Abstract
In this study, a new computational fluid dynamics (CFD) modeling approach for pharmaceutical aerosols is further developed by evaluating the effects of turbulence, polydisperse aerosol size distribution, and multiple lung lobes on deposition in the mouth–throat (MT) and entire tracheobronchial (TB) airways. To evaluate a range of respiratory drug delivery conditions, a model dry powder inhaler (DPI; NovolizerTM) and a model spray soft-mist inhaler (SMI; RespimatTM) were considered. The respiratory geometry consisted of a previously developed characteristic MT and complete upper TB geometry through the third bifurcation (B3). More distal TB airways were simulated using stochastic individual path (SIP) models extending into each of the five lung lobes through bifurcation B15. Based on comparisons with new in vitro deposition data, results indicated that the low Reynolds number (LRN) k–ω turbulence model with near-wall corrections for anisotropic turbulence and velocity conditions accurately predicted deposited drug mass from both inhalers. Simulating the polydisperse aerosol size distribution had a large impact on overall deposited drug mass compared with a monodisperse approximation. However, a new equivalent mass median diameter (MMD), calculated as MMDeq = 1.25 × MMD, was shown to provide a good monodisperse estimate of the aerosol deposition from both inhalers considered in this study used with different inhalation flow conditions in the SIP models. Large variations in deposition were observed among the five lung lobes. However, deposition in the left lower lobe (scaled by a factor of 5) was found to be characteristic of total lobar deposition in the range of B4–B15 and allowed for the simulation of only one lobe. Implementing the approximations of an equivalent MMD and simulating deposition in one characteristic lung lobe increased the efficiency of the airway CFD simulations by over 25 times at the expense of a maximum 12% relative error compared with the more exact simulations. As an example of model utility, results indicated that the state-of-the-art SMI improved delivery efficiency of drug by a factor of 1.5 in the upper TB region and by over an order of magnitude in the lower TB airways compared with a commonly used high quality DPI.
Copyright 2012 American Association for Aerosol Research
INTRODUCTION
Predicting the deposition of aerosols in the mouth–throat (MT) and tracheobronchial (TB) airways is critical for accurate estimates of total and regional lung doses from inhaled pollutants and inhaled pharmaceutical products. As recently reviewed by Longest and Holbrook (Citation2012), both algebraic, or one-dimensional (1-D), models and computational fluid dynamics (CFD) simulations are effective means to predict the deposition of inhaled aerosols throughout the airways. Compared with algebraic correlations, CFD simulations offer the advantage of directly accounting for factors that complicate the transport and deposition of pharmaceutical aerosols (Longest and Holbrook Citation2012), such as spray momentum (Longest et al. Citation2008), turbulent inlet jets (Matida et al. Citation2003), transient inhalation and spray effects (Longest et al. Citation2009), evaporation and condensation (Longest et al. Citation2007), and polydisperse aerosol size (Shi and Kleinstreuer Citation2007). Computational fluid dynamics simulations also provided the advantage of predicting highly localized deposition profiles, which can be used to more accurately estimate cellular-level doses of drugs (Balashazy et al. Citation1999; Longest et al. Citation2006). A disadvantage of CFD simulations is that they are typically limited to segments of the respiratory airways due to the computational power required to generate accurate grid-independent results (Vinchurkar and Longest Citation2008). Recent studies have reported CFD simulations of increasingly larger sections of the airways, such as from the nose or mouth to the third airway bifurcation and beyond (Longest and Xi Citation2008; Xi et al. Citation2008; Ma and Lutchen Citation2009; Lambert et al. Citation2011; Zhang and Kleinstreuer Citation2011). However, CFD simulations of the entire airway tree or even the TB airways, which extend to approximately the 15th bifurcation, are currently too computationally expensive for grid-independent solutions (Longest and Vinchurkar Citation2007) of complex flow dynamics and may not be an effective use of future computational resources (Longest and Holbrook Citation2012).
Instead of modeling all branches in the TB region, recent CFD simulations have formulated approximations to capture deposition characteristics across multiple scales. In a series of papers, Kleinstreuer and Zhang et al. (Zhang et al. Citation2008, 2009; Kleinstreuer and Zhang Citation2009) constructed CFD models to represent the entire TB airways using symmetric triple bifurcation units repeated in series and in parallel. Based on this model, Kleinstreuer and Zhang (Citation2009) reported that turbulent conditions could be neglected beyond approximately the 5th respiratory generation (G5) for the deposition of monodisperse aerosols during the inhalation of ambient air at 30 LPM. Zhang et al. (Citation2008) reported that fully developed flow and uniform particle concentrations could be assumed for the deposition of nanoparticles beyond G12. However, one disadvantage of this modeling approach is that it may be difficult to track an evolving group of polydisperse droplets from the inlet of a pharmaceutical inhaler to the point of deposition or model exit as size change due to evaporation and condensation occurs or for two-way coupled heat and mass transfer. Longest and colleagues recently reported a CFD-based stochastic individual path (SIP) modeling approach to predict the delivery of pharmaceutical aerosols throughout the TB airways (Tian et al. Citation2011a,b). In this approach, aerosol transport and deposition are modeled from the inhaler through the MT and to the third respiratory bifurcation of a complete asymmetrical characteristic geometry. Thereafter, individual pathways are stochastically generated in which one branch of each bifurcation is continued and one is not. As a result, three-dimensional stochastic paths are built to characterize deposition throughout the TB airways in a highly efficient manner. Tian et al. (Citation2011a) used this modeling approach to characterize the growth of pharmaceutical aerosols delivered with a newly proposed enhanced condensational growth (ECG) method. For a dry powder inhaler (DPI), Tian et al. (Citation2011b) showed that deposition in the upper airways through approximately the third bifurcation (B3) was affected by the use of a realistic transient inhalation waveform compared with a steady state approximation. In the SIP models starting with the fourth bifurcation (B4), steady state approximations were highly accurate and one SIP geometry was found to sufficiently represent the deposition in an individual lobe of the lung. In comparison with performing fully transient simulations of all branches in the regions considered, the SIP modeling approach reduces computational effort by a factor of approximately 3 × 105 while predicting regional and local deposition values to within approximately 5%.
The SIP approach developed in the studies of Tian et al. (Citation2011a,b) represents a highly effective method that is capable of accounting for a number of factors related to the delivery and deposition of pharmaceutical aerosols. However, simulating the transport and delivery of pharmaceutical aerosols from the site of formation to deposition in the TB or alveolar airways remains highly complex and time consuming. A primary challenge is developing a sufficiently accurate turbulence approximation that can simulate aerosol transport starting within the pharmaceutical inhaler (including both spray and dry powder devices) and then resolve turbulence decay and particle dispersion through the MT and decreasing geometric scales of the respiratory airways. The current trend in simulating airway turbulence is in the direction of using more complete models such as direct numerical simulation (DNS) and large eddy simulation (LES) (Matida et al. Citation2006; Jin et al. Citation2007; Lin et al. Citation2007; Jayaraju et al. Citation2008; Lambert et al. Citation2011). However, these more complex turbulence models have difficulty in simulating relatively large time scales, such as an inhalation period (e.g., 5 s) or spray duration (e.g., 0.1–1.5 s), which are known to influence the deposition of pharmaceutical aerosols (Longest et al. Citation2009; Tian et al. Citation2011b). Other factors that may complicate the simulation of both pharmaceutical and ambient aerosols include the consideration of polydisperse aerosol size distributions (Martonen and Katz Citation1993) and evaluating transport within multiple lobes of the lungs (Asgharian and Price Citation2006). Simulating polydisperse aerosols becomes difficult in bifurcating airways with the SIP or any modeling approach as the number of particles is cut in approximately half with each new airway generation. As a result, a very large number of initial particles are required at the inlet to fully resolve regional and local deposition across all size bins of the polydisperse distribution. Considering individual lobes of the lungs, Tian et al. (Citation2011b) indicated that one SIP geometry was sufficient per lobe to characterize branch-averaged and maximum local deposition. However, comparisons among lung lobes were not considered. In summary, a better understanding of these factors and their effects on deposition will lead to improved modeling of aerosol transport throughout the MT and TB airways using a SIP or other simulation approach to resolve large regions of the lungs. Further details on modeling turbulence, polydisperse aerosols, and considering deposition in multiple lung lobes are considered in the online supplemental information.
The objective of this study is to evaluate the effects of turbulence, polydisperse aerosol size distribution, and multiple asymmetrical lung lobes on pharmaceutical aerosol deposition in the MT and entire TB airways. To evaluate a range of respiratory drug delivery conditions, a model DPI (NovolizerTM) and a model spray soft-mist inhaler (SMI) (RespimatTM) are considered. The respiratory geometry consists of a previously developed characteristic MT and complete upper TB geometry through the third bifurcation. More distal TB airways are simulated using the SIP modeling approach extending into each of the five lung lobes through bifurcation B15. Model predictions of deposition are validated with comparisons to new in vitro experimental data of drug deposition from the inhalers considered, actuated into the exact MT and upper TB geometries used in the CFD simulations. The quality of the low Reynolds number (LRN) k–ω model with a near-wall anisotropic turbulence correction is evaluated through comparisons to the in vitro deposition results. Effects of turbulent particle dispersion vs. laminar particle tracking and polydisperse vs. monodisperse aerosol simulations are evaluated within individual sections of the conducting airways. Depositions within individual lobes of the lungs are then compared and used to suggest a single lobe approximation (with quantified variability) to characterize conventional pharmaceutical aerosol delivery to the TB airways. Results of this study are intended to help establish best practices for simulating polydisperse pharmaceutical aerosols delivered from real inhalers to the MT and TB regions of the airways in terms of selecting an adequate turbulence model that can resolve transient flow fields, establishing when and if monodisperse particles can be assumed, and evaluating variability in interlobe predictions of aerosol delivery.
METHODS
Inhalers and Airway Models
In order to consider a range of pharmaceutical aerosols and device types, both a DPI and an SMI were selected. Compared with metered dose inhalers (MDIs), both DPIs and SMIs are gaining market share based on improved ease of use, reduced dose variability, and increased drug delivery efficiency to the lungs (Smith et al. Citation2010). The characteristic DPI selected for this study was the Novolizer (Meda Pharmaceuticals, Somerset, NJ, USA), which was previously described in the study of Fenton et al. (Citation2003). In the current study, the salbulin Novolizer was implemented, which delivered 120 μg of albuterol sulfate (AS). The drug (AS) is stored in the device as a powder that is blended with much larger (approximately > 50 μm) lactose carrier particles. Delvadia et al. (Citation2012) recently considered a similar budelin Novolizer combination, which delivered 200 μg of budesonide, and evaluated MT, upper TB, and lung delivery with in vitro experiments using a replica model very similar to the one implemented in the current study. Delvadia et al. (Citation2012) showed that in vitro deposition characteristics in the MT and lung were very similar to the in vivo data reported by Newman et al. (Citation2000) and scaling of the MT–TB model could account for intersubject variability. The Novolizer device forms an aerosol by passing air over a metered mass of powder (drug and carrier), with the initially formed aerosol passing through a cyclone impactor and then exiting the inhaler through a 6 mm diameter jet. The jet is located in the middle of an oval 20 × 25 mm mouthpiece, which serves to maintain an open mouth position when using the inhaler. The numerical model of the Novolizer consists of this oval geometry with a central 6 mm jet entering the MT ().
FIG. 1 Inhalers and airway models including the (a) Novolizer dry powder inhaler (DPI) mouthpiece with a 6 mm diameter inlet jet, (b) Respimat soft-mist inhaler (SMI) with inlet flow surrounding a central post and microjet, and (c) MT and TB models through the conducting airways. The mouthpieces were connected to the MT model to evaluate the two inhalers. The TB model was complete through the third bifurcation (B3) and then continued with one SIP geometry entering each of the five lung lobes and ending with B15.
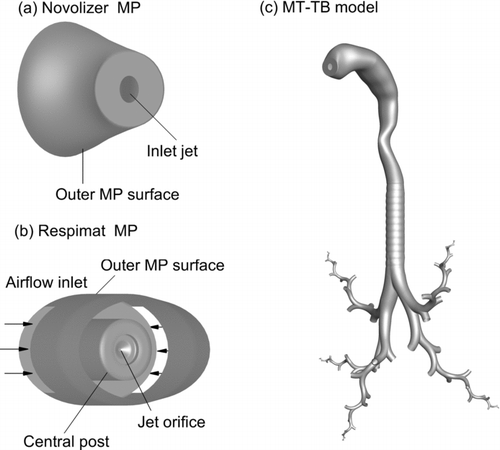
The characteristic spray device was the Respimat SMI (Boehringer Ingelheim, Ingelheim, Germany), which was previously described in the studies of Dalby et al. (Citation2004) and Longest and Hindle (Citation2009). This device forms an aerosol by colliding two microscale streams of liquid drug solution at a predetermined angle (approximately 90o), which creates breakup of the liquid columns and a spray effect. The device typically delivers 15 μL of solution over a period of 1.5 s, which is considerably longer than with MDI spray devices (∼0.2 s) (Hochrainer et al. Citation2005). In the current study, the Respimat delivers 15 μL of a solution containing AS at a concentration of 0.6% w/v in an aqueous vehicle. The mouthpiece (MP) of the inhaler contains side inlet vents through which the inhaled air passes. A central post within the MP contains the collision nozzle for forming the aerosol. Longest and Hindle (Citation2009) previously showed that recirculating airflow in front of the central post (in the region of aerosol formation) was partially responsible for high device deposition values. The numerical model of the Respimat MP used in the current study is illustrated in and contains the side air vents, central post, and an oval 17 × 26 mm outlet that connects to the MT inlet.
The inhalers were connected to a characteristic MT geometry based on the elliptical model originally proposed by Xi and Longest (Citation2007) (). This MT model was developed from the oral airway cast reported by Cheng et al. (Citation1997) and in-house CT data of the pharynx and larynx. The original inlet diameter of the MT model was 22 mm, which was considered similar to the top-to-bottom mouthpiece diameters of both inhalers. As a result, changes to the oral airway volume were not implemented to account for changes in the jaw position. The inhalers were directly connected to the MT geometry assuming an insertion depth of 0.5 cm and an angle of zero degrees with the horizontal plane. This insertion depth creates a small difference from the previous in vitro and numerical studies of Delvadia et al. (Citation2012) and Longest and Hindle (Citation2009). However, MP insertion only accounts for a small portion of the oral cavity length with these inhalers and is expected to have a very minor effect on the results. The MT volumes for the final DPI and Respimat geometries were 57.8 cm3 and 63.3 cm3 through the larynx (excluding mouthpieces), respectively.
The upper TB airways are represented with a characteristic model extending through the third bifurcation (). This asymmetrical model was based on the anatomical cast dimensions reported by Yeh and Schum (Citation1980) and scaled to a functional residual capacity (FRC) of 3.5 L, which is consistent with an adult male (ICRP Citation1994). The individual bifurcation units were generated using the physiologically realistic bifurcation (PRB) parameters reported by Heistracher and Hofmann (Citation1995). For the in vitro validation experiments and corresponding CFD simulations, a circular and smooth tracheal geometry was implemented. In all other cases, cartilaginous rings were included in the trachea and a D-shaped tracheal cross-section was implemented. Dimensions of these geometric features were based on in-house medical scans and the cadaver measurements of Russo et al. (Citation2008), as described by Tian et al. (Citation2011b). Previous studies have highlighted the importance of cartilage rings on deposition (Martonen et al. Citation1994; Zhang and Finlay Citation2005; Russo et al. Citation2008); however, this effect is likely a function of the ring dimensions and particle sizes considered. Further details of the upper TB model are provided in the supplemental information.
Beyond the third bifurcation, SIP models were considered extending into each of the five lung lobes (). Bifurcations within the SIP models were constructed as PRB units with the airway dimensions reported by Yeh and Schum (Citation1980), again scaled to a FRC of 3.5 L. An advantage of the Yeh and Schum (Citation1980) data is that average dimensions are provided for each lung lobe. Use of the individual path model based on PRB units allows for the application of a hexahedral mesh, which improves solution accuracy, computation speed, and requires fewer cells to adequately resolve the flow domain (Vinchurkar and Longest Citation2008). Based on available data, the bifurcations in the individual path model section (B4–B15) were symmetric and included a symmetric outflow assumption at each bifurcation level. Continuation of the left or right branch of each bifurcation was considered to be consistent with the flow distribution, resulting in an equal probability for the symmetric outflow assumption. However, if a selection led out of the general region of an initial lung lobe, the other branch was selected. Consecutive branches were rotated at 90o to approximate physiological conditions (Phalen et al. Citation1978). Bifurcation 15 was assumed to end with the terminal bronchioles in each lobe, based on existing anatomical data (Yeh and Schum Citation1980). The study of Tian et al. (Citation2011b) indicated that one SIP model could resolve the regional and bifurcation-averaged deposition efficiency (DE) in a lung lobe to within a relative error of approximately 5% for symmetric bifurcations. As a result, one SIP model was considered sufficient to characterize deposition within each of the five lung lobes.
CFD Model and Numerical Solution
Reynolds numbers based on inlet and parent diameters range between 744 and 15,300 within the inhaler mouthpieces and upper MT–TB model for the characteristic devices and inhalation conditions considered in this study. In the individual SIP geometries, characteristic Reynolds numbers range from approximately 2010 at the inlet (B4) to 270 at B8 and 90 at B15. Considering the presence of inlet jet flow, the larynx, and bifurcations, laminar through fully turbulent conditions are expected. Simulating transition from turbulent to laminar flow is especially critical with the SIP approach to accurately capture deposition in the upper and middle bronchi. To resolve these multiple flow regimes, the LRN k–ω model was selected based on its ability to effectively and accurately predict pressure drop, velocity profiles, and shear stress for transitional and turbulent flows (Ghalichi et al. Citation1998; Wilcox Citation1998). Another significant advantage of the LRN k–ω model is numerical efficiency, which allows for the simulation of typical SMI spray times (Longest et al. Citation2009) and transient inhalation profiles (Xi et al. Citation2008; Tian et al. Citation2011b). The conservation of mass and momentum equations used with the LRN k–ω model are available from Wilcox (Citation1998) and were previously reported by Longest and Xi (Citation2008). Similarly, the equations governing turbulent kinetic energy (k) and specific dissipation rate (ω) were also reported by Longest and Xi (Citation2008). The LRN k–ω model resolves the flow field through the buffer and viscous sub-layers in transitional and turbulent boundary layers and internal flows (Wilcox Citation1998), provided that the near-wall mesh spacing is sufficient.
Based on the aerosol size experiments, the polydisperse distributions include particles ranging from the submicrometer scale to greater than 10 μm. To address this broad range of particle sizes, a Lagrangian particle tracking method was employed (Longest et al. Citation2004; Longest and Xi Citation2007), as described in the supplemental information.
To model the effects of turbulent fluctuations on particle trajectories, i.e., turbulent particle dispersion, a random walk method was employed (Gosman and Ioannides Citation1981; Loth Citation2000; Matida et al. Citation2000). The primary limitation of this eddy interaction model in conjunction with the Reynolds averaged Navier Stokes (RANS) equations is that it does not account for reduced turbulent fluctuations in the wall-normal direction, which may result in an overprediction of deposition (Kim et al. Citation1987; Matida et al. Citation2000, 2004; Tian and Ahmadi Citation2007). To better approximate turbulent effects on particle deposition, an anisotropic turbulence correction was applied for particle tracking where the near-wall fluctuating velocity is calculated as (Wang and James Citation1999; Matida et al. Citation2004)
Based on the results of Tian et al. (Citation2011b), transient simulations are required in the upper airways through approximately B3, whereas steady state approximations are acceptable in the SIP models. In this study, transient simulations are conducted for the inhalers, MT and upper TB airways for the complete SD and QD waveforms. For the individual SIP models, steady state simulations are then performed at the equivalent mean flow rates (Figure S1, see online supplemental information). In these steady state simulations, the inhalers and upper MT–TB models are included to generate approximate flow fields and particle conditions at the inlet to each of the SIP geometries. Deposition efficiencies determined with the transient simulations for the MT–TB model are then combined with the SIP values to characterize deposition throughout the conducting airways. Tian et al. (Citation2011b) showed that this approach was accurate to within 5% of fully transient simulations in both the upper and lower airways, but provided a significant savings in solution time.
Computational fluid dynamics simulations were performed using a combination of the commercial code Fluent 12 (ANSYS, Inc.) and user-defined functions for calculating near-wall particle interpolations (Longest and Xi Citation2007), anisotropic turbulence corrections (Longest et al. Citation2008), and deposition fractions (DFs). In performing the CFD simulations, previously established best practices were implemented to provide a high quality solution, as described in the supplemental information. For the MT–TB model, including one SIP geometry, grid convergent results were found to occur with meshes consisting of approximately 2.0 million control volumes. Specifically, coarse, medium, and fine grids of approximately 850,000, 1,250,000, and 2,000,000 control volumes were considered. Maximum velocity values and DFs varied by less than 2% between the two highest grid densities considered. As a result, the fine grid was implemented in this study. The near-wall mesh spacing was approximately 0.18 mm in the upper airways. The wall y+ value was between 0 and 11 in the MT–TB region, and between 0 and 2.4 in the region of B4–B7. To improve the accuracy of particle deposition predictions in the turbulent flow fields, near-wall interpolation of the velocity profile was included, as described by Longest and Xi (Citation2007).
Two inhalers and two types of particle distribution profiles (monodisperse vs. polydisperse) were considered. For both inhalers and the monodisperse assumption, approximately 80,000 particles at the inlet of the Novolizer and Respimat were required to produce convergent deposition results. Model sections included the inhaler, B4 and B8. Nine size bins consistent with the midpoint sizes of the Next Generation Impactor (NGI) stages implemented at 75 LPM were considered for the polydisperse aerosol. To resolve deposition in all size bins, 450,000 particles were considered at the inlet of each model section. These values were determined as the number of required particles to reduce change in local DFs to below 5% relative difference with the addition of 10,000 monodisperse particles and 50,000 polydisperse particles.
Deposition Fractions
Deposition within regions of the respiratory tract can be reported as either a fraction or efficiency. The DF for the i-th region based on drug mass is calculated as
The corresponding DE for region i is calculated as
To determine a total DF, DF i values in individual regions can be directly summed. Deposition efficiency values are an effective method to report the characteristics of an individual section of the respiratory tract without the influence of upstream aerosol losses. In this study, DE values are calculated for each bifurcation based on deposited drug mass. Sectional DE values are then computed by combining the individual bifurcation values (ICRP Citation1994). For example, the DE in region B4–B15 within a single lung lobe is calculated as
The DF within an individual lung lobe is then calculated as
In this study, the deposition of a polydisperse pharmaceutical drug aerosol is considered. The expected size range of the drug particles, based on the impactor used in the experiments, is 0.145–12 μm with a mass median aerodynamic diameters (MMADs) of approximately 2–5 μm. However, simulating the correct number fraction of particles directly would result in an excessive number of submicrometer particles and unreasonable computational times. Instead, an equal number of particles are simulated across nine size bins. Deposition efficiencies within each size bin are calculated. These deposition efficiencies are then combined with the polydisperse aerosol distribution from the particle sizing experiments to determine the DE or DF of drug mass in each section of the geometry.
Inlet and Outlet Conditions
Typical recommended instructions for most DPIs and SMIs are to inhale “quickly and deeply” and “slowly and deeply,” respectively. Characteristic inhalation waveforms to represent quick and deep (QD) and slow and deep (SD) breathing with these inhalers are needed. Inhalation waveforms during inhaler use have recently been recorded and reported by Byron et al. (Citation2010), Delvadia et al. (Citation2010), and Olsson et al. (Citation2010). Representative QD and SD inhalation profiles for the Novolizer and Respimat devices, respectively, were established based on previous studies as described in the online supplemental information and Figure S1.
To facilitate the CFD solution, the airway model was divided into three sections, which were the inhaler to B3 (including the complete asymmetrical upper TB region), B4–B7 (middle TB airways), and B8–B15 (lower TB airways). Both velocity and particle inlet conditions were required for simulating each section. At the inhaler inlets, airflow entered the central jet of the Novolizer as a blunt profile and the side vents of the Respimat with constant velocity values. Particles were introduced uniformly over the DPI inlet jet area with velocities equal to the local air conditions. For the Respimat, particles were injected over a small area consistent with the nozzle outlet and with velocity conditions predicted by the study of Longest and Hindle (Citation2009) to match the spray plume travel velocity data of Hochrainer et al. (Citation2005). Based on expected performance of the Novolizer DPI and Respimat SMI, release times of the particles in the simulations were between 0–0.5 s and 0–1.5 s, respectively. At the inlets to B4 and B8, outlet profiles of velocity and turbulence quantities from B3 and B7, respectively, were implemented. Interpolation was not needed when translating from outlet to inlet profiles as control volume center locations were exactly matched between the structured grids. Particle injection profiles at inlets to B4 and B8 were either determined from upstream outlet conditions or approximated as blunt at B4 and parabolic at B8. Test results indicated that these approximate profiles produced sectional deposition results very similar (within 5%) to the more exact outlet profiles, and were therefore used. Use of monodisperse vs. polydisperse distributions for each of these inlet particle profiles was considered in the current study.
Outflow boundary conditions in the upper TB model were based on estimates of ventilation to each lung lobe. Lobar ventilation approximations presented in the studies of Horsfield et al. (Citation1971), Asgharian and Price (Citation2006), and Yin et al. (Citation2010) were considered. Reasonable consistency among these studies led to the following distribution estimates for each of the five lung lobes: right upper (RU) 14%, right middle (RM) 7%, right lower (RL) 33%, left upper (LU) 15%, left lower (LL) 31%. The resulting right and left ventilation proportions were 54% and 46%, respectively. Beyond the lobar bronchi, symmetric outflow conditions were assumed at each bifurcation level (B4–B15). This assumption is justified based on an association between branch diameter and downstream volume in the airways. However, symmetric outflow conditions do not result in symmetric particle divisions through each outlet. This is because the particle profiles entering each bifurcation are skewed based on upstream transport and deposition, especially from the upstream asymmetrical region, and because of asymmetrical settling and deposition in the lower airways.
Experimental Determination of Initial Polydisperse Size Distributions
For sizing the Novolizer DPI, a Next Generation Impactor (NGI; MSP Corp., Shoreview, MN, USA) was operated at a flow rate of 75 LPM, which is consistent with the QD inhalation waveform described previously. The Novolizer DPI was connected directly to a preseparator using a mouthpiece adaptor to ensure an airtight seal and the flow was generated using a vacuum pump. A three-way solenoid valve downstream of the impactor was used to generate a square wave inhalation waveform of 75 LPM for 3.2 s to actuate the DPI and draw a total 4 L of air through the system. Impactor stages and the preseparator were coated to prevent particle bounce and re-entrainment. Drug deposition in the impactor was determined using a validated high performance liquid chromatography (HPLC) assay method for AS for four single dose experiments. Particle size distributions were reported as AS mass distribution recovered from the impactor. The MMAD was defined as the particle size at the 50th percentile on a cumulative percent mass undersize distribution (D50) using linear interpolation.
Measured particle size distributions were translated to size bins in the numerical model using the midpoint diameters of the NGI stages for a flow rate of 75 LPM. Drug mass in the NGI preseparator was assumed to have the preseparator cut-off diameter of approximately 12 μm. It is expected that a fraction of this aerosol in the preseparator is composed of larger diameter particles due to some drug remaining attached to lactose carrier particles. However, both 12 μm and larger particles are typically retained in the MT region. As a result, MT and TB partitioning of drug will be similar to partitioning values that would occur from a more exact fractioning of sizes above 12 μm. The quality of this assumption will be evaluated with comparisons between the CFD predictions and in vitro estimates of drug deposition for the DPI.
Considering the Respimat inhaler, the previously determined size distribution reported by Longest and Hindle (Citation2009) was used in the CFD model. Briefly, a laser diffraction system (Spraytec, Malvern Instruments Inc., Southborough, MA, USA) was implemented to determine the size distribution of the spray as it exited the Respimat spray nozzle (Longest and Hindle Citation2009). The mouthpiece structure was cut away from the inhaler to prevent interference with the sizing procedure. To assess the size distribution in a relatively stable section of the aerosol plume, the center of the laser was positioned 1.5 cm away from the spray nozzle unit and 30 LPM of co-flow air was pulled around the nozzle during size analysis. The measured particle size distribution was translated to size bins in the CFD model using midpoint diameters of the NGI stages, as with the DPI distribution.
Experimental Determination of Deposited Drug Mass
An exact replica of the MT–TB model used in the CFD simulations through B3 was constructed using an in-house rapid prototyping facility (Viper SLA Machine; 3D Systems, Valencia, CA, USA). The model was constructed with clear Accura 60 plastic resin using a build layer thickness of 0.15 mm and a laser spot diameter of 0.25 mm. The MT–TB model was installed in a custom-built Plexiglas housing with minimal dead space and connected to a three-way solenoid valve and vacuum pump as previously described by Byron et al (Citation2010). During each test, the inhaler was primed and inserted into the mouth opening. For both inhalers, an airtight seal between the inhaler mouthpiece and the MT–TB model was maintained.
In testing the Novolizer, the internal surfaces of the MT–TB model were coated with a glycerol:methanol (1:2 by volume) mixture, followed by methanol evaporation before each experiment. A low resistance filter was placed inline to retain aerosolized drug that passed through the model and exited the Plexiglass chamber. As with the initial particle sizing experiments, a square wave inhalation profile of 75 LPM for 3.2 s was employed to actuate the Novolizer DPI and deliver aerosol to the geometry. Drug mass retained in the MT, TB, and Plexiglas housing plus filter was calculated following recovery using a wash solution and analysis for AS by a validated HPLC assay method.
For the Respimat inhaler, coating of the airway model was not required to evaluate the sectional deposition of droplets. A steady state flow rate of 30 LPM was generated through the inhaler and MT–TB model using a downstream vacuum pump. The Respimat inhaler was then fired into the steady state airstream. Drug deposition in the MT–TB model and remaining geometry (chamber, filter) was then determined using washing and a validated HPLC technique for AS, as with the DPI.
RESULTS
In Vitro Analysis
Excluding the preseparator, which is common practice in pharmaceutical aerosol analysis, the Novolizer aerosol had an MMAD of 1.96 μm (standard deviation [SD] = 0.03) and a geometric standard deviation (GSD) of 1.73. The mass fraction distribution of the Novolizer aerosol based on analysis with the NGI operated at 75 LPM is reported in Figure S2. For the CFD simulations, the fraction deposited in the preseparator was assigned a diameter of 12 μm and included in the cumulative mass distribution, resulting in an MMAD of 5.75 μm (GSD = 1.78). In the simulations, geometric aerosol size was implemented and the aerosol was assumed to consist entirely of separated drug (AS) particles with a density of 1.340 g/cm3. The resulting mass median diameter (MMD) of the aerosol was 4.97 μm at the oral inlet, which was implemented as the monodisperse size of the polydisperse Novolizer aerosol. This approximation will be compared with polydisperse deposition to determine if it is adequate, or if a correction factor is needed to define an equivalent monodisperse size. For the Respimat, Longest and Hindle (Citation2009) previously reported that the aerosol with AS had a MMD of 5.3 μm (GSD = 1.61), based on laser diffraction measurements.
Deposition of drug mass in the MT and upper TB airways from the Novolizer and Respimat inhalers is reported in based on constant flow rates of 75 and 30 LPM, respectively, and in vitro experiments. As with most jet-based DPIs, the Novolizer delivers a majority of the drug dose to the MT region (Newman and Busse Citation2002; Zhang et al. Citation2007). In contrast, the Respimat significantly reduces MT deposition, but has a high mouthpiece DF. Minor differences in device and MT deposition values between these current in vitro results and the previous experimental results of Delvadia et al. (Citation2012) and Longest and Hindle (Citation2009) arise from the increased inhaler insertion depth in the current study and, in the case of the Novolizer, use of different drug formulations as well as waveforms. Due to the high MT deposition of the Novolizer combined with the overall small particle size, drug deposition in the first three bifurcations of the TB region is low. In contrast, the Respimat delivers approximately three times more drug to the upper TB airways, based on the in vitro experiments.
Validation of CFD Simulations and Upper Airway Deposition
For both inhalers, CFD predictions closely match the experimental results with time-constant inlet flow profiles (). Considering the Novolizer, CFD predictions closely match both the high MT and very low TB deposition values. This resolution of deposited drug mass over two-orders of magnitude compared with the experiments is impressive. CFD predictions of Novolizer MP deposition are low and combined with the MT deposition data. With the Respimat, the CFD model accurately predicts MP, MT, and upper TB DFs. Again, the model accurately resolves DFs of drug mass for a polydisperse pharmaceutical aerosol over multiple orders of magnitude. Simulation of the Respimat inhaler is complicated by the additional momentum of the spray aerosol, as described by Longest and Hindle (Citation2009). The observed agreement between model results and the experiments supports the assumption that deposited drug mass in the NGI preseparator can be assigned a diameter equal to the preseparator cutoff. Furthermore, evaporation of the Respimat droplets can be neglected due to the relatively high mass of water and limited vapor capacity of the air (two-way coupling), resulting in limited evaporation of the pharmaceutical aerosol (Finlay Citation1998). Minor differences between the in vitro results and CFD predictions of deposited drug mass in the upper TB airway are likely due to the surface roughness of the prototyped geometry that was not included in the CFD model.
Considering the transient waveforms used with each inhaler, reports extrathoracic and upper TB deposition of drug mass based on CFD simulations. It is noted that the TB geometry used in these and all subsequent results includes tracheal rings, as visible in the figures. Compared with steady state CFD conditions that excluded the tracheal rings (), deposition trends are largely unchanged for the two inhalers selected. The largest difference in deposition magnitude is observed for the upper TB region and DPI, where DF increases by a factor of approximately 2 with QD inhalation and rings compared with steady state and no rings. This increase is likely due to a majority of the aerosol residing in the upper TB region during the time of peak inspiratory flow rate (PIFR). In contrast, the Respimat aerosol is delivered throughout a 1.5 s period, which better corresponds to delivery during mean flow. Only a small DF increase in the MT region of the Novolizer is likely observed with QD inhalation because a vast majority of the larger aerosol fraction also deposits in this region during steady state. These differences in steady vs. transient deposition in the upper TB airways support the previous finding of Tian et al. (Citation2011b), who indicated the importance of resolving the transient inhalation profile, especially for QD inhalation conditions.
Evaluation of Turbulence Modeling and Effects
Considering turbulence effects first requires an understanding of the flow fields associated with both inhalers. The supplemental information provides an evaluation of both velocity and turbulent viscosity fields for the inhalers. As a result of this analysis, the depth of turbulence penetration in the airways is a function of inhalation flow rate, and for both a characteristic DPI and SMI, laminar conditions are expected at B8 and for more distal airways.
Comparisons of particle deposition profiles for turbulent and laminar flow field assumptions in the SIP model starting with B4 and continuing through B15 are displayed in . As expected in B4–B7, large differences between laminar and turbulent predictions of particle deposition are observed for both inhalers. These differences are more significant for the Novolizer, where flow rate and turbulence levels are highest, compared with the Respimat. Still, laminar to turbulent differences with the Respimat in regions B4–B7 are large. Beginning with B8, very little difference is observed between the laminar and turbulent DFs for both inhalers. It is noted that the SIP model simulates sections B4–B7 and B8–B15 separately. Therefore, differences in DF starting with B4 are carried forward through B7. At B8, the simulation restarts, and zero difference in DF between the laminar and turbulent simulations is possible. Again, flow fields including turbulence properties from the outlet of B7 to the inlet of B8 are applied. As a result of these simulations, it appears that a fully turbulent simulation is required in the SIP model region of B4–B7 and that a laminar flow assumption is adequate in B8–B15. It is noted that the CFD results were not compared with in vitro data beyond B3 in this study. However, it is assumed that model predictions are accurate in the region of B4–B15 based on good agreement with experimental data in the upper airways. Finally, it is observed that turbulence reduces DFs in B4–B7 in some cases. Possible reasons for this reduction are considered in the supplemental information.
Comparison of Monodisperse vs. Polydisperse Aerosol Deposition
A comparison of implementing the MMD of each aerosol as a representative monodisperse aerosol size vs. simulating the polydisperse distributions (divided into size bins) is presented as . Deposition results in the MT and upper TB model for SD inhalation and a polydisperse aerosol from are repeated as panel (b) for comparison. Considering device and upper airway deposition, large differences in the inhaler (65% relative error) and upper TB airways (30% relative error) are observed for the monodisperse approximation (). However, these differences in monodisperse vs. polydisperse deposition are reduced in B4 and demonstrate an overall decreasing trend with more distal bifurcations (). A SIP model of the right lobe (RL) was selected for this comparison. For both upper and SIP airway geometries, it is observed that the monodisperse approximation underestimates deposition (with the exception of B7).
FIG. 5 Deposition fractions of drug mass for the Respimat inhaler based on CFD predictions in the (a and b) MT and upper TB airways and (c and d) mid-TB airways. Panels in the left column (a and c) represent results for a monodisperse aerosol based on MMD, and panels in the right column (b and d) represent simulations using the experimentally measured polydisperse distribution subdivided into 10 size bins.
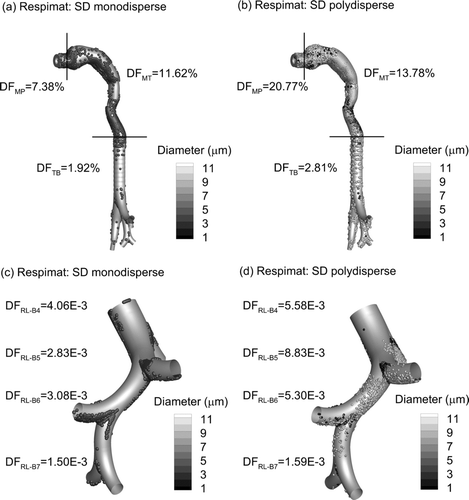
FIG. 6 Deposition fraction of drug mass for both inhalers implementing monodisperse vs. polydisperse approximations with the monodisperse particle size determined from the (a) regional inlet MMD or (b) regional inlet equivalent MMD calculated as 1.25 × MMD. In each case, the MMD is calculated from the polydisperse aerosol at the inlet to B4 and B7. The equivalent MMD approximation closely matches the polydisperse simulations and provides a five-fold increase in computational efficiency.
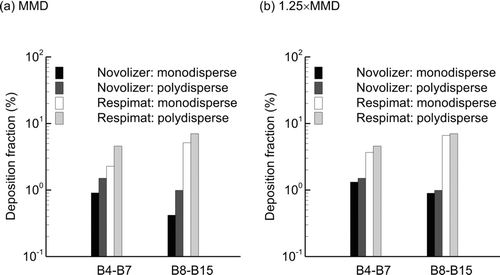
While it appears that differences in monodisperse vs. polydisperse approximations are reduced for lower TB airways, these differences can accumulate and produce large effects on regional DFs. illustrates regional DFs in the SIP models for B4–B7 and B8–B15 for monodisperse vs. polydisperse aerosol assumptions. For both inhalers, monodisperse estimates are approximately 50% (relative difference) below the more accurate polydisperse approximations. When considered closely, this difference is not surprising given that a larger fraction of the polydisperse particles above the median will deposit by impaction and sedimentation compared with the fraction below the median. As a result, an equivalent monodisperse diameter to approximate polydisperse deposition should be larger than the median. Considering that different polydisperse aerosol size distributions with bimodal shapes were found for the two inhalers, an analytical estimate of an equivalent monodisperse correction factor was not effective. Instead, a range of factors to modify the MMD and produce equivalent polydisperse deposition values was considered. It was determined that 1.25 × MMD provided monodisperse estimates of DF within approximately 10% compared with the more accurate polydisperse size approximation (). The one exception was in B4–B7 with the Respimat, where the relative difference was less than 20%. However, use of the 1.25 factor is observed to significantly improve the predictions of the monodisperse aerosol at considerable computational savings.
Comparison of Deposition Among Lobes
Deposition fractions with the Respimat and Novolizer inhalers using the correct inhalation waveforms in the upper TB airways (B1–B3) and individual lobes are displayed in . As displayed in , one SIP per lung lobe is considered. The lobes of the lungs are denoted as LU, LL, RU, RM, and RL. As expected from previous studies, large differences are observed in the DFs among the lobes. These differences arise from variations in particle inlet fractions, airflow ventilation, and orientation of each lobe with respect to gravity. Moreover, small DFs are observed in the TB airways as a whole, with values of 4.0% and 13.1% for the Novolizer and Respimat inhalers, respectively. It would be advantageous if a single lung lobe could be used to characterize deposition through the TB airways. and d present each lobar DF multiplied by a factor of 5 (to represent all five lung lobes) and compared with the total TB lobar (TBB4–B15) DFs. For both inhalers, the characteristic lower left lobe value, denoted 5LL in the figure, provided a good representation of the total TB lobar DF with relative errors of 12% and below. This relative error appears small compared with the associated fivefold decrease in computational effort associated with using one lung lobe to represent total lobar deposition.
FIG. 7 Deposition fractions for individual lung lobes and the TB region for the (a) Novolizer and (b) Respimat inhalers. To determine if lobar values were characteristic of total TB deposition in the range of B4–B15, deposition values were multiplied by a factor of 5 and compared with TBB4–B15 for the (c) Novolizer and (d) Respimat inhalers.
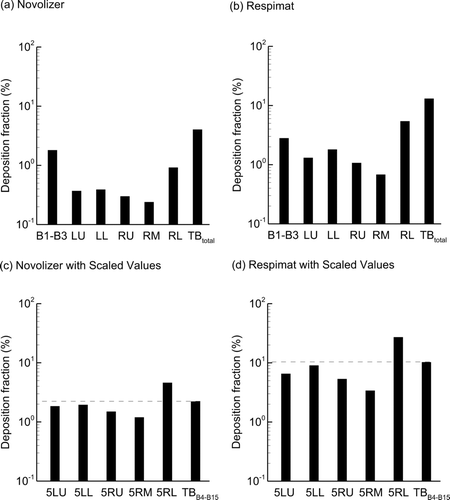
Performance Comparison of Inhalers
presents a performance comparison in terms of DF within multiple airway regions for the two inhalers considered. Results are based on an equivalent MMD assumption in the SIP model (1.25 × MMD) and simulating all five lung lobes. Drug delivery from the Respimat is approximately 1.5 times higher compared to the Novolizer in the region of the trachea to B3. Effectiveness of the Respimat increases further in the lower TB airways with DFs that are an order of magnitude higher in B8–B15 compared with the Novolizer. Surprisingly, the Novolizer delivers very little drug to the lower TB airways (DF in B8–B15 is <1%). Deposition fractions reported in are also reported in quantitatively. It is noted that the MT DF for the Respimat inhaler includes both the mouthpiece and MT deposition in both and . Penetration fractions (PFs) are also provided in , which represent the fraction of initial drug mass that exits each section of the model. For example, the Respimat MT PF indicates that 0.655 of the initial dose (out of the spray nozzle) exits the MT and enters the trachea. Based on the reported PFs (), the Respimat inhaler is observed to deliver over 1.5 times more drug to the alveolar airways compared with the Novolizer, which is beneficial for medications intended to be absorbed into the blood for systemic delivery. Further studies are needed to determine if this delivered alveolar fraction actually deposits, or if a fraction is exhaled back into the TB region.
DISCUSSION
Previous studies by Tian et al. (Citation2011a,b) have introduced the SIP modeling approach and applied it to evaluate conventional and next-generation forms of pharmaceutical aerosol delivery to the entire conducting airways. In this study, the SIP approach was further explored for a typical DPI and a state-of-the-art SMI with comparisons to in vitro results of upper airway deposition. Major challenges that arise when simulating aerosol deposition throughout the conducting airways were addressed including the selection of an efficient and accurate turbulence model, the simulation of polydisperse vs. monodisperse aerosols, and the consideration of differences in deposition among individual lung lobes. The results of this study indicate appropriate model selections for the SIP and other whole-lung approaches to resolve MT and TB aerosol deposition using CFD simulations. Findings related to the selection of an appropriate turbulence model, use of an equivalent MMD (MMDeq or MMADeq), and simulation of one characteristic lobe are discussed in the supplemental information.
Considering the previous study of Tian et al. (Citation2011b) along with the current results, a highly effective method for providing high-resolution CFD solutions of pharmaceutical aerosol delivery throughout the airways is emerging. Results of previous studies and the current study indicate that the LRN k–ω transport model with near-wall corrections is appropriate for simulating respiratory aerosol deposition. For capturing transport and deposition from the inhaler through the MT and into the upper TB airways, transient simulations should be employed that resolve the inhalation waveform (especially for QD conditions), the flow field should be simulated as turbulent for most practical inhalation flow rates, and polydisperse size distributions should be considered. The SIP modeling approach is then used to resolve deposition within the lung lobes using randomly generated individual paths. Outflow conditions at the exit of each B3 are used as the inlet conditions of each SIP model. Tian et al. (Citation2011b) previously indicated that one SIP model per lobe could adequately resolve deposition for symmetric bifurcations with out-of-plane rotation. Results of the current study indicate that an equivalent monodisperse aerosol can be used in the SIP geometries with diameters calculated as 1.25 × MMD for each B3 outlet distribution. Turbulence modeling should be included in the B4–B7 section of each SIP geometry and laminar flow can be assessed in B8 and more distal bifurcations. Results of the current study also indicate that deposition in the LL lobe can be used to characterize TB deposition in all lobes (DF5LL) with the introduction of a 12% relative error. Combining the over five times increase in computational efficiency associated with the use of monodisperse aerosols with the five times increase associated with simulating a single lobe results in a factor of 25 decrease in computational effort. This improvement is in addition to the 3 × 105 efficiency improvement reported by Tian et al. (Citation2011b) for use of the SIP model vs. resolving all TB pathways. Due to the complexity of the system, the associated relative errors cannot be combined analytically. However, all relative errors associated with the dramatic increase in computational efficiency of the SIP approximation are below 12%.
FIG. 8 Performance comparison of the Novolizer and Respimat inhalers in terms of DF within airway regions based on the MMDeq assumption in the SIP models and calculations for all five lung lobes.
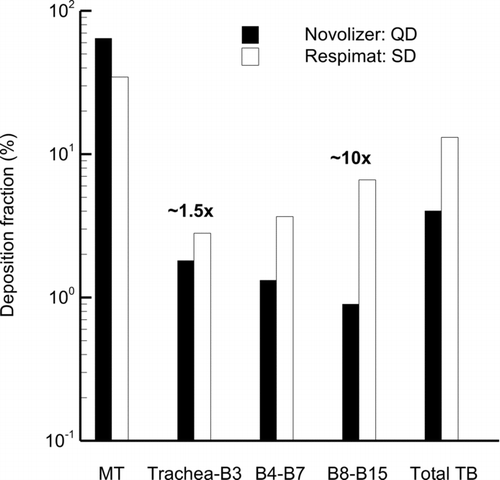
Limitations of the current study include both approximations of the aerosol and physical features that are not currently included in the model. Considering the discrete phase, it is expected that aerosol size change will affect deposition to some degree. However, size change was excluded in the current study to allow for better evaluation of the variables of interest. Use of the preseparator cutoff diameter under predicts the size of the larger particles present in the DPI aerosol, which could affect the site of deposition in the MT region. A primary physical limitation of the current SIP approach is the use of symmetric bifurcations starting at B4. Asymmetry in bifurcations is known to occur (Phalen et al. Citation1978) and will likely affect deposition. Wall motion in the lower TB airways, as considered in previous studies of stress and strain (Koombua et al. Citation2009; Xia et al. Citation2011), may also increase deposition. In addition, a fixed outflow proportion among the lobar branches was used for both steady state and transient inhalations. This outflow distribution likely changes over time. Finally, the inclusion of an alveolar section of the model is required to resolve deposition over an entire respiratory cycle (Sznitman et al. Citation2009). While these assumptions will likely have an effect on the overall predicted DFs, they are expected to have a limited impact on the comparisons made in this study and the associated findings.
TABLE 1 Deposition fractions (DFs) and penetration fractions (PFs) at the outlet of individual airway regions with correct inhalation (Novolizer with QD and Respimat with SD)
Considering the lobar deposition results, maximum deposition in the lower lobes is consistent with previous findings (Subramaniam et al. Citation2003). The ratio of right to left deposition of particles in the current study was approximately 1.2. For a patient-specific TB model, Lambert et al. (Citation2011) previously reported a right to left deposition ratio for 2.5 μm particles of approximately one through the seventh respiratory generation. The distribution of right to left particle ventilation is affected by the shape of the trachea, laryngeal jet, and position of the main carina relative to the tracheal flow. Including curvature of the trachea as well as more careful consideration of the variation in position of the main carina will influence the right vs. left ventilation of particles in the model, and should be considered in future studies.
Several limitations of the current findings should be considered in future applications of this work. First, the developed MMDeq factor of 1.25 is based on the evaluation of one DPI and one SMI with very different polydisperse aerosol distribution profiles. Considering different pharmaceutical inhalers and aerosols with different GSDs may result in modifications to the equivalence factor. However, it is impressive that the MMDeq factor of 1.25 was consistent for very different inhaler types. Provided that changes in lung geometry do not alter deposition mechanisms, this factor is expected to be applicable to patient-specific airway models of adults and possibly children. It is also noted that the MMDeq developed in this study is for micrometer aerosols depositing primarily by impaction and sedimentation and that this relationship is not valid for the deposition of nanoparticles by diffusion. Considering deposition in individual lobes, it is noted that the finding of characteristic deposition in the LL lobe may change for patient-specific geometries, different inhalation maneuvers, and disease states. However, results of characteristic deposition in the LL lobe were consistent in this study for two different pharmaceutical aerosols and two types of inhalation waveforms.
In conclusion, this study has further developed the SIP modeling approach to allow for CFD calculations of pharmaceutical and ambient aerosols delivered throughout the TB airways. The LRN k–ω turbulence model with near-wall corrections was found to be effective at simulating local and regional deposition of complex pharmaceutical aerosols from two different inhaler platforms. Implementation of a new equivalent monodisperse diameter and evaluation of deposition in a single characteristic lung lobe reduced computational effort required for the simulations by a factor of approximately 25 with increases in relative errors below 12%. Based on the current SIP model analysis, results indicated that a state-of-the-art SMI improved TB delivery efficiency of drug by a factor of 1.5 in the upper TB region and by over an order of magnitude in the lower TB airways compared with a commonly used high quality DPI. Moreover, the SIP model predicted that the Respimat inhaler delivered a higher dose of drug to the alveolar airways compared with the DPI. Best practices for evaluating conducting airway deposition based on this and previous studies using the SIP model for both pharmaceutical and ambient aerosols were established. Future studies are needed to evaluate the effects of aerosol size change on the performance of DPI and SMI inhalers and to evaluate physiological features such as wall motion and asymmetry of lower TB airways on the deposition of pharmaceutical aerosols using complete conducting airway models like the SIP approach.
ABBREVIATIONS
AS | = |
albuterol sulfate |
B# | = |
bifurcation number (1 is trachea plus main bronchi) |
CFD | = |
computational fluid dynamics |
CT | = |
computed tomography |
DE | = |
deposition efficiency |
DF | = |
deposition fraction |
DNS | = |
direct numerical simulation |
DPI | = |
dry powder inhaler |
ECG | = |
enhanced condensational growth |
Exp | = |
experiment |
FR | = |
fraction remaining |
FRC | = |
functional residual capacity |
GSD | = |
geometric standard deviation |
LES | = |
large eddy simulation |
LRN | = |
low Reynolds number |
MDI | = |
metered dose inhaler |
MMAD | = |
mass median aerodynamic diameter |
MMD | = |
mass median diameter |
MMDeq | = |
mass median diameter equivalent |
MT | = |
mouth–throat |
NGI | = |
next generation impactor |
PF | = |
penetration fraction |
PRB | = |
physiologically realistic bifurcation |
QD | = |
quick and deep inhalation waveform |
SD | = |
slow and deep inhalation waveform |
SIP | = |
stochastic individual path |
SMI | = |
soft-mist inhaler |
Ta–mean | = |
time of mean accelerating flow |
TB | = |
tracheobronchial |
uast_a_708799_sup_27285848.zip
Download Zip (421.8 KB)Acknowledgments
This study was supported in part by a contract from the U.S. Food and Drug Administration (Number HHSF223201000093C). The content is solely the responsibility of the authors and does not necessarily represent the official views of the U.S. Food and Drug Administration.
[Supplementary materials are available for this article. Go to the publisher's online edition of Aerosol Science and Technology to view the free supplementary files.]
REFERENCES
- Asgharian , B. and Price , O. T. 2006 . Airflow Distribution in the Human Lung and Its Influence on Particle Deposition . Inhal. Toxicol. , 18 : 795 – 801 .
- Balashazy , I. , Hofmann , W. and Heistracher , T. 1999 . Computation of Local Enhancement Factors for the Quantification of Particle Deposition Patterns in Airway Bifurcations . J. Aerosol Sci. , 30 : 185 – 203 .
- Byron , P. R. , Delvadia , R. R. , Longest , P. W. and Hindle , M. 2010 . Stepping into the Trachea with Realistic Physical Models: Uncertainties in Regional Drug Deposition from Powder Inhalers . Resp. Drug Deliv. 2010 , 1 : 215 – 224 .
- Cheng , K. H. , Cheng , Y. S. , Yeh , H. C. and Swift , D. L. 1997 . Measurements of Airway Dimensions and Calculation of Mass Transfer Characteristics of the Human Oral Passage . J. Biomech. Eng. , 119 : 476 – 482 .
- Dalby , R. , Spallek , M. and Voshaar , T. 2004 . A Review of the Development of Respimat Soft Mist Inhaler . Int. J. Pharm. , 283 : 1 – 9 .
- Delvadia , R. R. , Byron , P. R. , Longest , P. W. and Hindle , M. 2010 . In Vitro Prediction of Regional Drug Deposition from Dry Powder Inhalers . Resp. Drug Deliv. 2010 , : 907 – 911 .
- Delvadia , R. , Longest , P. W. and Byron , P. R. 2012 . In Vitro Tests for Aerosol Deposition. I. Scaling a Physical Model of the Upper Airways to Predict Drug Deposition Variation in Normal Humans . J. Aerosol Med. Pulm. Drug Deliv. , 25 ( 1 ) : 32 – 40 .
- Fenton , C. , Keating , G. M. and Plosker , G. L. 2003 . Novolizer: A Multidose Dry Powder Inhaler . Drugs , 63 ( 22 ) : 2437 – 2445 .
- Finlay , W. H. 1998 . Estimating the Type of Hygroscopic Behavior Exhibited by Aqueous Droplets . J. Aerosol Med. , 11 ( 4 ) : 221 – 229 .
- Ghalichi , F. , Deng , X. , Champlain , A. D. , Douville , Y. , King , M. and Guidoin , R. 1998 . Low Reynolds Number Turbulence Modeling of Blood Flow in Arterial Stenoses . Biorheology , 35 ( 4&5 ) : 281 – 294 .
- Gosman , A. D. and Ioannides , E. 1981 . Aspects of Computer Simulation of Liquid-Fueled Combustors . J. Energy , 7 : 482 – 490 .
- Heistracher , T. and Hofmann , W. 1995 . Physiologically Realistic Models of Bronchial Airway Bifurcations . J. Aerosol Sci. , 26 ( 3 ) : 497 – 509 .
- Hochrainer , D. , Holz , H. , Kreher , C. , Scaffidi , L. , Spallek , M. and Wachtel , H. 2005 . Comparison of the Aerosol Velocity and Spray Duration of Respimat Soft Mist Inhaler and Pressurized Metered Dose Inhalers . J. Aerosol Med. , 18 ( 3 ) : 273 – 282 .
- Horsfield , K. , Dart , G. , Olson , D. E. , Filley , G. F. and Cumming , G. 1971 . Models of the Human Bronchial Tree . J. Appl. Phys. , 31 : 207 – 217 .
- ICRP . 1994 . Human Respiratory Tract Model for Radiological Protection , New York : Elsevier Science Ltd. .
- Jayaraju , S. T. , Brouns , M. , Lacor , C. , Belkassem , B. and Verbanck , S. 2008 . Large Eddy and Detached Eddy Simulations of Fluid Flow and Particle Deposition in a Human Mouth-Throat . Aerosol Sci. , 39 : 862 – 875 .
- Jin , H. H. , Fan , J. R. , Zeng , M. J. and Cen , K. F. 2007 . Large Eddy Simulation of Inhaled Particle Deposition Within the Human Upper Respiratory Tract . Aerosol Sci. , 38 : 257 – 268 .
- Kim , J. , Moin , P. and Moser , R. D. 1987 . Turbulence Statistics in Fully Developed Channel Flow at Low Reynolds Number . J. Fluid Mech. , 177 : 133 – 166 .
- Kleinstreuer , C. and Zhang , Z. 2009 . An Adjustable Triple-Bifurcation Unit Model for Air-Particle Flow Simulations in Human Tracheobronchial Airways . J. Biomech. Eng. , 131 : 021007
- Koombua , K. , Pidaparti , R. M. , Longest , P. W. and Ward , K. R. 2009 . Tissue Flexibility Effects on Airway Pressure and Stress During Mechanical Ventilation . Mol. Cell. Biomech. , 6 ( 4 ) : 203 – 216 .
- Lambert , A. R. , O’Shaughnessy , P. T. , Tawhai , M. H. , Hoffman , E. A. and Lin , C.-L. 2011 . Regional Deposition of Particles in an Image-Based Airway Model: Large-Eddy Simulation and Left-Right Lung Ventilation Asymmetry . Aerosol Sci. Technol. , 45 : 11 – 25 .
- Lin , C.-L. , Tawhai , M. H. , McLennan , G. and Hoffman , E. A. 2007 . Characteristics of the Turbulent Laryngeal Jet and Its Effect on Airflow in the Human Intra-Thoracic Airways . Resp. Phys. Neurobiol. , 157 : 295 – 309 .
- Longest , P. W. and Hindle , M. 2009 . Evaluation of the Respimat Soft Mist Inhaler Using a Concurrent CFD and In Vitro Approach . J. Aerosol Med. Pulm. Drug Deliv. , 22 ( 2 ) : 99 – 112 .
- Longest , P. W. , Hindle , M. and Das Choudhuri , S. 2009 . Effects of Generation Time on Spray Aerosol Transport and Deposition in Models of the Mouth-Throat Geometry . J. Aerosol Med. Pulm. Drug Deliv., , 22 ( 3 ) : 67 – 84 .
- Longest , P. W. , Hindle , M. , Das Choudhuri , S. and Byron , P. R. 2007 . Numerical Simulations of Capillary Aerosol Generation: CFD Model Development and Comparisons with Experimental Data . Aerosol Sci. Technol. , 41 : 952 – 973 .
- Longest , P. W. , Hindle , M. , Das Choudhuri , S. and Xi , J. 2008 . Comparison of Ambient and Spray Aerosol Deposition in a Standard Induction Port and More Realistic Mouth-Throat Geometry . J. Aerosol Sci. , 39 : 572 – 591 .
- Longest , P. W. and Holbrook , L. T. 2012 . In Silico Models of Aerosol Delivery to the Respiratory Tract – Development and Applications . Adv. Drug Deliv. Reviews , 64 : 296 – 311 .
- Longest , P. W. , Kleinstreuer , C. and Buchanan , J. R. 2004 . Efficient Computation of Micro-Particle Dynamics Including Wall Effects . Computers and Fluids , 33 ( 4 ) : 577 – 601 .
- Longest , P. W. and Vinchurkar , S. 2007 . Effects of Mesh Style and Grid Convergence on Particle Deposition in Bifurcating Airway Models with Comparisons to Experimental Data . Med. Eng. Phys. , 29 ( 3 ) : 350 – 366 .
- Longest , P. W. , Vinchurkar , S. and Martonen , T. B. 2006 . Transport and Deposition of Respiratory Aerosols in Models of Childhood Asthma . J. Aerosol Sci. , 37 : 1234 – 1257 .
- Longest , P. W. and Xi , J. 2007 . Effectiveness of Direct Lagrangian Tracking Models for Simulating Nanoparticle Deposition in the Upper Airways . Aerosol Sci. Technol. , 41 : 380 – 397 .
- Longest , P. W. and Xi , J. 2008 . Condensational Growth May Contribute to the Enhanced Deposition of Cigarette Smoke Particles in the Upper Respiratory Tract . Aerosol Sci. Technol. , 42 : 579 – 602 .
- Loth , E. 2000 . Numerical Approaches for Motion of Dispersed Particles Droplets and Bubbles . Prog. Energy Comb. Sci. , 26 : 161 – 223 .
- Ma , B. and Lutchen , K. R. 2009 . CFD Simulations of Aerosol Deposition in an Anatomically Based Human Large-Medium airway Model . Ann. Biomed. Eng. , 37 ( 2 ) : 271 – 285 .
- Martonen , T. B. and Katz , I. 1993 . Deposition Patterns of Polydisperse Aerosols Within Human Lungs . J. Aerosol Med.—Depos. Clear. Effects Lung , 6 ( 4 ) : 251 – 274 .
- Martonen , T. B. , Yang , Y. and Xue , Z. Q. 1994 . Influences of Cartilaginous Rings on Tracheobronchial Fluid Dynamics . Inhal. Toxicol. , 6 ( 3 ) : 185 – 198 .
- Matida , E. A. , DeHaan , W. H. , Finlay , W. H. and Lange , C. F. 2003 . Simulation of Particle Deposition in an Idealized Mouth with Different Small Diameter Inlets . Aerosol Sci. Technol. , 37 : 924 – 932 .
- Matida , E. A. , Finlay , W. H. , Breuer , M. and Lange , C. F. 2006 . Improving Prediction of Aerosol Deposition in an Idealized Mouth Using Large-Eddy Simulation . J. Aerosol Med. , 19 ( 3 ) : 290 – 300 .
- Matida , E. A. , Finlay , W. H. and Grgic , L. B. 2004 . Improved Numerical Simulation of Aerosol Deposition in an Idealized Mouth-Throat . J. Aerosol Sci. , 35 : 1 – 19 .
- Matida , E. A. , Nishino , K. and Torii , K. 2000 . Statistical Simulation of Particle Deposition on the Wall from Turbulent Dispersed Pipe Flow . Int. J. Heat Fluid Flow , 21 : 389 – 402 .
- Newman , S. P. and Busse , W. W. 2002 . Evolution of Dry Powder Inhaler Design, Formulation, and Performance . Resp. Med. , 96 : 293 – 304 .
- Newman , S. P. , Pitcairn , G. R. , Hirst , P. H. , Bacon , R. E. , O’Keefe , E. Reiners , M. 2000 . Scintigraphic Comparison of Budesonide Deposition from Two Dry Powder Inhalers . Eur. Resp. J. , 16 ( 1 ) : 178 – 183 .
- Olsson , B. , Berg , E. and Svensson , M. 2010 . Comparing Aerosol Size Distributions That Penetrate Mouth-Throat Models under Realistic Inhalation Conditions . Resp. Drug Deliv. , : 225 – 234 . 2010
- Phalen , R. F. , Yeh , H. C. , Schum , G. M. and Raabe , O. G. 1978 . Application of an Idealized Model to Morphometry of the Mammalian Tracheobronchial Tree . Anat. Rec. , 190 : 167 – 176 .
- Russo , J. , Robinson , R. and Oldham , M. J. 2008 . Effects of Cartilage Rings on Airflow and Particle Deposition in the Trachea and Main Bronchi . Med. Eng. Phys. , 30 : 581 – 589 .
- Shi , H. and Kleinstreuer , C. 2007 . Simulation and Analysis of High-Speed Droplet Spray Dynamics . J. Fluids Eng. , 129 : 621 – 633 .
- Smith , I. J. , Bell , J. , Bowman , N. , Everard , M. , Stein , S. and Weers , J. G. 2010 . Inhaler Devices: What Remains to be Done? . J. Aerosol Med. Pulm. Drug Deliv. , 23 : S25 – S37 .
- Subramaniam , R. P. , Asgharian , B. , Freijer , J. I. , Miller , F. J. and Anjilvel , S. 2003 . Analysis of Lobar Differences in Particle Deposition in the Human Lung . Inhal. Toxicol. , 15 ( 1 ) : 1 – 21 .
- Sznitman , J. , Heimshch , T. , Wildhaber , J. H. , Tsuda , A. and Rosgen , T. 2009 . Respiratory Flow Phenomena and Gravitational Deposition in a Three-dimensional Space-filling Model of the Pulmonary Acinar Tree . J. Biomech. Eng. , 131 031010–1–15
- Tian , G. , Longest , P. W. , Su , G. and Hindle , M. 2011a . Characterization of Respiratory Drug Delivery with Enhanced Condensational Growth (ECG) using an Individual Path Model of the Entire Tracheobronchial Airways . Ann. Biomed. Eng. , 39 ( 3 ) : 1136 – 1153 .
- Tian , G. , Longest , P. W. , Su , G. , Walenga , R. L. and Hindle , M. 2011b . Development of a Stochastic Individual Path (SIP) Model for Predicting the Tracheobronchial Deposition of Pharmaceutical Aerosols: Effects of Transient Inhalation and Sampling the Airways . J. Aerosol Sci. , 42 : 781 – 799 .
- Tian , L. and Ahmadi , G. 2007 . Particle Deposition in Turbulent Duct Flows-Comparisons of Different Model Predictions . J. Aerosol Sci. , 38 : 377 – 397 .
- Vinchurkar , S. and Longest , P. W. 2008 . Evaluation of Hexahedral, Prismatic and Hybrid Mesh Styles for Simulating Respiratory Aerosol Dynamics . Computers and Fluids , 37 : 317 – 331 .
- Wang , Y. and James , P. W. 1999 . On the Effect of Anisotropy on the Turbulent Dispersion and Deposition of Small Particles . Int. J. Multiphase Flow , 22 : 551 – 558 .
- Wilcox , D. C. 1998 . Turbulence Modeling for CFD , 2nd ed. , California : DCW Industries, Inc. .
- Xi , J. and Longest , P. W. 2007 . Transport and Deposition of Micro-Aerosols in Realistic and Simplified Models of the Oral Airway . Ann. Biomed. Eng. , 35 ( 4 ) : 560 – 581 .
- Xi , J. , Longest , P. W. and Martonen , T. B. 2008 . Effects of the Laryngeal Jet on Nano- and Microparticle Transport and Deposition in an Approximate Model of the Upper Tracheobronchial Airways . J. Appl. Phys. , 104 : 1761 – 1777 .
- Xia , G. H. , Tawhai , M. H. , Hoffman , E. A. and Lin , C. L. 2011 . Airway Wall Stiffening Increases Peak Wall Shear Stress: A Fluid-Structure Interaction Study in Rigid and Compliant Airways . Ann. Biomed. Eng. , 38 ( 5 ) : 1836 – 1853 .
- Yeh , H. C. and Schum , G. M. 1980 . Models of Human Lung Airways and Their Application to Inhaled Particle Deposition . Bull. Math. Biol. , 42 : 461 – 480 .
- Yin , Y. , Choi , J. , Hoffman , E. A. , Tawhai , M. H. and Lin , C.-L. 2010 . Simulation of Pulmonary Air Flow with a Subject-Specific Boundary Condition . J. Biomech. , 43 : 2159 – 2163 .
- Zhang , Y. and Finlay , W. H. 2005 . Measurement of the Effect of Cartilaginous Rings on Particle Deposition in a Proximal Lung Bifurcation Model . Aerosol Sci. Technol. , 39 : 394 – 399 .
- Zhang , Y. , Gilbertson , K. and Finlay , W. H. 2007 . In vivo-In vitro Comparison of Deposition in Three Mouth-Throat Models with Qvar and Turbuhaler Inhalers . J. Aerosol Med. , 20 ( 3 ) : 227 – 235 .
- Zhang , Z. and Kleinstreuer , C. 2011 . Computational Analysis of Airflow and Nanoparticle Deposition in a Combined Nasal-Oral-Tracheobronchial Airway Model . J. Aerosol Sci. , 42 : 174 – 194 .
- Zhang , Z. , Kleinstreuer , C. and Kim , C. S. 2008 . Airflow and Nanoparticle Deposition in a 16-Generation Tracheobronchial Airway Model. . Ann. Biomed. Eng. , 36 ( 12 ) : 2095 – 2110 .
- Zhang , Z. , Kleinstreuer , C. and Kim , C. S. 2009 . Comparison of Analytical and CFD Models with Regard to Micron Particle Deposition in a Human 16-Generation Tracheobronchial Airway Model . J. Aerosol Sci. , 40 : 16 – 28 .