Abstract
Human foot motions such as walking and foot tapping resuspend the particulate matter on the floor and redistribute it, increasing the particle concentration in air and affecting the indoor air quality. The objective of this article is to experimentally investigate the mechanism of particle resuspension and redistribution due to human foot motion from focusing on aerodynamic effect. In particular, we have examined generation and deformation of a vortex produced by foot motion and how it is affected by the shape of the shoe sole. The experimental methods used were particle visualizations and particle image velocimetry (PIV) measurements in air, supplemented by dye flow visualization in water. The flow visualizations with human foot tapping and stomping were performed to elucidate the particle resuspension in real situations. In the laboratory experiment, the foot was modeled as either an elongated plate or a prosthetic foot wearing a slipper, moving normal to the floor downward or upward. The particle resuspension and redistribution were associated with the wall jet between the foot and floor and the vortex dynamics. With the elongated plate foot model and the slipper, three-dimensional vortex structure strongly affected the particle redistribution and its direction.
Copyright 2013 American Association for Aerosol Research
1. INTRODUCTION
Any amount of particulate matter suspended in the air affects the air quality. Walking and other human activities in the room are known to cause elevated concentrations of dust suspended in indoor air. The particulate matter is first detached and resuspended from the floor, and then entrained into the human thermal plume, where it is inhaled. Ferro et al. (Citation2004) obtained the particle size and mass of resuspended particles from human activities. Aerosol concentrations and particle size distributions were measured both indoors and outdoors by Thatcher and Layton (Citation1995), who studied the resuspension of the particles, the mass loading of dust on floor surfaces and the deposition velocity of the particles. In spite of the global effect that walking has on particle concentrations, the detailed mechanism of how human motion causes the resuspension of particles has not been defined.
Predicting the particle resuspension poses challenges because the particles are strongly attracted to the floor via various forces (i.e., adhesion, electrostatic, Van Der Waals) (Soltani and Ahmadi 1994) and can be detached by either ballistic or aerodynamic mechanisms (Eames and Dalziel 2000), or a combination of both. The ballistic mechanism occurs when colliding particles break the cohesive bond which exists between particles. The aerodynamic mechanism takes place when the particles are resuspended purely by flow disturbances generated by the human body such as foot motion. Addressing a fundamental mechanism, the dust resuspension caused by a sphere impacting the wall was investigated by Eames and Dalziel (2000). Particle resuspension due to a vertically traveling disk was studied experimentally by Kubota et al. (Citation2009). Analytical and numerical studies of the aerodynamic resuspension mechanism of particles attached to the wall beneath a falling disk were conducted by Khalifa and Elhadidi (2007). The vortex dynamics around a sphere impacting the wall was studied by Leweke et al. (Citation2004). The unsteady vortex structure and its interaction with the accelerating and decelerating disk were experimentally and numerically investigated in Higuchi et al. (1996b).
This article examines the particle suspension due to human foot motion (foot tapping with a pivoting action and stomping involving only the vertical motion) and a model foot undergoing a simulated vertical foot motion. As a follow up on a previous study using disk geometry the three-dimensional geometric effect as well as human foot motion is examined. As was the case in the previous study, the laboratory test focuses on the aerodynamic mechanism of particle resuspension assuming no floor vibrations. The wall jet and the vortex dynamics caused by the idealized foot motion are measured by Particle Image Velocimetry (PIV). Also, this article addresses the subsequent redistribution of the particles by the aerodynamic mechanism.
The foot movement during the human walking has been recorded in this experiment as described in the next section. Modeling the human walking motion and its associated foot kinetics, however, with a vertical, a horizontal and a rotational component (Winter, Citation2005), is extremely complex. In this laboratory experiment, an idealized foot motion is used. As a first approximation, the stepping motion is modeled as normal motion to the floor, and both the downward and upward portion of the foot stomping are examined. In these experiments, the motion is stopped just before touching the floor to focus on the aerodynamic resuspension mechanism, ensuring negligible floor vibration. The idealized foot is modeled as an elongated plate with the equivalent area to a US size 8 human shoe and a foot model wearing an indoor slipper.
2. PARTICLE VISUALIZATION OF HUMAN FOOT MOVEMENT
2.1. Experimental Procedure
The measurements of vertical foot position during the walking were carried out. The foot position was captured with Photron high-speed video camera. The results of time-histories of heel, mid-section, and toe are in good agreement with those reported by Winter (Citation2005) and the difference may stem from foot size and minor difference in walking patterns. As noted in the introduction, in the present study, the essential components of the foot motion pertinent to the dust suspension has been represented by foot tapping and stomping actions. Therefore, particle flow visualizations with an actual foot tapping and stomping were first conducted. These two modes focus on the rotation and vertical motions of the foot, and are regarded as major elements in the walking process. The human subject wore an indoor slipper with a smooth sole during tapping and stomping motions. The foot position and velocity were recorded with a Photron high-speed video camera at 125 frames per second (fps) and analyzed on the computer. For particle visualization, 3M Ceramic Microspheres G-200 with density of 2.5 g/cm3 and a mean diameter of 8 μm were uniformly distributed underneath the foot prior to the foot motion by using a sifter. The typical initial density of seeding was 5.6 mg/cm2. The typical density was measured with the gathered particle from the certain area (10 × 10 mm2). The particles were gathered from beside the foot, since the initial seeding pattern was considered. The floor surface was cleaned up in every trial to keep the experimental condition. The flow field was illuminated in its cross section with a thin laser sheet from an Argon ion laser beam. The particle resuspension from the smooth hard surface due to the human foot motion was recorded as the foot either tapped or stomped.
2.2. Results of Particle Resuspension by Human Foot Tapping and Stomping
The effect of the foot tapping was studied first. The foot tapping motion consisted of the foot pivoting around the heel and then touching the floor. The toe is lifted up at t = 0 s and contacts the floor shortly after t = 0.5 s. The angular velocity was approximately 5 rad/s when the toe contacted the floor. The sequence of particle flow visualization with the foot tapping motion is shown in . The toe is facing the reader. The foot downstroke motion is completed at t = 0 s in shows the results during the upstroke motion. The particles that were seeded beneath the foot were expelled from the floor and entrained into the vortex at t = 0 s. Later at t = 0.1 s in , the resuspended particles were driven further out to the side. During the upward motion at t = 0.2 s in , the particles were also resuspended in the wake that was behind and under the foot. The visualization in side cross sectional view was also carried out. The particles resuspension was observed at a longitudinal cross sectional view in the toe region at the completion of the downward tapping motion. Less amount of particles were ejected forward than toward the sides of the foot.
The foot stomping motion and resulting particle resuspension were next examined with the high-speed camera. The foot stomping motion was a vertical motion; with the sole of the slipper kept parallel to the floor during the motion. The time history of the foot position was measured, and vertical trajectory of the foot resembles that of the walking (measured in house). During the downstroke near the wall, the foot is near parallel and the overall foot position and the velocity near the floor at the end of the downstroke were also measured. The overall movement of the foot and its velocity time history were also recorded and incorporated into the laboratory experiment as will be shown. The results of the particle flow visualization of the stomping motion are shown in . These results were taken 0.2 s after the foot completed the downstroke motion. As before, particles were initially seeded beneath the foot. The subject wore an indoor slipper. In , the toe is facing the reader and the laser light sheet illuminates the mid section of the lateral particle flow motion. shows the resuspension of particles from the toe in the longitudinal cross section. shows the resuspension of particles from the heel in the longitudinal cross section. The particles were expelled from the floor. The particles were also resuspended from the heel since the result of particle flow visualization. These are the evidence that the particles are expelled in both areas of the foot. Three-dimensional particle resuspension patterns were examined in more detail with the model foot and will be described later. The resuspended particles became entrained into the vortex structure around the foot. The size of the vortex core also varied around the foot. The mid-section of the foot in produced a larger vortex than in front of both the toe in and the heel in , indicating the effect of the geometry of the sole on the resuspension and redistribution of particles. Resuspension of particles from the floor due to foot tapping and stomping motions was clearly demonstrated. To better understand the process of particle resuspension and redistribution in a three-dimensional geometry, laboratory experiments were carried out using an idealized model foot.
3. LABORATORY EXPERIMENT ON SIMULATED FOOT MOTION
3.1. Set Up
The particle resuspension study of the actual foot motion was followed up in the laboratory setting with an idealized foot to maximize the measurement accuracy and repeatability, minimize motion variability and to examine the detailed mechanism of the particle resuspension process. The focus of the present study was on the three-dimensional effects due to geometry that are more representative of shoes than disk geometry. Two different geometries were used: (1) elongated plate and (2) indoor slipper. Both had a smooth bottom surface. The geometry of the elongated plate was a rectangle with two half circles at both ends: the overall dimensions were W = 71.7 mm in width and L = 269.8 mm in length. The total area corresponds to US shoe size 8 and the effective diameter is D = 152.4 mm. The idealized foot motions were driven vertically by a linear servomotor. The motion was controlled by specifying the maximum velocity, acceleration, deceleration, and stroke. Both the particle visualization and the flow-field velocity measurement were conducted in a 1.2 × 1.2 m2 sealed case to ensure that no cross-flow drafts were present.
FIG. 4 Resuspended particle with different initial seeding patterns. (a) Pattern matching the plate, (b) seeded between center and W/8 from the edge of the plate, and (c) seeded between the center and W/4 from the edge of the plate.
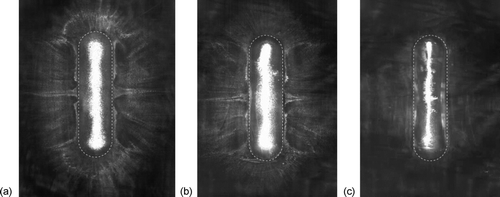
Prior to the laboratory experiment, a velocity time history of the idealized foot motion was generated based on the time-dependent position of a human foot stomping. A sample time history is shown in , that consists of three phases: acceleration from rest, constant velocity, and deceleration to rest to approximate the actual human foot stomping motion. The details of the motion are shown parametrically in . An initial foot height (Zi ), a final gap size (Zf ), and a Reynolds number (Re Uc) were specified, and two different sets of parameters for the downstroke and upstroke motions were selected for the investigation. In order to focus on the aerodynamic effects, the model was stopped 0.1 mm above the acrylic floor.
TABLE 1 Parameters used in the laboratory experiment simulating a stomping motion
Particle flow visualization was conducted with the same condition as in the foot tapping and stomping, such as particle seeding density, a laser sheet, and a high speeds camera. In addition, a high definition SONY Camcorder was used to take the images during the upward motion to get wider viewing area and higher image sensitivity.
A Dantec two-component PIV system was used for quantitative velocity field measurements. Seeding was provided by a Laskin-nozzle type seeder with olive oil (nominal droplet diameter 1–5 μm). The flow field was illuminated in its cross-section with a thin laser light sheet. The PIV image acquisition was triggered at various locations of the stepping cycle so that the results could be phase-averaged. The results were averaged from 50 realizations. The uncertainty in the mean velocity is estimated to be less than 10%, depending on the wall proximity.
A supplementary experiment was also carried out in water to study the evolution of the three-dimensional vortex ring and described in the results section.
3.2. Results of Particle Resuspension with an Elongated Plate
3.2.1. Downstroke
The particle streak pattern left by an elongated plate after the downward motion is complete is shown in . The particles were initially seeded beneath the model foot at particle density 5.6 mg/cm2, and the initial seeding pattern was changed to study the effect of initial particle position on particle resuspension, that is, to study where the resuspended particles came from. The edge of foot is indicated with the broken line in the figures. An initial seeding pattern shown in is matched the elongated plate, is the particles spread over up to W/8 from the edge of the foot and beneath the foot in a racetrack pattern. is the particles seeded beneath the foot up to W/4 from the edge of the foot. The result in indicates the three-dimensional effects due to geometry of the foot. As observed under the disk, the particles were expelled under the foot by an unsteady wall jet. On the other hand, strongly directional particle patterns can be seen at the mid-section of the foot. Streaks in the particle patterns are believed to be associated with flow instability as well as the particle interactions, which was also seen around the disk. also shows the particles left underneath the plate. The initial positions of the particles affected the particle redistribution as observed under the disk (Khalifa and Elhadidi 2007; Kubota et al. Citation2009). Particles near the edge and the mid-section of the foot were expelled more significantly and the rest of the particles were left underneath the foot. From the comparison among the seeding patterns, 44% of particles settled beneath the foot were expelled. shows the particle flow visualizations in side view cross-sections, in both longitudinal and lateral directions, during the downward stomping motion. The initial seeding pattern was just underneath the foot, and all of the experimental conditions were the same as in the bottom view visualization in . The geometrical details of the foot model assembly are also visible in the . The foot was fixed to the linear servomotor by using a flange, and a rod. The foot was attached to a flange, 50 mm in diameter that was connected to the rod fixed to the linear servomotor. The thicknesses of foot and flange were both 12.7 mm. The particles were expelled more in the lateral direction than in the longitudinal direction from under the foot and resuspended. Then, they were redistributed away from the floor. As in the case of the particle resuspension underneath the disk, the cause of particle resuspension was associated with the wall jet with high wall shear stress beneath the foot, and the redistribution is associated with the vortex roll-up and particle entrainment. In the figure, the scale of vortex is clearly larger in the lateral direction than in the longitudinal direction. Also, these results agree with the results of the actual foot motion. The vortex in is more organized due to the straight side edge of the elongated plate. The vortex dynamics are associated with the redistribution of particles, and the larger scale vortex was evident in the mid-section of the foot.
FIG. 6 PIV velocity field measurements in downstroke stomping motion of an elongated plate. (a) Lateral cross section and (b) longitudinal cross section.
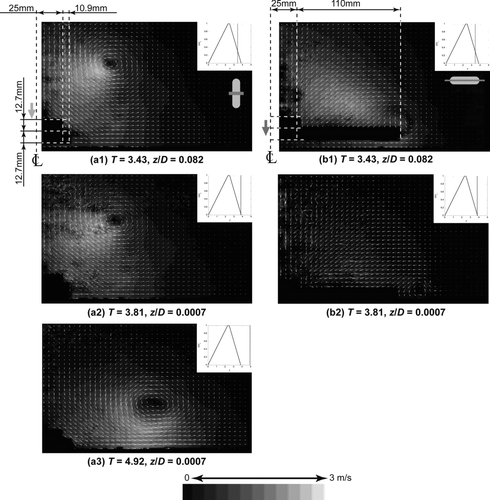
Next, the associated flow-field was investigated quantitatively with the PIV system. The velocity field measurements of the elongated plate during the downstroke stomping motion are shown in along the lateral cross section and in along the longitudinal cross section, respectively. Small insets depict the relative timing of the instantaneous velocity field as well as the orientation of the cross section. In , a large wake vortex is formed behind the plate. Note the horizontal location of the vortex center is outside the plate width. Shortly after the plate stopped, , the vortex impinged the floor and together with the wall jet from the gap the high velocity region is formed near the wall. The highest speed flow motion continued outward even at T = 4.92 in . In the longitudinal cross section, large amount of flow was entrained behind the plate in . However, after the plate stopped, only the small-scale vortex was present near the floor and magnitude of velocity in this cross section was already substantially diminished by this time. In comparison, the induced velocity in the lateral cross section was stronger, with the velocity indicated to be of the order of 3 m/s in this figure, and the vortex core is further away from the plate. The larger lateral velocity may be heuristically explained by the difference in pressure gradient between the stagnation region under the plate and the external region across the varying distance. This larger scale vortex is also evident in the particle flow visualization in . The vortex formed in the wake of the plate and impacted the floor as the plate decelerated, in a similar manner as observed behind decelerated disk (Higuchi et al. 1996). The high velocity region corresponds to the strong particle streaks from the mid-section of the foot in . A strong wall jet was also observed underneath the elongated plate when it was stopped above the floor at 0.1 mm height, creating high wall shear to expel the particles from under the elongated plate. The velocity profiles between the elongated plate and the floor for the downward stomping motion were similar to those in the channel flow with maximum velocity in the middle. For example, at T = 3.52, Z/D = 0.054, the maximum velocity within the gap was 1 m/s in lateral direction, and 0.65 m/s in longitudinal direction. The velocity was higher at smaller gap size. Note in the figure with combined wall jet and the vortex generated over 3 m/s velocity after the plate stopped in the outer region near the floor. However, when the plate was closer to the floor the velocity profile measurement was subject to large uncertainty, let alone determining the instantaneous wall shear stress. The PIV velocity field measurement at proximity to the floor was impaired by the reflection of the laser light sheet. Assessment of the particle detachment and resuspension as well as the vortex dynamics behind the elongated plate is addressed later.
3.2.2. Upstroke
The results of particle flow visualization and velocity field measurements during upward motion by the elongated plate are shown in . The masked areas in the figures correspond to the model foot. For particle flow visualization, the particles were initially seeded beneath the plate matching the plan form of the plate. In both longitudinal and lateral directions, the particles became airborne from the wall. In the longitudinal direction in , the resuspended particles were entrained behind the upward moving foot. In the lateral direction in , the particle entrainment was into a more extended region behind the foot. A strong vortex pair extending far beyond the plate width can be seen in this cross section. The results of velocity field measurements are shown in . Large concentrations of resuspended particles corresponded to the high velocity region under the foot in both longitudinal and lateral directions. During this upstroke motion, the effect of the three-dimensional vortex deformation is evident.
3.3. Supplementary Flow Visualization in Water and Vortex Simulation Behind an Elongated Plate
As a supplementary study, vortex formation behind the elongated plate was visualized with dye in the water tank. The results of particles resuspension as shown in show the significant expulsion of particle from the mid-section of foot. The comparison between circular foot and elongated foot shows that the significant pattern with elongated foot caused with the difference of foot. In other words, the significant pattern was related to the three-dimensionality of vortex formation, since the particle redistribution was related to the vortex formation behind the foot. Thus, the process of three dimensional vortex formation was investigated both experimentally and numerically. The fluorescence dye under the UV light was used to illuminate the flow structure. Initially the elongated plate was coated uniformly with the dye mixture and the wake behind the foot during the motion was observed from the top and the side. A high-speed camera was operated at 60 fps to take the images. The downward foot movement was reproduced with dropping motion. The parameters of Zi , Zf , and Re Uc in were kept the same as in the idealized downward stomping motion in air.
FIG. 9 Particle resuspension with a slipper in downward stomping motion, bottom view. (a) T = 3.71 and (b) T = T final.
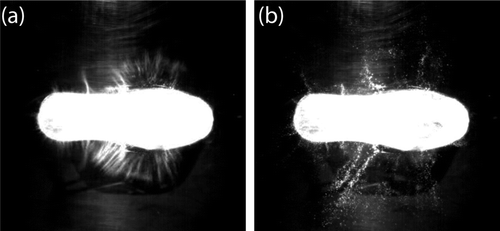
The sequence of vortex deformation with the dye flow visualization in the top view is shown in . In the results of the dye flow visualization, t = 0 s corresponds to the time the foot stopped from the downward motion. At t = –0.5 s, the vortex can be seen behind the foot in the wake region. In t = –0.4 s, the vortex has started to stretch from the minor axis. At t = 0 s, the axis of vortex has switched. This phenomenon of axis switching agreed with the results of the side view (not shown). The vortex continued to stretch in lateral direction after the foot stopped (t > 0 s). The strong streaks from the mid-section of the foot in are also related to the effect of axis switching of vortex and vortex stretching after the foot stopped. The deformation of the vortex and axis switching were also visible behind the upward moving model foot in . A simple three-dimensional vortex blob method was applied to the present geometry and the top view sequence of vortex deformation with the numerical simulation is shown in . The computed results show the out-of plane vortex deformation and the vortex axis switching, and they are in general agreement with the dye flow visualizations. This numerical result indicates the relation between foot shape and redistribution of particles. This supplementary study clarified the deformation of vortex during the foot motion, and also indicated an evolution of vortex geometry to grow in the lateral direction. In short, the elongated plate caused a strong three-dimensional flow which affected the particles redistribution. Higuchi et al. (1996) studied start-up wake formations behind polygonal plates, that is, square, triangle, hexagonal, and octagonal plates, where three-dimensional vortex stretching and curvature effect caused large deformation of non-circular vortex rings. Kiya and Abe (Citation1999) studied the steady state turbulent wakes behind an elliptic plate and a rectangular plate along both major axis and minor axis. Toyoda et al. (Citation1994) measured the coherent structures behind rectangular jets of various aspect ratios and identified deformation of noncircular vortex rings. Zaman (Citation1996) examined the vortex axis switching with an elliptic jet. It is evident that these three-dimensional vortex dynamics are playing significant role in the present problem of impacting three-dimensional geometry.
3.4. Particle Resuspension with a Model Foot Wearing an Indoor Slipper
The resuspension of particle underneath a commercially available indoor slipper during downward stomping are shown in . The particles were initially seeded just beneath the slipper. At T = 3.71 when the slipper stopped at 0.1 mm above the floor, the ejected particles were mostly from the mid-section of the slipper. Later at T = T final, particles were redistributed to the diagonal direction in the mid-section of the slipper as shown in .
The side cross-sectional views of particles resuspended and airborne by the downward moving slipper were carried out (not shown). The observation focused on the typical resuspension in . The particles were ejected diagonally from the mid-section as seen in the bottom view in . Thus, the laser light sheet was directed in this diagonal plane. Diagonal expulsion in shows the strong wall jet that projected the resuspended particle distance away from the sole. Strong three-dimensionality of the flow-field was evident.
4. DISCUSSIONS AND CONCLUSIONS
The experiments for the particle resuspension and particle redistribution were carried out with the human foot tapping, the human foot stomping and the mechanical foot motion. The particle resuspension and particle redistribution occurred during both upward motion and downward motion in all cases.
From the results of the laboratory experiments using an elongated plate and a slipper moving vertically toward or away from the wall, the strong three-dimensional aerodynamic effects were clarified in comparison to the axisymmetric results with the disk. Fundamental mechanism of the particle resuspension and redistribution, however, was common in both cases, with a strong wall jet and vortex motions. The resuspension and redistribution of particles from the mid-section of the elongated plate foot more significant than those from other section of the plate. The strong particle streaks were observed from the mid-section and were affected by a stronger wall jet and induced velocity by the deformed vortex ring. The three-dimensional vortex dynamics particularly affected the strength of redistribution of already resuspended particles. Also, the particle resuspension and redistribution during the upward motion were driven by the highly three-dimensional wake structure. The wake axis switching was also evidenced by the present numerical simulation and dye flow visualization. The laboratory experiment with an indoor slipper showed a pattern of the particle resuspension that was more convoluted and three-dimensional with diagonal wall jets. The floor was lightly loaded with particles for the experiment emulating typical “dusty” floor, but nonetheless, it is believed that there were multiple layers of particles and thus less amount of the friction velocity was needed to resuspend the particles than needed to detach single layer of particles against adhesion forces to the floor.
We will briefly review our results in light of previous analytical information. Applying Stokes’ law, the time constant for particles to follow velocity field is given as example, Merzkirch (Citation1987).
In general, the response of the particle is the faster with the smaller the particle size and the density ratio ρP/ρT. This equation qualitatively explains the possible lag between particle speed and flow velocity. Stokes’ law determined that Reynolds number is less than 1, so the significant size of particle for resuspension can be calculated as dp ≤ 15 μm. Since the EquationEquation (1), it is estimated that the particles used in this experiment accelerate to 95% of their surrounding fluid velocity within 1.5 ms. Furthermore, the sedimentation velocity is estimated to be negligibly small compared to the duration of the present experiments. Thus, once particles are resuspended, they are entrained into the unsteady flow field induced by the foot motion, except into the core region of the vortex where the velocity gradient is very high and this region is mostly void of the particles as illustrated by the dark center region of vortices of the particle visualizations as shown in . This is also demonstrated by the fact that the particle visualization closely matched the velocity field measurement, the latter using much lighter and smaller oil droplets. As demonstrated, the present velocity field is sufficient to levitate the detached particles from the floor and redistribute away from the floor.
One remaining question is what is the relation between the resuspension and the adhesion force. The particles have adhered to the floor with microscopic forces such as surface tension and Van Der Waals forces and have become detached (Soltani and Ahmadi 1994). Taheri and Bragg (Citation1992) performed an experiment on detachment of glass particles of 20 μm and 35 μm diameter from a glass substrate under a steady turbulent boundary layer. They observed 40% removal at the wall shear stress, U τ around 1 m/s. This was higher than the rolling detachment and lower than that for sliding detachment in dry air (Soltani and Ahmadi 1994). Zimon's measurement showed that from a steel surface 20 μm diameter (the smallest diameter tested) glass particles required the steady-state friction velocity, U τ, of about 0.8 m/s (Zimon 1982). Soltani and Ahmadi (1994) predicted that the turbulent boundary layer burst model gives a slightly lower required wall shear stress than the steady state sublayer model.
The present experiment involved an impulsive wall jet and vortex impingement near the wall, and the measurements did not resolve the region immediately next to the floor in submicron scale to assess particles detachment. Particles are likely on the floor in multiple layers rather than in a single layer attached via molecular forces. However, the following assessment could be made. In the present case, the velocity of the wall jet underneath the foot and consequently the wall shear stress was larger in the lateral direction than in the longitudinal direction, corresponding to more particle resuspension in the lateral direction. If we assume approximately 3 m/s jet velocity shown in exited the final plate gap, the estimated friction velocity, U τ of approximately 0.5 m/s was sufficient to detach and suspend 10 μm diameter particles on the floor based on Khalifa and Elhadidi (2007), utilizing Soltani and Ahmadi's analysis (1994) cited above. Incidentally, it is of interest that their incompressible flow numerical analysis on falling disk predicted the wall jet velocity of the order of 100's of m/s, which was not observed in the present study, even taking into account of the uncertainty and limited resolution of the present velocity measurements, and further three-dimensional numerical study is warranted using realistic foot motions. In the typical dusty environment, it may be necessary to examine multiple layer of particles for practical applications rather than whether a single layer of particles that have adhered to the surface are indeed resuspended in the present experiment, given the evidence of particle resuspension and redistribution into the air as evidenced in this study. In practice, the flooring such as carpet, and the sole geometry of the shoe start to play major roles.
With a human subject tapping or stomping his foot, we documented particle resuspension. The subject was wearing the same slipper tested in the laboratory. In the downward foot motion, the highest speeds were found in the wall jet between the foot and the floor causing particle resuspension, and the vortex formed in the wake of the foot, resulting in the redistribution of the particles. On the upward motion, the particles were resuspended by a wall jet formed by the rapid inflow of air into the low-pressure wake region underneath the foot. The present results also showed the importance of large-scale flow structures such as the wake vortices for the redistribution of particles over large distance. The large-scale flow structures and the particle redistribution are affected by walking kinematics as well as sole geometry. With additional forward motion of the foot, the suspended particles will be carried in the wake region behind the leg itself.
NOMENCLATURE
Acc | = |
acceleration in motion |
Dec | = |
deceleration in motion |
Re Uc | = |
Reynolds number based on disk diameter, U c D/υ |
T | = |
normalized time, U c t/D |
t | = |
time, s |
T 1 | = |
timing at the end of acceleration phase |
T 2 | = |
timing at the end of constant velocity phase |
T 3 | = |
timing at the end of deceleration phase |
T final | = |
defined as 5 s after T 3 |
U | = |
foot velocity |
U c | = |
constant foot velocity |
ρF | = |
density of fluid |
ρp | = |
density of particles |
τw | = |
wall shear stress |
Uτ | = |
friction velocity, |
Acknowledgments
This work was carried out at Syracuse University and was supported in part by the New York State STAR Center for Environmental Quality Systems and the US Environmental Protection Agency (US EPA grant/cooperative agreement Award #CR-83199201-0). Although this research has been funded in part by the US EPA, it has not been subjected to the Agency's required peer and policy review, and therefore does not necessarily reflect the views of the Agency and no official endorsement should be inferred. Dr. Hiroshi Higuchi, one of the authors, passed away on 23 November 2010 after he finished working on this article. I am deeply grateful to Dr. Higuchi.
REFERENCES
- Eames , I. and Dalziel , S. B. 2000 . Dust Resuspension by the Flow Around an Impacting Sphere . J. Fluid Mech. , 403 : 305 – 328 .
- Ferro , A. R. , Kopperud , R. J. and Hildemann , L. M. 2004 . Elevated Personal Exposure to Particulate Matter from Human Activities in a Residence . J. Exp. Anal. Environ. Epidemiol. , 14 : S34 – S40 .
- Higuchi , H. , Anderson , R. W. and Zhang , J. 1996a . Three-Dimensional Wake Formations Behind a Family of Regular Polygonal Plates . AIAA J. , 34 : 1138 – 1145 .
- Higuchi , H. , Balligand , H. and Strickland , J. H. 1996b . Numerical and Experimental Investigations of the Flow Over a Disk Undergoing Unsteady Motion . J. Fluids Struct. , 10 : 705 – 719 .
- Khalifa , H. E. and Elhadidi , B. 2007 . Particle Levitation Due to a Uniformly Descending Flat Object . Aerosol Sci. Technol. , 41 : 33 – 42 .
- Kiya , M. and Abe , Y. 1999 . Turbulent Elliptic Wakes . J. Fluids Struct. , 13 : 1041 – 1067 .
- Kubota , Y. , Hall , J. W. and Higuchi , H. 2009 . An Experimental Investigation of the Flowfield and Dust Resuspension Due to Idealized Human Walking . J. Fluids Eng. , 131 081104–1-081104–6
- Leweke , T. , Thompson , M. C. and Hourigan , K. 2004 . Vortex Dynamics Associated with the Collision of a Sphere with a Wall . Phys. Fluids , 16 : L74 – L77 .
- Merzkirch , W. 1987 . Flow Visualization , Academic Press, New York .
- Soltani , M. and Ahmadi , G. 1994 . On Particle Adhesion and Removal Mechanisms in Turbulent Flows . J. Adhes. Sci. Technol. , 8 : 763 – 785 .
- Taheri , M. and Bragg , G. M. 1992 . A Study of Particle Resuspension in a Turbulent Flow Using a Preston Tube . J. Adhes. Sci. Technol. , 15 : 15 – 20 .
- Thatcher , T. L. and Layton , D. W. 1995 . Deposition, Resuspension, and Penetration of Particles within a Residence . Atmos. Environ. , 29 : 1487 – 1497 .
- Toyoda , K. , Okamoto , T. and Shirahama , Y. 1994 . Eduction of Vortical Structures by Pressure Measurements in Noncircular Jets . Appl. Sci. Res. , 53 : 237 – 248 .
- Winter , D. A. 2005 . Biomechanics and Motor Control of Human Movement , Hoboken, NJ : John Wiley & Sons .
- Zaman , K. B. M. Q. 1996 . Axis Switching and Spreading of an Asymmetric Jet: The Role of Coherent Structure Dynamics . J. Fluid Mech. , 316 : 1 – 27 .
- Zimon , A. D. 1982 . Adhesion of Dust and Powder , New York : Plenum Press .