Abstract
Silica (SiO2) aerosol gels were formed via Brownian aggregation of silica nanoparticles in a closed reaction chamber. A sudden and quick detonation reaction of pyrophoric silane (SiH4) with either oxygen or nitrous oxide created silica nanoparticles with diameters ranging from ∼22 to 90 nm in the presence of an inert background gas with a volume fraction of ca. 10−4, conditions necessary for gelation. The background gas was necessary for quick thermal quenching of freshly formed silica molecules and molten nanoparticles and some control of the particle size could be achieved by variation of the gas. The silica aerosol gels were found to have very low densities in the range 4–15 mg/cm3 and high specific surface areas of 300–500 m2/g. Wide angle X-ray diffraction showed that the nanoparticles were amorphous silica. Neutron scattering showed that they were arranged in networks with a fractal dimension of 1.75 between 10 and 1000 nm length scales.
Copyright 2012 American Association for Aerosol Research
INTRODUCTION
In previous work, we presented an aerosol gelation method to produce porous materials with high specific surface area and extremely low density (Dhaubhadel et al. Citation2007). The method involved the gelation of nanoparticles in the aerosol phase to yield a material that we have named an “aerosol gel.” Unlike well-known aerogel materials which begin with a liquid phase sol-gel step, aerosol gels are made in the gas phase. Simply said a cloud of smoke in a volume gels or freezes to form a volume spanning, very light weight, porous body; truly “frozen smoke.” The initial aerosol is composed of nanometer-sized particles produced rapidly by exploding in a chamber a hydrocarbon precursor with an oxidizer, e.g., oxygen. The nanometer particles so produced aggregate and then gel on the order of tens of seconds to form the aerosol gel. The carbon materials we made previously have densities as low as 2.5 mg/cc. The current state-of-the-art for manufacture of aerogel materials is the sol-gel/supercritical-drying method (Brinker and Scherer Citation1990; Hüsing and Schubert Citation1998). The gas phase aerosol gelation method is a significantly different than this state-of-the-art and hence might offer advantages because (1) there is no need for a supercritical drying step as for aerogels and (2) the aerosol gel method should be applicable to a greater variety of substances. Disadvantages of our method lie in the current need for a detonation which could be hard to scale up, and it is a batch process.
Gelation is a consequence of random aggregation of noncoalescing particles to form ramified fractal aggregates. Fractal aggregates have a mass-size scaling exponent, the fractal dimension Df , that is less than the spatial dimension, d, and this inequality represents a fundamental condition for gelation (Sorensen and Chakrabarti Citation2011). Because of this, the ratio of aggregate mean nearest neighbor separation to aggregate size declines as the aggregation process proceeds with time until the separation equals the size. At this point, the aggregates jam together to form a gel.
A practical condition for gelation is that the time for the dispersed system to form a gel, the gel time, be short relative to other characteristic times such as settling time, wall deposition time, etc. Under the conditions of spherical, same-sized monomers, and monodisperse aggregates, simple kinetic arguments (Dhaubhadel et al. Citation2007) lead to an expression for the gel time as
Such conditions were met in our initial discovery of aerosol gelation in an acetylene flame (Sorensen et al. Citation1998a) and our first work to create an aerosol gel in which we reacted hydrocarbons, most notably acetylene, explosively with oxygen under fuel-rich conditions (Dhaubhadel et al. Citation2007). The rapid reaction led to nanometer-sized carbon particles that gelled via random Brownian motion on the order of 100 s. The resulting material had very low density and high specific surface area.
In this work, we extend the aerosol gelation method to another compound, silica (SiO2). The precursor gas was silane (SiH4), which reacts on contact with oxygen and nitrous oxide. A special apparatus was built to control this detonation reaction. Our result was the production of aerosol gels of silica.
EXPERIMENTAL METHODS
Silica (SiO2) aerosols were created by explosive oxidation of silane (SiH4) with an oxidizer, either oxygen (O2) or nitrous oxide (N2O). Since silane is a pyrophoric gas, a special arrangement was built for rapid mixing of the silane with the oxidizer in a closed reaction chamber. A schematic diagram of the set up is illustrated in . The reaction chamber was a thick-walled aluminum chamber with 12.5 cm internal diameter and a 31.5 cm height thus a volume of 3.9 liters. This reaction chamber was connected to two reservoirs, each through an electric solenoid valve and a one-way spring-loaded piston check valve. One of these reservoirs contained silane, while the other contained the oxidizing gas. Arrangements were also made so that a relatively inert “background gas” (He, N2, and CO2) could be introduced in either the reaction chamber or mixed with the silane or oxidizer in the reservoirs. The reservoirs were over pressured relative to the reaction chamber so that when the valves were opened, the gases in the reservoirs rushed into the chamber and reacted. The silane gas cylinder was equipped with a cross purge system to handle the pyrophoric gas. We used N2 as the purging gas. The whole arrangement was placed under an exhaust hood for the safety purpose. Safety precautions were also exercised by remotely releasing the electric solenoid valve(s) using a wired electric switch. After hearing the detonation sound, we waited several minutes to allow aerosol aggregation and subsequent gelation before opening the reaction chamber.
We experimented making the silica aerosol by allowing silane and oxidizer to mix in the reaction chamber in various ways. We studied the effect of simultaneous mixing of two gases into either an evacuated reaction chamber or one with some background gas in it, introducing an oxidizing gas to pre-existing silane in the reaction chamber and introducing silane to the pre-existing oxidizing gas in the chamber and in some cases various amounts of background gases mixed with the reactants in the reservoirs before mixing in the reaction chamber. We varied the amount of silane being introduced into the reaction chamber to change the silica monomer volume fraction.
The ideal stoichiometries for the chemical reactions with O2 and N2O are
The densities of the silica aerosol gels were determined by dividing the measured masses by the sample volumes. Brunauer–Emmett–Teller (BET) analysis with nitrogen gas as an adsorbate was used to measure the specific surface area of an aerosol gel sample. Microscopic imaging was obtained by spreading small samples of the aerosol gel onto carbon coated electron microscope grids and looking at these with a Philips EM201 transmission electron microscope (TEM) operating at 100 kV. Diffraction data were obtained with wide angle X-ray diffraction (XRD, Scintag XDS-2000 instrument which uses a Cu Kα radiation at 0.1544 nm) and neutron scattering at the NIST Center for Neutron Research (NCNR).
RESULTS AND DISCUSSION
Background Gases
The first experiments were performed at stoichiometry without a background gas, i.e., the silane and oxidizer met in the evacuated reaction chamber and then reacted. The result was a thin, white “paint” layer of silica coated on the inner wall of the chamber instead of particles in an aerosol gel.
This “paint” was an initial surprise. However, it was reasoned that the exothermic reaction yielded silica molecules, or perhaps molten nanoparticles, that moved ballistically in the absence of an inert background gas and “splashed” on the chamber walls. This can explain our finding of the thin silica layer on the inner walls of the reaction chamber when no background gas was used. Subsequent addition of an inert background gas into the reaction chamber before the reactants eliminated the paint and yielded particles.
We propose that the role of the inert background gas was to quickly absorb, after the explosive reaction, the initial high energy of the silica molecules so that they would coalesce to form a nanoparticle aerosol in the background gas. Moreover, the background gas can remove heat from the fresh nanoparticles to bring their temperature below the melting temperature of silica, which is near 1650°C. An alternative explanation, suggested by a referee, is that the background gas lowered the adiabatic flame temperature so that solid particles could form quicker. With the use of background gases, aerosol gels were formed. The aerosol gels are a soft, white material that can be scooped with a spoon from the reaction chamber, examples of which are shown in .
FIG. 2 Silica aerosol gels prepared using oxygen as the oxidizer and nitrogen as a background gas when the silane to oxygen molar ratio was 0.5 (stoichiometric). The dish is 9 cm in diameter.
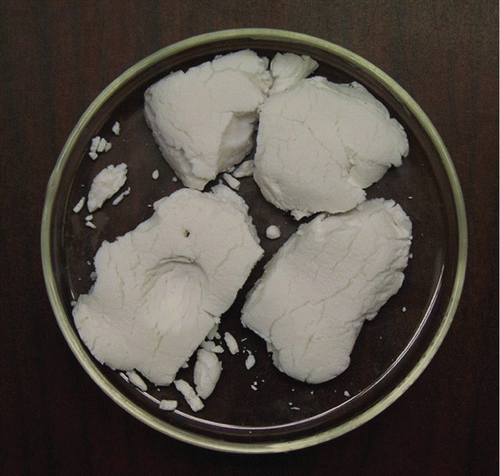
We hypothesized that the use of a heavier background gas with higher thermal conductivity would result in quicker absorption of the initial (at the time of their formation during the explosive reaction) kinetic and thermal energy of the silica nanoparticles. Thus we investigated the effect by using helium (He, mass = 4), nitrogen (N2, mass = 28), and carbon dioxide (CO2, mass = 44), as the background gas. We found no distinguishable difference between the silica aerosol gels using nitrogen and carbon dioxide. The silica aerosol gels in both cases had similar appearance, densities in the range 4– 6 mg/cm3, specific surface area in the range 300–500 m2/g, and similar mean monomer sizes of 22 nm diameter and distribution ± 15%. shows a TEM image of silica aggregates from a silica aerosol gel produced with CO2 as the background gas. The monomers appear to have a narrow size distribution and are more or less rounded with some necking between monomers.
FIG. 3 TEM images of silica aerosol gels prepared using carbon dioxide (left) and helium (right) as the background gas.
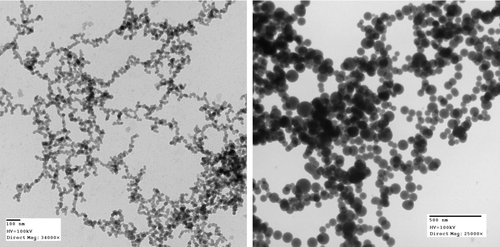
Use of helium, which has a small molar mass, as the background gas yielded a dense-looking white silica aerosol gel in small amounts. We also noticed a thin silica “paint” layer on the inner wall of the chamber. Comparison of TEM images shows that with helium as the background gas, the silica monomers were larger with a mean diameter of 90 nm, more spherical and more polydisperse, ±30%, than for N2 and CO2. With such results, we concluded that decreasing the molecular weight of the background gas caused slower absorption of the initial kinetic and thermal energies of the silica nanoparticles and thus allowed them to coalesce for a longer time (Heinson et al. Citation2010) to become larger.
Variation of the amount of background gas from molar ratios with silane of 0.16–1.0 appeared to affect only the amount of silica produced because as the amount of background gas increased, the amount of silane typically decreased.
Gels
Never was the chamber opened after a reaction to find it completely filled with an aerosol gel. Typically the inner surfaces were coated with the porous silica material layer with thicknesses varying from a few millimeters to a couple of centimeters. Sometimes, roughly spherical, centimeter scale “cotton balls” were found on top of the layer. Thus one might question whether or not a volume spanning aerosol gel ever formed. For carbon aerosol gels, a thickly coated interior was also typically found, but occasionally the chamber was opened to find a volume spanning gel that subsequently collapsed to form a thick layer (Dhaubhadel et al. Citation2007). Perhaps the silica did the same. Regardless, other gel-like properties persist for the silica materials (e.g., they wiggle), so we will continue to apply the term “aerosol gel” to them.
Various Mixings of the Gases
We experimented with premixing either the silane or the oxygen with the nitrogen background gas in the reaction chamber. Premixing silane with the background gas in the explosion chamber yielded fluffier (density 4 mg/cm3) and bluish white aerosol gels. When oxygen was premixed with the background gas in the combustion chamber, a denser (13 mg/cm3) bright white silica aerosol gel was produced.
Brown aerosol gels formed when the silane/molecular oxygen molar ratio was greater than the stoichiometric value of 1/2. This brown color could be due to the presence of free elemental silicon (Si), or, as suggested by a referee, a sub-oxide of silica.
Hydrophilic and Hydrophobic Silica Aerosol Gels
Either hydrophilic or hydrophobic silica aerosol gels were produced by switching from one oxidizer to another. The silica aerosol gels made using oxygen as the oxidizing gas were found to readily absorb water showing its hydrophilic property. However, when we used nitrous oxide or a mixture of nitrous oxide (greater than 90%) and oxygen (less than 10%), white colored silica aerosol gels were formed which were hydrophobic. These results were for nitrogen as the background gas in the explosion chamber. These results are a mystery to us.
Microscopic Structure of the Silica Aerosol Gel
XRD patterns for the silica aerosol gels were essentially the same for all the combinations of silane, oxidizer, background gas, and mixing conditions. An example is given in with a comparison to amorphous silica powder. The patterns are quite similar indicating that the aerosol gel is silica with a low degree of crystalinity, as indicated by the fairly broad diffraction peak. Electron diffraction patterns using the TEM yielded the same interpretation.
FIG. 4 X-ray diffraction patterns for the silica aerosol gel (upper curve) and powdered amorphous silica.
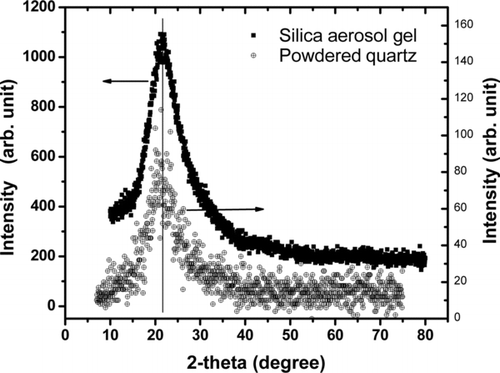
Matching of the peaks confirmed the material of the aerosol gel to be definitely silica. Broad peak indicated low degree of crystalline for the silica aerosol gel.
Morphology of the Silica Aggregates
The TEM pictures of the silica aerosol gels () show that the gels consist of networks with ramified structures. To study the gel structure deeper, the aerosol gel was studied with neutron scattering at NCNR. A silica aerosol gel was prepared using a molar ratio of silane to oxygen of 0.5 with nitrogen as the background gas. A bulk sample removed from the reaction chamber was shipped to NCNR where both ultra small angle neutron scattering (USANS) and small angle neutron scattering (SANS) data were obtained from the sample. USANS and SANS can together provide q-range of approximately 4 × 103 to 1 × 107 cm−1. The results are shown in .
FIG. 5 Combined USANS and SANS result from a collapsed silica aerosol gel sample. Scattered neutron intensity I(q) is plotted versus the scattering wave vector q. Clearly evident are power-law regimes: ∼q −1.75 at intermediate q indicative of a mass fractal aggregate and ∼q −4 at high q indicative of the 3d monomer structure. Stronger power-law decay of scattered intensity at low q can be explained as an artifact of the collapsing of the aerosol gel.
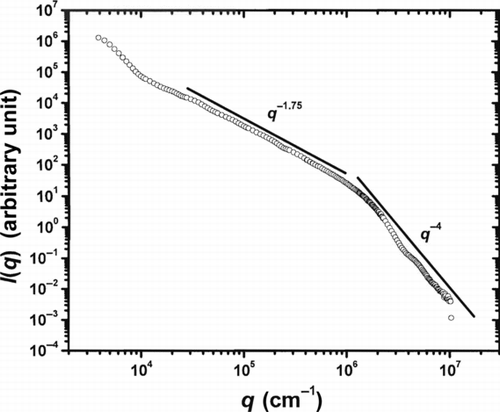
shows scattered neutron intensity I(q) plotted versus the scattering wave vector q. The power-law regime of q −1.75 at intermediate q (corresponding to a length scale range of 10–1000 nm) indicates a mass fractal morphology with a fractal dimension of Df = 1.75. This fractal dimension is consistent with the DLCA value (Meakin Citation1988). At high q (length scales below 10 nm), the data show a q −4 power law which can be interpreted as the Porod regime of the smooth surface, 3d silica monomers. A weak ripple observed in the Porod regime could be due to a narrow size distribution for monomers (Rieker et al. Citation1999). The TEM pictures () showing a uniform monomer size distribution support this observation. The stronger power-law decay of the scattered intensity at low q can be explained as the appearance of superaggregates with a fractal dimension of ca. 2.6, which we have shown can occur in dense aerosols near the gel point (Sorensen et al. Citation2003; Kim et al. Citation2004, Citation2006). On the other hand, this could also be an artifact of the collapsing of the aerosol gel while shipping and handling to NCNR (Sorensen et al. Citation1998b).
CONCLUSION
Silica aerosol gels were created via a controlled detonation of silane gas with either oxygen or nitrous oxide in a chamber to create nanoparticles followed by aggregation of the nanoparticles to form gels. The materials so produced had densities ranging from 4 to 15 mg/cm3 and surface areas in the range 300–500 m2/g. The monomeric nanoparticles were amorphous silica particles with diameters in the range 22–90 nm depending on the background gas. The prevailing submicron structure was fractal with a dimensionality of 1.75.
This work extends our previous work which created carbon aerosol gels and thereby implies that the aerosol gelation method can be applied to a wide variety of materials.
Declaration of interest: Any opinion, finding, and conclusions or recommendations expressed in this material are those of the authors and do not necessarily reflect the views of the National Science Foundation.
Acknowledgments
This work was supported by NSF grant CTS 0080017. T. P. Rieker acknowledges NSF support, while working at the Foundation.
REFERENCES
- Brinker , C. J. and Scherer , G. W. 1990 . Sol-Gel Science: The Physics and Chemistry of Sol-Gel Processing , Boston : Academic Press .
- Dhaubhadel , R. , Gerving , G. S. , Chakrabarti , A. and Sorensen , C. M. 2007 . Aerosol Gelation: Synthesis of a Novel, Lightweight, High Specific Surface Area Material . Aerosol Sci. Technol. , 41 : 804 – 810 .
- Heinson , W. R. , Sorensen , C. M. and Chakrabarti , A. 2010 . Computer Simulation of Aggregation with Consecutive Coalescence and Non-Coalescence Stages in Aerosols . Aerosol Sci. Technol. , 44 : 380 – 387 .
- Hüsing , N. and Schubert , U. 1998 . Aerogels—Airy Materials: Chemistry, Structure, and Properties . Angew. Chem. Int. Ed. , 37 : 22 – 45 .
- Kim , W. G. , Sorensen , C. M. and Chakrabarti , A. 2004 . Universal Occurrence of Soot Aggregates with a Fractal Dimension of 2.6 in Heavily Sooting Laminar Diffusion Flames . Langmuir , 20 : 3969 – 3973 .
- Kim , W. G. , Sorensen , C. M. , Fry , D. and Chakrabarti , A. 2006 . Soot Aggregates, Superaggregates and Gel-Like Networks in Laminar Diffusion Flames . J. Aerosol Sci. , 37 : 386 – 401 .
- Meakin , P. 1988 . Fractal Aggregates . Adv. Coll. Interface Sci. , 28 : 249 – 331 .
- Rieker , T. P. , Hanprasopwattana , A. , Datye , A. and Hubbard , P. 1999 . Particle Size Distribution Inferred from Small-Angle X-Ray Scattering and Transmission Electron Microscopy . Langmuir , 15 : 638 – 641 .
- Sorensen , C. M. and Chakrabarti , A. 2011 . The Sol to Gel Transition in Irreversible Particulate Systems . Soft Matter , 7 : 2284 – 2296 .
- Sorensen , C. M. , Hageman , W. B. , Rush , T. J. , Huang , H. and Oh , C. 1998a . Aerogelation in a Flame Soot Aerosol . Phys. Rev. Lett. , 80 : 1782 – 1785 .
- Sorensen , C. M. , Oh , C. , Schmidt , P. W. and Rieker , T. P. 1998b . Scaling Description of the Structure Factor of Fractal Soot Composites . Phys. Rev. , 58 : 4666 – 4672 .
- Sorensen , C. M. , Kim , W. , Fry , D. and Chakrabarti , A. 2003 . Observation of Soot Superaggregates with a Fractal Dimension of 2.6 in Laminar Acetylene/Air Diffusion Flames . Langmuir , 19 : 7560 – 7563 .