 – 273 .
- Gundlach , D. J. , Lin , Y. Y. , Jackson , T. N. , Nelson , S. F. and Schlom , D. G. 1997 . Pentacene Organic Thin-Film Transistors: Molecular Ordering and Mobility . IEEE Electron Device Lett., , 18 : 87 – 89 .
- Hinds , W. C. 1999 . Aerosol Technology: Properties, Behavior, and Measurement of Airborne Particles , New York : John Wiley .
- Hoerr , C. W. and Ralston , A. W. 1944 . The Solubilities of the Normal Saturated Fatty Acids. II. . J. Org. Chem., , 9 : 329 – 337 .
- Hou , D. Z. , Xie , C. S. , Huang , K. J. and Zhu , C. H. 2003 . The Production and Characteristics of Solid Lipid Nanoparticles (SLNs) . Biomaterials, , 24 : 1781 – 1785 .
- ICH . 1997 . Impurities: Guideline for Residual Solvents in Q3C(R4) , Brussels : ICH, ed. ICH Secretariat .
- Kolb , D. K. and Brown , J. B. 1955 . Low Temperature Solubilities of Fatty Acids in Selected Organic Solvents . J. Am. Oil Chem. Soc., , 32 : 357 – 361 .
- Leong , K. H. 1981 . Morphology of Aerosol Particles Generated from the Evaporation of Solution Drops . J. Aerosol Sci., , 12 : 417 – 435 .
- Martins , P. M. , Rocha , F. and Damas , A. M. 2008 . Understanding Water Equilibration Fundamentals as a Step for Rational Protein Crystallization . PLoS ONE, , 3 : 1 – 10 .
- Mehnert , W. and Mader , K. 2001 . Solid Lipid Nanoparticles—Production, Characterization and Applications . Adv. Drug Delivery Rev., , 47 : 165 – 196 .
- Muller , R. H. , Mader , K. and Gohla , S. 2000 . Solid Lipid Nanoparticles (SLN) for Controlled Drug Delivery—A Review of the State of the Art . Eur. J. Pharm. Biopharm., , 50 : 161 – 177 .
- Olbrich , C. , Kayser , O. and Muller , R. H. 2002 . Enzymatic Degradation of Dynasan 114 SLN—Effect of Surfactants and Particle Size . J. Nanopart. Res., , 4 : 121 – 129 .
- Palermo , V. , Morelli , S. , Simpson , C. , Mu , K. and Samor , P. 2006 . Self-Organized Nanofibers from a Giant Nanographene: Effect of Solvent and Deposition Method . J. Mater. Chem., , 16 : 266 – 271 .
- Pawar , A. A. and Venkataraman , C. 2011 . Droplet-Phase Synthesis of Nanoparticle Aerosol Lipid Matrices with Controlled Properties . Aerosol Sci. Technol., , 45 : 811 – 820 .
- Pruppacher , H. R. and Klett , J. D. 1997 . Microphysics of Clouds and Precipitation , Dordrecht, the Netherlands : Kluwer Academic .
- Raula , J. , Eerikainen , H. and Kauppinen , E. I. 2004 . Influence of the Solvent Composition on the Aerosol Synthesis of Pharmaceutical Polymer Nanoparticles . Int. J. Pharm., , 284 : 13 – 21 .
- Runt , J. and Rim , P. B. 1982 . Effect of Preparation Conditions on the Development of Crystallinity in Compatible Polymer Blends: Poly(styrene-Co-Acrylonitrile)/Poly(ecaprolactone) . Macromolecules, , 15 : 1018 – 1023 .
- Shah , P. S. , Novick , B. J. , Hwang , H. S. , Lim , K. T. , Carbonell , R. G. Johnston , K. P. 2003 . Kinetics of Nonequilibrium Nanocrystal Monolayer Formation: Deposition from Liquid Carbon Dioxide . Nano Lett., , 3 : 1671 – 1675 .
- Westesen , K. , Bunjes , H. and Koch , M. H. J. 1997 . Physicochemical Characterization of Lipid Nanoparticles and Evaluation of their Drug Loading Capacity and Sustained Release Potential . J. Control. Release, , 48 : 223 – 236 .
- Wilson , L. J. , Bray , T. L. and Suddath , F. L. 1991 . Crystallization of Proteins by Dynamic Control of Evaporation . J. Cryst. Growth , 110 : 142 – 147 .
- Wilson , L. J. and Suddath , F. L. 1992 . Control of Solvent Evaporation in Hen Egg White Lysozyme Crystallization . J. Cryst. Growth , 116 : 414 – 420 .
- zur Muhlen , A. , Schwarz , C. and Mehnert , W. 1998 . Solid Lipid Nanoparticles (SLN) for Controlled Drug Delivery—Drug Release and Release Mechanism . Eur. J. Pharm. Biopharm., , 45 : 149 – 155 .