Abstract
In this article, a new approach was established to estimate the fractional contributions of soil dust, biomass burning, biogenic emissions, coal burning, and vehicle exhaust to the carbonaceous particulate matter by carbon isotope and linear regression techniques of OC-K+ (organic carbon) and OC-EC (elemental carbon). Using the method described herein, the fractional distributions of these sources were quantitatively determined for the OC and semiquantitatively for the EC in the size-resolved particles (size ranges: <0.49, 0.49–0.95, 0.95–1.5, 1.5–3.0, 3.0–7.2, and >7.2 μm) collected in Jiading District, a suburb of Shanghai, China. Distinct size distribution of contributions of these sources to the OC and EC was observed. Generally, biomass burning contributed a large fraction to OC in the smaller particles and biogenic emissions shared a bigger fraction to OC in the larger particles. Soil dust made contributions solely to the OC, for no EC fraction was found in the soil dust. OC from coal burning concentrated in the fine particles (smaller than 3.0 μm), and that from vehicle exhaust exhibited bimodal distribution, with peaks for both fine and coarse particles. The fossil sources dominated EC in almost all the size ranges. Though a few deviations are brought about in the calculation, this approach provides an effective way to distinguish the sources of the carbonaceous particulate matter.
Copyright 2013 American Association for Aerosol Research
INTRODUCTION
Carbonaceous matter is a ubiquitous component of ambient particulate matter, and often makes up the largest fraction of submicrometer particulate matter in terrestrial regions (Andreae and Crutzen Citation1997; Schwier et al. Citation2010). It mainly consists of organic carbon (OC), elemental carbon (EC), and carbonate carbon (CC). It plays a significant role in global and regional climate change, visibility reduction, and negative effects on both the environment and human health (Colburn and Johnson Citation2003; Nel Citation2005; Andreae Citation2007; Rosenfeld et al. Citation2008; Gustafsson et al. Citation2009; Jimenez et al. Citation2009). Consequently, it is necessary to estimate fractional distributions of various sources for decreasing and eliminating the carbonaceous particulate matter.
Two kinds of approaches to the source apportionment of carbonaceous matter are found in previous researches. On the one hand, individual molecules are used for the tracers of certain emissions, such as cholesterol for meat cooking, levoglucosan for biomass burning, hopanes and steranes for vehicle exhaust, and 2-methylglyceric acid, 2-methylthreitol, and 2-methylerythritol for isoprene biogenic secondary organic carbon (Ding et al. Citation2008; Kleeman et al. 2008a,b, 2009; Fu et al. 2010). Using the ratio of specified molecule/OC from the emissions, the contributions of specific sources can be obtained. On the other hand, studies utilize chemical models of source orientation based on source information and transport calculation to predict the carbonaceous species from certain emissions at specific sites, or the receptor-oriented models to determine the source contributions by calculating the best liner fittings of a series of tracer molecules between the source profiles and the receptor sites Chan et al. 1999; Holmes and Morawska Citation2006; Yuan et al. Citation2006). These methods are based on the premise that the organic tracers and other chemical components remain inert and do not participate in the chemical reactions, which is impossible upon the presence of other active species (Gelencsér et al. Citation2007; May et al. Citation2012). As a result, the approaches to carbon source apportionment at the molecular level may bring high and unknown uncertainties.
Radiocarbon (14C) is an ideal tracer to distinguish the fraction of fossil fuels from contemporary components in the carbonaceous matter (Petsch et al. Citation2001; Reddy et al. Citation2002; Bennett et al. Citation2008; Gustafsson et al. Citation2009; Fushimi et al. Citation2011). Since the contemporary sources mainly consist of biomass burning, biogenic emissions (secondary organic carbon [SOC] from the biological volatile organic compounds [BVOCs]), and soil dust, their 14C values are different from each other. The contributions of the contemporary sources to the carbonaceous matter may be estimated based on the mass balance of 14C. Additionally, the fossil fuels have their identifiable stable carbon composition (δ13C); for example, the δ13C value of Chinese coal is –24.7‰ to –22.5‰ (Tang Citation2001) and the average value of –27.3‰ for fossil fuels (Lichtfouse et al. Citation2002). According to the mass balance of 13C, contributions of coal burning and vehicle exhaust to the carbonaceous matter may be further estimated based on the assumption that the fossil fuel emissions mainly include coal burning and vehicle exhaust.
Shanghai is the largest commercial and industrial city in China, with a population of more than 20 million. Accompanied by the rapid development of the economy, both the increase in industry and variations in urban construction have led to tremendous energy consumption. Consequently, the poor air quality, such as the lower visibility and higher concentrations of the carbonaceous matter in the ambient particulate matter, has drawn great attention. However, only a few studies about the qualitative source apportionment of carbonaceous matter have been conducted in this area (Ye et al. Citation2003; Feng et al. Citation2006). The objectives of this research include: (1) trying to establish an approach to quantitatively estimate fractional contributions of soil dust, biomass burning, biogenic emissions, coal burning, and vehicle exhaust to the size-resolved carbonaceous matter by using 14C, 13C, and linear regression techniques, and (2) quantitatively estimating the proportions of these contributors to the OC and EC in size-resolved particles in the Shanghai suburb.
EXPERIMENTAL
Basic Principle: Source Apportionment with Carbon Isotope Composition
The major fossil emissions from coal burning, diesel exhaust, motorcycle exhaust, and other vehicle exhaust, and but no oil combustion particulates were found at the same sampling site of this research using the individual particles analysis (Yue et al. Citation2006). So, the fossil sources of carbonaceous matter can be limited to coal burning and vehicle exhaust. Considering the situations at the sampling site and data from the above research, soil dust, biological fragment, and biomass burning are defined as the primary contemporary sources. Besides, the noncombustion OC, which is mainly considered as SOC derived from BVOCs, should be taken into account, especially at the rural site (Lemire et al. 2002). The carbonaceous particles from long-distance transport are almost impossible to quantitatively determine, and their impacts on the carbon isotope of the atmospheric particles are neglected. Thus, the sources of carbonaceous matter in this research can be divided into contemporary and fossil categories. The former consists of biomass source (biomass burning and biogenic emissions) and soil dust, and the latter consists of coal burning and vehicle exhaust. Fractional distributions of contemporary components of the OC and EC in aerosols can be approximated by 14C concentration, which is expressed as a unit of percent modern carbon (pMC):
Since the contemporary sources consist of soil dust and biomass source, the fractional contributions of soil dust and biomass source can be calculated based on 14C mass balance:
Although 14C is in free state in fossil fuels, the δ13C values are different in the particulates derived from coal burning and vehicle exhaust. Then the fractional contributions of coal burning and vehicle exhaust can be calculated based on the mass balance of δ13C values. Here, the δ13C is expressed as
Due to the limitation of the model, the sources should be restricted to biomass source (biomass burning and biogenic emissions), soil dust, coal burning, and vehicle exhaust, as presented in EquationEquations (4) and Equation(7). The biogenic emissions are not combustion sources and do not produce EC; thus, the biomass source refers to biomass burning for EC. The contributions of these sources to EC can be partitioned by Equations (1)–(8). However, the OC derived from biomass finds its source in biomass burning and biogenic emissions, so the determination of pMCb and δ13Cb is the key to obtain the fractional contributions of these sources.
Determination of pMC and δ13C of Biomass Source
The pMC and δ13C of biomass burning OC can be obtained by collecting the particles from this source. OC from the biogenic emissions is the oxidized product of VOCs emitted by vegetation, so the pMC should be the same as that of living plants. The pMC of new leaves and grass around the sampling site is reasonable to be used as those of biogenic emissions. Thus, it is necessary to obtain the proportions of biomass burning and biogenic emissions contributed to the biomass source to determine the pMC and δ13C of biomass source.
Potassium is regarded as an effective tracer of biomass burning. OC is emitted simultaneously with potassium during biomass burning, so OC derived from biomass burning can be estimated based on the linear regression of OC-K+ (Duan et al. Citation2004). Besides, the intercept for the linear regression of OC-EC is considered as the noncombustion-derived OC (Chu Citation2005; Saylor et al. Citation2006). The linear regressions for OC-K+ and OC-EC are used to distinguish the OC from biomass burning and biogenic emissions, with a total of 29 sets (174 filters) of samples collected from April 2008 to October 2010 (Zeng et al. Citation2012) at the same sampling site. In brief, as the K+ approaches zero, the intercept of OC-K+ is considered as the biogenic fraction. The slope represents the ratio of biomass burning OC and potassium. We should note that the K+ from nonbiomass-burning emissions would lead to an overestimation of biomass burning unless it is subtracted. The biomass burning-derived K+ bb can be expressed as
The biomass OC is the sum of biomass burning OC (OCbb) and biogenic OC (OCbiogenic). So, the pMC and δ13C of the biomass source can be calculated as
The standard δ13C value for C3 and C4 plants is –27‰ and –13‰, respectively (Deines Citation1980). The C4 plants in Shanghai account for about 2% of the total vegetation area (http://zzys.agri.gov.cn/nongqing.aspx). So, the δ13C of biogenic OC can be determined as: δ13Cbiogenic = (–27‰) × 98% + (–13‰) × 2% = –26.72‰. The OCbb and OCbiogenic can be obtained by the linear regressions of OC-K+ and OC-EC, and the pMCbb and δ13Cbb can be determined by the analysis of particles from biomass burning. pMCbiogenic is regarded as the same as that of new leaves and grass. Using EquationEquations (11) and (12), the pMC and δ13C of biomass source can be determined, and then, the fractions of soil dust, coal burning, and vehicle exhaust can be obtained using EquationEquations (1)–(8).
Sample Collection: Size-Resolved Particles
The sampling was carried out on the rooftop of a five-story building at a height of about 18 m at the Shanghai Institute of Applied Physics (31°24′N, 121°17′E), which is located in the suburbs, 5 km northeast of Jiading urban area, and 20 km away from downtown Shanghai. Jialuo Road and Liuxiang Road are located 420 m and 410 m in the south and the east of the sampling site, respectively. The institute is surrounded by three small villages, and widespread cultivated fields. Each of the villages has a small boiler, which is used for boiling water for the residents, using waste wood as fuel. A hostel of the institute is located about 500 m southwest of the sampling site, with a boiler using coal as fuel for its heating system.
Size-resolved ambient aerosols were collected using six-stage cascade impactors (M235 TFIA-2; Staplex, New York, NY, USA), with aerodynamic equivalent diameter in size ranges of <0.49, 0.49–0.95, 0.95–1.5, 1.5–3.0, 3.0–7.2, and >7.2 μm. The quartz substrates were precombusted at 850°C for 5 h to eliminate the background carbon pollution (Cao et al. Citation2011). Twenty-nine sets (174 filters) of samples were collected from 3 April 2008, to 30 October 2010 for the linear regression of OC-K+ and OC-EC. The sampling details are described in our previous study (Wang et al. Citation2012). The samples for the carbon isotope analysis were collected from 1 May to 3 May, 11 May to 13 May, and 19 May to 21 May 2012.
Source-Emitted Particles and Atmospheric CO2
Quantitatively estimating the sources of carbonaceous particles depends on the carbon isotope compositions in both particles derived from the five origins: soil dust, biomass burning, biogenic emissions, coal burning, vehicle exhaust, and atmospheric CO2, according to the Equations (2)–(12). Two sites without any vegetation were selected for sampling soil cores, as shown in . The soil at a depth of 0–3 cm was collected with a soil sampler (ϕ = 3 cm), dried in a furnace at 80°C for 24 h, ground and then filtered with a 200 mesh sieve. Three samples were collected at each site. The sieve-filtered soil was resuspended in a closed chamber with a fan, and the size-resolved soil dust was collected by the same six-stage cascade impactors. For the biomass burning, the size-resolved particles derived from wood burning were collected in the outlet of chimneys of the three boilers. Additionally, the BVOCs are directly exhaled by vegetation, some of them transform to noncombustion OC as SOC. We assume that little isotope fractionation exists in this transformation, so the pMC of noncombustion OC should be considered to have the same values as those of the new leaves and grass. A mixture of newborn leaves (Cinnamomum hupehanum and spruce) and grass were collected, cleaned with the deionized water, dried at 80°C for 48 h, and ground for further experiment. Only the PM2.5 originating from vehicle exhaust was collected at the center of the Out Ring Tunnel in Baoshan District. This tunnel is one of the heaviest traffic zones in Shanghai, with all types of vehicles passing through. Its length is 2880 m. The coal used in Shanghai originates from Shanxi, China, and the δ13C values of Chinese coal have been measured to be from –24.7‰ to –22.3‰ (Tang Citation2001). Since the differences in Chinese coal types are not large, the average δ13C value of Chinese coal was selected to be that of particulate matter derived from coal burning.
The atmospheric CO2 was sampled at Lin’an national background station (30°18′N, 119°44′E) on the observation platform at a height of 139 m, about 200 km from Shanghai. The sampling was performed at 7:00 am, 17:00 pm, and 21:30 pm, using a personal air sampler (LP-7; A.P. BUCK Inc., Orlando, FL, USA; flow rate: 5.18 L·min−1). About 25 L of air was pumped and stored in sealed gas bags for each sample.
TABLE 1 The averaged carbon isotope composition of atmospheric particlesa
Carbon Isotope Analysis
The carbonate carbon (CC) should be evaluated carefully during the carbon isotope analysis owing to its higher abundance of 13C (Wang et al. Citation2005; Koulouri et al. Citation2008; Ho et al. Citation2011), which may lead to a positive artifact in the results. The pH of the particulate matter solution before and after acid treatment (Yamamoto et al. Citation2007) was measured. The solution of untreated particulate matter smaller than 3.0 μm was acidic, while it was alkaline for the matter larger than 3.0 μm, with pH of 5.1–6.2 and 7.3–8.2, respectively. The alkaline solution indicated the probable existence of CC. The pH of the acid-treated aerosols was less than 3.4. So, in this study, aerosols larger than 3.0 μm were treated with hydrochloric acid.
A two-step combustion procedure was adopted for separating OC and EC (Szidat et al. Citation2004b). In brief, the OC was combusted at 340°C for 3 h, with the pure analytical grade O2 and N2 as carrier gas (flow rates, O2: 50 ml·min−1, N2: 50 ml·min−1). After that, the EC was combusted at 850°C for 3 h (flow rates, O2: 80 ml·min−1, N2: 20 ml·min−1). The CO2 in the off-gas was purified through a gas-purified system (Moriizumi et al. Citation2004). About 1.5 mg of carbon of the purified CO2 was graphitized to determine 14C with a tandem accelerator mass spectrometer (High Voltage Engineering Europe, Amersfoort, The Netherlands) (Liu et al. Citation2006). Approximate 0.5 mg of carbon of the purified CO2 was introduced into an isotope ratio mass spectrometer (DELTA V Advantage; Thermo Scientific, MA, USA) to measure the δ13C. Blank correction was performed as follows: the two-step combustion procedure was carried out without particulate matter samples and the off-gases were collected. The CO2 in the off-gases was purified in the same way as described for the particulate matter.
According to the research by Szidat et al. (Citation2004a), by doing the water extraction prior to the EC analysis, the pyrolyzed organic carbon (OP) is mistaken as part of the EC, accounting for about 30% of this fraction. So, the amounts of pMC and δ13C of the EC obtained by the two-step procedure are influenced by those of pyrolyzed carbon.
OC/EC Analysis
Samples were analyzed for OC and EC following the Interagency Monitoring of Protected Visual Environments (IMPROVE) protocol (Chow et al. Citation2001, Citation2002; Cao et al. Citation2004) by a thermal and optical carbon analyzer (DRI model 2001A; Desert Research Institute, NV, USA). For quality control, the analyzer was calibrated using the standard sucrose solution every day. Duplicate punches from each sample were analyzed to minimize the influence from the nonuniform depositions on the filter. Replicate analyses were performed with 10% of total samples, and the differences indicated by replicate analyses were within 15% for OC and 10% for EC. Filed blanks were analyzed and subtracted (Wang et al. Citation2012). A total of 29 sets (174 filters) samples were analyzed for OC and EC.
Potassium Analysis
A part (size-resolved slot 12.33 cm2, size-resolved filter 2*D17.4-mm punch) of each sample was selected through perforation and extracted ultrasonically for 40 min by 5 ml ultrapure water (18MΩ·cm−1). Potassium was analyzed by ion chromatography (Dionex ICS2000, Dionex Corporation, CA, USA) using a separation column (Dionex CS12A; Desert Research Institute, NV, USA). The relative standard was within 5% for the reproducibility test. The detection limit of K+ was 0.003 mg·l−1. The blanks of each quartz substrates were analyzed and subtracted. A total of 29 sets (174 filters) samples were analyzed for potassium.
RESULTS AND DISCUSSION
Carbon Isotope Compositions of the Size-Resolved Particulate Matter and the Reference Samples
The carbon isotope compositions of the size-resolved particulate matter and the reference samples are presented in and , respectively.
TABLE 2 The carbon isotope composition of the reference materials
The averaged δ13COC varied from –27.21‰ to –26.02‰, while δ13CEC varied from –26.03‰ to –24.80‰. This result was very close to that from the research on the southern cities in China, including Shanghai by Cao et al. (Citation2011) using the same treatment. The averaged pMCOC ranged from 31.0% to 69.3%, and pMCEC ranged from 42.3% to 53.7%, implying that the biomass source played a considerable role in the contributions to the OC and EC.
shows that the average pMC of OC from the wood burning was 113.0–134.6%, corresponding to the trees 20–50 years old (Levin and Kromer Citation1997). This result is in good agreement with the age of the waste wood from old houses and fragments of the wood used for making pianos. The pMC of atmospheric CO2 in this research is 105.1%, similar to the recommended value of 103.5–104.5% in the United States. Besides, the pMC of newborn leaves and grass is 103.8%. This result is consistent with the values at the rural site in Beijing (Xi et al. Citation2011).
Additionally, shows that the δ13C and pMC of the soil dust were –26.71‰ to –22.16 ‰, and 48.1% to 71.4%, respectively. The depleted 14C in the soil dust is attributed to the fossil fuel-related deposited dust. Rethemeyer et al. (Citation2004) found that the pMC of bulk soil in Germany urban roadsides was 54% due to the contribution of fossil carbon. The soil dust described herein is a combination of soil and resuspended dust. The δ13COC of the PM2.5 from vehicle exhaust was –28.44‰, and the δ13CEC was –26.75‰. Since the δ13C of petroleum ranged from –20‰ to –35‰, with a stronger peak value of –29‰ (Sakai and Matsuhisa Citation1996), the δ13C values derived from vehicle exhaust were reasonable. The δ13C of Chinese coal ranged from –24.7 ‰ to –22.3‰, and the δ13C values within Chinese coal were not largely variable. Hence, its average value of –23.5‰ was reliably selected.
Carbon Isotope Composition of the Biomass Source
The fractions of biomass burning and biogenic emissions to the biomass source are determined as described in the “Basic Principle” section. Using Equations (11) and (12) and the data in , the carbon isotope composition of the biomass source was obtained.
Quantitative Source Apportionment of OC
Combined with the data in – and Equations (1)– (12), the factional contributions of the major sources to OC are determined and presented in .
TABLE 3 The carbon isotope composition of OC from the biomass source
FIG. 2. Size-fractional distributions of soil dust, biomass burning, biogenic emissions, coal burning, and vehicle exhaust to the OC of atmospheric particulate matter in Jiading, Shanghai. (Data are expressed as average value and standard deviation of the three sets samples). (Color figure available online.)
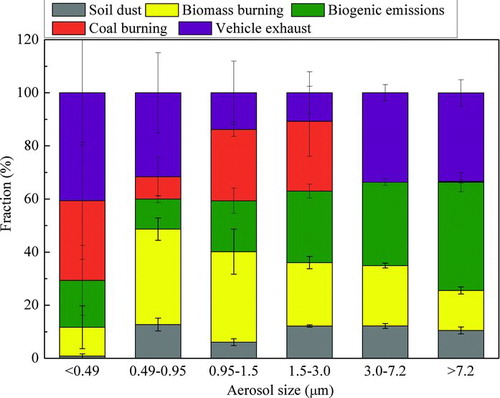
FIG. 3. Size-fractional distributions of biomass burning, coal burning, and vehicle exhaust to the EC of atmospheric particulate matter in Jiading, Shanghai. (Data are expressed as average value and standard deviation of the three sets samples.) (Color figure available online.)
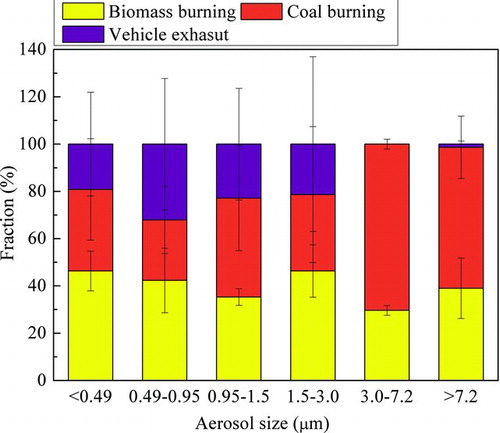
Among these sources, soil dust contributed 1–12% to the OC in particles of different sizes. It contributed the least fraction to OC in particles smaller than 0.49 μm, while contributed larger fractions to OC in aerosols of 0.49–0.95 μm and those larger than 1.5 μm. This may be explained by the fact that the soil dust in this research is a mixture of soil and resuspended dust. The fraction in particles of 0.49–0.95 μm seems more easily to resuspend into the atmosphere, and the part in the coarse mode derives directly from the soil (Zhang et al. Citation2003; Zhao et al. Citation2003). Biomass burning was the largest contributor to the OC in the particulate matter of sizes 0.49–0.95 μm and 0.95–1.5 μm, accounting for 36% and 34% to the corresponding OC, respectively. For the particles larger than 0.49 μm, the contribution of biomass burning decreased with the increase in particle size. However, the contribution of biogenic emissions demonstrated an opposite trend to the biomass burning, sharing a fraction of 11–41%. The characteristics of the two sources are effectively reflected by their fractional distributions: the particles from the wood burning and newly formed secondary organic carbon are of submicrometer size (Pandis et al. Citation1993; Silva et al. Citation1999; Bonn and Moortgat Citation2002), but the bioparticulate matter, such as pollen, spores, and bacteria, are coarse particles (Ariya and Amyot Citation2003).
For the fossil component, the coal burning contributed 0–30%, and vehicle exhaust contributed 11–40% to the OC in particles of different sizes. The coal burning contribution concentrated in particulate matter smaller than 3.0 μm, and contributed almost no fractions to aerosols larger than 3.0 μm. The fractional contribution of vehicle exhaust to the OC shows a bimodal distribution, with peaks in size ranges of less than 0.49 μm and larger than 3.0 μm. The peak mass of the fossil fuels derived particulates in the range of 0.1–0.52 μm (McElroy et al. Citation1982; Hildemann et al. Citation1991; Kleeman et al. Citation2000). So, we assume OC from coal burning and vehicle exhaust in particles smaller than 0.49 μm is a mixture of primary OC and SOC. The absorption of the organic species from vehicle exhaust and the formation of secondary organic particulate matter on the surface of the existing aerosols may be responsible for the fraction in particles larger than 3.0 μm. The lack of coal burning to the OC in the coarse particles is ascribed to the fewer fractions from this source and larger deviations during the estimation.
Semiquantitative Source Apportionment of EC
EC is formed as a byproduct of incomplete combustion of organic materials (Madhavi Latha and Badarinath Citation2003; Yuan et al. Citation2006). In this research, no EC was detected by the DRI 2001A analyzer following the same protocol (340°C for OC, 850°C for EC) after the particles derived from soil dust were pretreated with hydrochloric acid, indicating that no EC exists in soil dust. Thus, the EC can be considered to originate from three sources: biomass burning, coal burning, and vehicle exhaust. The contemporary EC can be inferred to originate solely from the biomass burning, and the fraction of biomass source to EC can be determined asf b=f con. The fractional contribution of the three emissions can be determined with Equations (1), (2), and (6)–(8), setting the fraction of soil dust (f s) as zero.
The carbon isotope composition of EC was affected by the OP because of the lack of water extraction prior to the thermal separation of EC. As Szidat et al. (Citation2004a) indicated that the water-soluble EC, which was considered as the OP fraction, accounted for about 30% of the EC, so the δ13C and pMC of EC (in both atmospheric and biomass burning particulate matter) were subtracted by 30% from these values of OC in the same sample and then divided by the percentage of 70% (as described by the footnote of ). Considering the discrepancy of δ13C and pMC in OC and OP, as well as the constant fraction of OP, this correction is rather rough. Thus, the source apportionment of EC is semiquantitative.
The EC sources were limited to biomass burning, coal burning, and vehicle exhaust. As shown in Figure 3, biomass burning made rather stable contributions to the EC in particles of different sizes, about 30–45%. The fossil emissions dominated EC in particles of all size ranges. Coal burning shared almost all the fossil fraction of EC in particles larger than 3.0 μm, while the contribution of vehicle exhaust concentrated in the aerosols less than 3.0 μm.
Uncertainty Analysis
The uncertainties mainly depend on whether selected carbon isotopes of particulate matter derived from the known sources are representative and reliable, as well as the variability in the reference values used in these equations. The biomass OC consists of combustion-derived OC and the noncombustion-derived OC from the biogenic emissions. In order to obtain the pMC and δ13C of the biomass OC, it is necessary to determine the proportions of combustion-derived OC and biogenic OC. In this study, both linear regression methods of OC-K+ and OC-EC were used to obtain the OC from wood burning and biogenic emissions. The estimation of OC from these two sources may bring about rather large deviations, for the slope and intercept are affected by the weather conditions, especially the extreme episodes. However, the discrepancies of pMC between the biomass burning and biogenic emissions are rather small (), resulting in small deviations in the pMC and δ13C of biomass source OC. We afford that 10% deviations in the biomass burning and biogenic emissions to the biomass OC (the proportions of biomass burning increase 10% and the biogenic OC decrease 10% in , the pMC of biomass OC will increase 0.9%, 1.6%, 1.1%, 2.1%, 2.0%, and 3.1%, while the δ13C will decrease 0.24‰, 0.50‰, 0.07‰, 0.32‰, –0.32‰, and –0.30‰ for the particles of size <0.49, 0.49–0.95, 0.95–1.5, 1.5–3.0, 3.0–7.2, and >7.2 μm. The deviations in the source apportionment results were: 0–7% for soil dust, 0–8% for biomass burning, 1–7% for biogenic emissions, 8–17% for coal burning, and 8–17% for vehicle exhaust.
δ13C was estimated by the standard values of C3 and C4 plants with their proportions. In fact, the carbon isotope composition of the same plant varies among different compounds (Rieley et al. Citation1991; Bi et al. Citation2005). The δ13C values of volatile organic species emitted by vehicle exhaust and coal burning are different from those for the particles. For instance, the δ13C of CO2 collected in the same tunnel is –31.80‰ (Liu et al. Citation2012). This indicates SOC formed by the volatile gases from vehicle exhaust will decrease the δ13C of vehicle exhaust. When –31.80 ‰ is used as the δ13C of vehicle exhaust (other parameters unchanged), the bias of coal burning and vehicle exhaust are (coal burning increase and vehicle exhaust decrease) 19%, 28%, 6%, 4%, 7%, and 6% for the particles of size <0.49, 0.49–0.95, 0.95–1.5, 1.5–3.0, 3.0–7.2, and >7.2 μm. So, the lack of coal burning to the OC in particles larger than 3.0 μm in should be a combination of the fewer fractions and larger biases brought about by the variability in the source profiles.
For EC, the OP was mistaken as part of EC in both the atmospheric aerosols and the biomass burning particles due to the defect of the two-step separation procedure. The pMC and δ13C of EC obtained by this method are combinations of the OP and “real EC.” In fact, the pMC and δ13C of OP should be between the values of OC and EC. For the EC source apportionment, the pMC and δ13C of OC were used instead of OP, because the OP cannot be separated and determined. Besides, the proportion of OP to the EC in PM10 was used from Szidat et al. (Citation2004a). The proportions of OP should vary for the particles of different sizes and origins. So, we define the source apportionment of EC semiquantitatively.
Since there are particles from long-distance transportation of foreign sources, it is theoretically difficult to obtain the precise carbon isotopes of these sources. However, most of the wind blew from the south and the southeast (http://www.wunderground.com/history/airport/ZSSS/2010/5/1/CustomHistory.html?dayend=21&monthend=5&yearend=2010&req_city=NA&req_state=NA&req_statename=NA) during the sampling period. The particles originated mainly from the Shanghai urban area, having the similar carbon isotope, so the neglect of foreign particles was reasonable and acceptable.
The fractional distributions highly depend on the carbon isotope composition of the source particles. Therefore, more precise investigations into the source profiles are needed for further study.
CONCLUSIONS
In this article, a new approach was established to estimate the fractional contributions of soil dust, biomass burning, biogenic emissions, coal burning, and vehicle exhaust to the carbonaceous particulate matter by using 14C, 13C, and linear regressions techniques. Representative and reliable pMC and δ13C values of source-derived particles were proven to be critical to this approach. Using the developed method, fractional distributions of these sources were determined for the OC and EC in size-resolved particulate matter (size ranges: <0.49, 0.49–0.95, 0.95–1.5, 1.5–3.0, 3.0–7.2, and >7.2 μm) collected in Jiading District, a suburb of Shanghai. Biomass burning was the largest contributor to the OC in the particulate matter of sizes 0.49–0.95 and 0.95–1.5 μm, accounting for 36% and 34% to the corresponding OC, respectively. For the particles larger than 0.49 μm, the contribution of biomass burning decreased with the increase in particle size. The biogenic emissions demonstrated an opposite trend to the biomass burning, sharing a fraction of 11–41%. The soil dust accounted for approximately 1–12% of the OC in particles of different sizes and made no contribution to the EC. The fractional contributions of both coal burning and vehicle exhaust to the OC and EC showed distinct different size contributions. Coal burning contributed 0–30% to the OC, and vehicle exhaust contributed 11–40% to the OC. The fraction of vehicle showed a bimodel distribution, with peaks in aerosols smaller than 0.49 μm and larger than 3.0 μm, while the coal burning concentrated in particles smaller than 3.0 μm. Due to the influence of pyrolyzed carbon, the pMC and δ13C of EC were roughly corrected, so the source apportionment of EC was semiquantitatively determined.
Acknowledgments
This work was supported by the National Science Fund for Young Scholars (11005144), the Key Program of Basic Research of Shanghai Science and Technology Commission Foundation (10JC1417200), and New Method of Determination Residence Time of Atmospheric Aerosol and Its Application (11075070).
REFERENCES
- Aelion , C. M. , Kirtland , B. C. and Stone , P. A . 1997 . Radiocarbon Assessment of Aerobic Petroleum Bioremediation in the Vadose Zone and Groundwater at an AS/SVE Site . Environ. Sci. Technol. , 31 : 3363 – 3370 .
- Andreae , M. O. 2007 . Aerosols Before Pollution. . Science , 315 : 50 – 51 .
- Andreae , M. O. and Crutzen , P. J. 1997 . Atmospheric Aerosols: Biogeochemical Sources and Role in Atmospheric Chemistry . Science , 276 : 1052 – 1058 .
- Ariya , P. A. and Amyot , M. 2003 . New Directions: The Role of Bioaerosols in Atmospheric Chemistry and Physics . Atmos. Environ. , 38 : 1231 – 1232 .
- Bennett , M. , Volckens , J. , Stanglmaier , R. , McNichol , A. P. , Ellenson , W. D. and Lewis , C. W. 2008 . Biodiesel Effects on Particulate Radiocarbon (14C) Emissions from a Diesel Engine . J. Aerosol Sci. , 39 : 667 – 678 .
- Bi , X. , Sheng , G. , Liu , X. , Li , C. and Fu , J. 2005 . Molecular and Carbon and Hydrogen Isotopic Composition of N-alkanes in Plant Leaf Waxes . Org. Geochem. , 36 : 1405 – 1417 .
- Bonn , B. and Moortgat , G. K. 2002 . New Particle FFormation during α- and -βpinene Oxidation by O3, OH and NO3, and the Influence of Water Vapour: Particle Size Distribution Studies . Atmos. Chem. Phys. , 2 : 183 – 196 .
- Cao , J. J. , Chow , J. C. , Tao , J. , Lee , S.-C. , Watson , J. G. Ho , K.-F. 2011 . Stable Carbon Isotopes in Aerosols From Chinese Cities: Influence of Fossil Fuels . Atmos. Environ. , 45 : 1359 – 1363 .
- Cao , J. J. , Lee , S. C. , Ho , K. F. , Zou , S. C. , Fung , K. Li , Y. 2004 . Spatial and Seasonal Variations of Atmospheric Organic Carbon and Elemental Carbon in Pearl River Delta Region, China . Atmos. Environ. , 38 : 4447 – 4456 .
- Chan , Y. C. , Simpson , R. W. , Mctainsh , G. H. , Vowles , P. D. , Cohen , D. D. and Bailey , G. M. 1999 . Source Apportionment of PM2.5 and PM10 Aerosols in Brisbane (Australia) by Receptor Modeling . Atmos. Evrion. , 33 : 3251 – 3268 .
- Chow , J. C. , Watson , J. G. , Crow , D. , Lowenthal , D. H. and Merrifield , T. 2001 . Comparison of IMPROVE and NIOSH Carbon Measurements . Aerosol. Sci. Tech. , 34 : 23 – 34 .
- Chow , J. C. , Watson , J. G. , Edgerton , S. A. and Vega , E. 2002 . Chemical Composition of PM2.5 and PM10 in Mexico City During Winter 1997 . Sci. Total. Environ. , 287 : 177 – 201 .
- Chu , S. H. 2005 . Stable Estimate of Primary OC/EC Ratios in the EC Tracer Method . Atmos. Environ. , 39 : 1383 – 1392 .
- Colburn , K. A. and Johnson , P. R. S. 2003 . Air Pollution Concerns Not Changed by S-PLUS Flaw . Science , 299 : 665 – 666 .
- Deines , P. 1980 . “ The Isotopic Composition of Reduced Organic Carbon ” . In Handbook of Environmental Isotope Geochemistry , Edited by: Fritz , P. and Fontes , J. C. 329 – 406 . Amsterdam : Elsevier Science .
- Ding , X. , Zheng , M. , Edgerton , E. S. , Jansen , J. J. and Wang , X. 2008 . Contemporary or Fossil Origin: Split of Estimated Secondary Organic Carbon in the Southeastern United States . Environ. Sci. Technol. , 42 : 9122 – 9128 .
- Duan , F. , Liu , X. , Yu , T. and Cachier , H. 2004 . Identification and Estimate of Biomass Burning Contribution to the Urban Aerosol Organic Carbon Concentrations in Beijing . Atmos. Environ. , 38 : 1275 – 1282 .
- Endo , M. , Yamamoto , N. , Yoshinaga , J. , Yanagisawa , Y. , Endo , O. Goto , S. 2004 . 14C Measurement for Size-Fractionated Airborne Particulate Matters . Atmos. Environ. , 38 : 6263 – 6267 .
- Feng , J. L. , Chan , C. K. , Fang , M. , Hu , M. , He , L. Y. and Tang , X. Y. 2006 . Characteristics of Organic Matters in PM2.5 in Shanghai . Chemosphere , 64 : 1393 – 1400 .
- Fu , P. Q. , Kawamura , K. , Kanaya , Y. and Wang , Z. F. 2010 . Contributions of Biogenic Volatile Organic Compounds to the Formation of Secondary Organic Aerosols over Mt. Tai, Central East China . Atmos. Evrion. , 44 : 4817 – 4826 .
- Fushimi , A. , Wagai , R. , Uchida , M. , Hasegawa , S. , Takahashi , K. Kondo , M. 2011 . Radiocarbon (14C) Diurnal Variations in Fine Particles at Sites Downwind From Tokyo, Japan, in Summer . Environ. Sci. Technol. , 45 : 6784 – 6792 .
- Gelencsér , A. , May , B. , Simpson , D. , Sánchez-Ochoa , A. , Kasper-Giebl , A. Puxbaum , H. 2007 . Source Apportionment of PM2.5 Organic Aerosol Over Europe: Primary/Secondary, Natural/Anthropogenic, and Fossil/Biogenic Origin . J. Geophys. Res. , 112 ( D23S04 )
- Gustafsson , Ö. , Kruså , M. , Zencak , Z. , Sheesley , R. J. , Granat , L. Engström , E. 2009 . Brown Clouds Over South Asia: Biomass or Fossil Fuel Combustion? . Science , 323 : 495 – 498 .
- Hildemann , L. M. , Markowski , G. R. , Jones , M. C. and Cass , G. R. 1991 . Submicrometer Aerosol Mass Distributions of Emissions From Boilers, Fireplaces, Automobiles, Diesel Trucks, and Meat-Cooking Operations . Aerosol Sci. Technol. , 14 : 138 – 152 .
- Ho , K. F. , Zhang , R. J. , Lee , S. C. , Hang Ho , S. S. , Liu , S. X. Fung , K. 2011 . Characteristics of Carbonate Carbon in PM2.5 in a Typical Semi-Arid Area of Northeastern China . Atmos. Environ. , 45 : 1268 – 1274 .
- Holmes , N. S. and Morawska , L. 2006 . A Review of Dispersion Modelling and Its Application to the Dispersion of Particles: An Overview of Different Dispersion Models Available . Atmos. Environ. , 40 : 5902 – 5928 .
- Hurt , R. H. and Gibbins , J. R. 1995 . Residual Carbon from Pulverized Coal Fired Boilers: 1. Size Distribution and Combustion Reactivity . Fuel , 74 : 471 – 480 .
- Jimenez , J. L. , Canagaratna , M. R. , Donahue , N. M. , Prevot , A. S. H. , Zhang , Q. Kroll , J. H. 2009 . Evolution of Organic Aerosols in the Atmosphere . Science , 326 : 1525 – 1529 .
- Kim , S.-H. , Chuang , J. C. , Kelly , P. B. and Clifford , A. J. 2011 . Carbon Isotopes Profiles of Human Whole Blood, Plasma, Red Blood Cells, Urine and Feces for Biological/Biomedical 14C-Accelerator Mass Spectrometry Applications . Anal. Chem. , 83 : 3312 – 3318 .
- Kleeman , M. J. , Schauer , J. J. and Cass , G. R. 2000 . Size and Composition Distribution of Fine Particulate Matter Emitted From Motor Vehicles . Environ. Sci. Technol. , 34 : 1132 – 1142 .
- Kleeman , M. J. , Robert , M. A. , Riddle , S. G. , Fine , P. M. , Hays , M. D. , Schauer , J. J. and Hannigan , M. P. 2008a . Size Distribution of Trace Organic Species Emitted from Biomass Combustion and Meat Charbroiling . Atmos. Evrion. , 42 : 3059 – 3075 .
- Kleeman , M. J. , Riddle , S. G. , Robert , M. A. and Jakober , C. A. 2008b . Lubricating Oil and Fuel Contributions To Particulate Matter Emissions from Light-Duty Gasoline and Heavy-Duty Diesel Vehicles . Environ. Sci. Technol. , 42 : 235 – 242 .
- Kleeman , M. J. , Riddle , S. G. , Robert , M. A. , Jakober , C. A. , Fine , P. M. , Hays , M. D. , Schauer , J. J. and Hannigan , M. P. 2009 . Source Apportionment of Fine (PM1.8) and Ultrafine (PM0.1) Airborne Particulate Matter during a Severe Winter Pollution Episode . Environ. Sci. Technol. , 43 : 272 – 279 .
- Koulouri , E. , Saarikoski , S. , Theodosi , C. , Markaki , Z. , Gerasopoulos , E. Kouvarakis , G. 2008 . Chemical Composition and Sources of Fine and Coarse Aerosol Particles in the Eastern Mediterranean . Atmos. Environ. , 42 : 6542 – 6550 .
- Lemire , K. R. , Allen , D. T. , Klouda , G. A. and Lewis , C. W. 2002 . Fine Particulate Matter Source Attribution for Southeast Texas using 14C/13C ratios . J. Geophys. Res. , 107 : 4613 – 4619 .
- Levin , I. and Kromer , B. 1997 . Twenty Years of Atmospheric 14CO2 Observations at Schauinslandstation, Germany . Radiocarbon , 39 : 205 – 218 .
- Levin , I. and Kromer , B. 2006 . The Tropospheric 14CO2 Level in Mid Latitudes of the Northern Hemisphere (1959–2003) . Radiocarbon , 46 ( 3 ) : 1261 – 1271 .
- Lichtfouse , E. , Lichtfouse , M. and Jaffrézic , A. 2002 . δ13C Values of Grasses as a Novel Indicator of Pollution by Fossil-Fuel-Derived Greenhouse Gas CO2 in Urban Areas . Environ. Sci. Technol. , 37 : 87 – 89 .
- Liu , W. , Moriizumi , J. , Yamazawa , H. and Iida , T. 2006 . Depth Profiles of Radiocarbon and Carbon Isotopic Compositions of Organic Matter and CO2 in a Forest Soil . J. Environ. Radioact. , 90 : 210 – 223 .
- Liu , W. , Wei , N. N. , Wang , G. H. , Yao , J. , Zeng , Y. S. Fan , X. B. 2012 . Quantitative Estimation Source of Urban Atmospheric CO2 by Carbon Isotope Composition . Environ. Sci. , 33 : 1041 – 1049 . in Chinese
- Madhavi Latha , K. and Badarinath , K. V. S. 2003 . Black Carbon Aerosols Over Tropical Urban Environment – A Case Study . Atmos. Res. , 69 : 125 – 133 .
- May , A. A. , Saleh , R. , Hennigan , C. J. , Donahue , N. M. and Robinson , A. L. 2012 . Volatility of Organic Molecular Markers Used for Source Apportionment Analysis: Measurements and Implications for Atmospheric Lifetime . Environ. Sci. Technol. , [Epub ahead of print].
- McElroy , M. W. , Carr , R. C. , Ensor , D. S. and Markowski , G. R. 1982 . Size Distribution of Fine Particles From Coal Combustion . Science , 215 : 13 – 19 .
- Moriizumi , J. , Goto , A. and Iida , T. 2004 . A New Extraction Technique for Atmospheric 14CO and Its Application . Nucl. Instrum. Methods Phys. Res., Sect. B , 223–224 : 511 – 515 .
- Nel , A. 2005 . Air Pollution-Related Illness: Effects of Particles . Science , 308 : 804 – 806 .
- Pandis , S. N. , Wexler , A. S. and Seinfeld , J. H. 1993 . Secondary Organic Aerosol Formation and Transport – II. Predicting the Ambient Secondary Organic Aerosol Size Distribution . Atmos. Environ. , 27 : 2403 – 2416 .
- Petsch , S. T. , Eglinton , T. I. and Edwards , K. J. 2001 . 14C-Dead Living Biomass: Evidence for Microbial Assimilation of Ancient Organic Carbon During Shale Weathering . Science , 292 : 1127 – 1131 .
- Reddy , C. M. , Pearson , A. , Xu , L. , McNichol , A. P. , Benner , B. A. Wise , S. A. 2002 . Radiocarbon as a Tool to Apportion the Sources of Polycyclic Aromatic Hydrocarbons and Black Carbon in Environmental Samples . Environ. Sci. Technol. , 36 : 1774 – 1782 .
- Rethemeyer , J. , Kramer , C. , Gleixner , G. and Wiesenberg , G. L. B. 2004 . Complexity of Soil Organic Matter: AMS 14C Analysis of Soil Lipid Fractions and Individual Compounds . Radiocarbon , 46 : 465 – 473 .
- Rieley , G. , Collier , R. J. , Jones , D. M. , Eglinton , G. , Eakin , P. A. and Fallick , A. E. 1991 . Sources of Sedimentary Lipids Deduced From Stable Carbon Isotope Analyses of Individual Compounds . Nature , 352 : 425 – 427 .
- Rosenfeld , D. , Lohmann , U. , Raga , G. B. , O’Dowd , C. D. , Kulmala , M. Fuzzi , S. 2008 . Flood or Drought: How Do Aerosols Affect Precipitation? . Science , 321 : 1309 – 1313 .
- Rubli , S. , Belevi , H. and Baccini , P. 2003 . Optimizing Municipal Solid Waste Combustion Through Organic and Elemental Carbon as Indicators . Environ. Sci. Technol. , 37 : 1025 – 1030 .
- Sakai , H. and Matsuhisa , Y. 1996) . Stable Isotope Geochemistry , 182 – 185 . Tokyo : University of Tokyo Press .
- Saylor , R. D. , Edgerton , E. S. and Hartsell , B. E. 2006 . Linear Regression Techniques for Use in the EC Tracer Method of Secondary Organic Aerosol Estimation . Atmos. Environ. , 40 : 7546 – 7556 .
- Schichtel , B. A. , Malm , W. C. , Bench , G. , Fallon , S. , McDade , C. E. Chow , J. C. 2008 . Fossil and Contemporary Fine Particulate Carbon Fractions at 12 Rural and Urban Sites in the United States . J. Geophys. Res. , : 113 D02311
- Schwier , A. N. , Sareen , N. , Mitroo , D. , Shapiro , E. L. and McNeill , V. F. 2010 . Glyoxal-Methylglyoxal Cross-Reactions in Secondary Organic Aerosol Formation . Environ. Sci. Technol. , 44 : 6174 – 6182 .
- Silva , P. J. , Liu , D.-Y. , Noble , C. A. and Prather , K. A. 1999 . Size and Chemical Characterization of Individual Particles Resulting From Biomass Burning of Local Southern California Species . Environ. Sci. Technol. , 33 : 3068 – 3076 .
- Szidat , S. , Jenk , T. M. , Gäggeler , H. W. , Synal , H. A. , Fisseha , R. Baltensperger , U. 2004a . Source Apportionment of Aerosols by 14C Measurement in Different Carbonaceous Particle Fractions . Radiocarbon. , 46 : 475 – 484 .
- Szidat , S. , Jenk , T. M. , Gäggeler , H. W. , Synal , H. A. , Hajdas , I. Bonani , G. 2004b . THEODORE, a Two-Step Heating System for the EC/OC Determination of Radiocarbon (14C) in the Environment . Nucl. Instrum. Methods Phys. Res., Sect. B , 223–224 : 829 – 836 .
- Tang , G. J. 2001 . δ13C Characteristics of Carboniferous Coal in North China and Its Palaeogeographic Implications. Political Scholars Anthology of Peking University , Peking : Peking University . in Chinese
- Turpin , B. J. and Huntzicker , J. J. 1991 . Secondary Formation of Organic Aerosol in the Los Angeles Basin: A Descriptive Analysis of Organic and Elemental Carbon Concentrations . Atmos. Environ. , 25 : 207 – 215 .
- Wang , G. H. , Zeng , Y. S. , Yao , J. , Qian , Y. , Huang , Y. Liu , K. 2012 . Source Apportionment of Atmospheric Carbonaceous Particulate Matter Based on the Radiocarbon . J Radioanal. Nucl. Chem. , DOI: 10.1007/s10961-DR-2245-5
- Wang , Y. Q. , Zhang , X. Y. , Arimoto , R. , Cao , J. J. and Shen , Z. X. 2005 . Characteristics of Carbonate Content and Carbon and Oxygen Isotopic Composition of Northern China Soil and Dust Aerosol and Its Application to Tracing Dust Sources . Atmos. Environ. , 39 : 2631 – 2642 .
- Widory , D. 2006 . Combustibles, Fuels and Their Combustion Products: A View Through Carbon Isotopes . Combust. Theor. Model. , 10 : 831 – 841 .
- Xi , X. , Ding , X. , Fu , D. , Zhou , L. and Liu , K. 2011 . Regional Δ14C Patterns and Fossil Fuel Derived CO2 Distribution in the Beijing Area Using Annual Plants . Chin. Sci. Bull. , 56 : 1721 – 1726 .
- Yamamoto , N. , Muramoto , A. , Yoshinaga , J. , Shibata , K. , Endo , M. Endo , O. 2007 . Comparison of Carbonaceous Aerosols in Tokyo Before and After Implementation of Diesel Exhaust Restrictions . Environ. Sci. Technol. , 41 : 6357 – 6362 .
- Ye , B. M. , Ji , X. L. , Yang , H. Z. , Yao , X. H. , Chan , C. K. Cadle , S. H. 2003 . Concentration and Chemical Composition of PM2.5 in Shanghai or a 1-Year Period . Atmos. Environ. , 37 : 499 – 510 .
- Yuan , Z. B. , Yu , J. Z. , Lau , A. K. H. , Louie , P. K. K. and Fung , J. C. H. 2006 . Application of Positive Matrix Factorization in Estimating Aerosol Secondary Organic Carbon in Hong Kong and Its Relationship With Secondary Sulfate . Atmos. Chem. Phys. , 6 : 25 – 34 .
- Yue , W. , Li , X. , Liu , J. , Li , Y. , Yu , X. Deng , B. 2006 . Characterization of PM2.5 in the Ambient Air of Shanghai City by Analyzing Individual Particles . Sci. Total Environ. , 368 : 916 – 925 .
- Zeng , Y. S. , Wang , G. H. , Yao , J. , Li , Y. L. , Huang , Y. Yuan , N. 2012 . Characteristics of Carbonaceous, Particulate Matter in Shanghai . Acta Sci.Circumst. , (in press).
- Zhang , X. Y. , Gong , S. L. , Shen , Z. X. , Mei , F. M. , Xi , X. X. Liu , L. C. 2003 . Characterization of Soil Dust Aerosol in China and Its Transport and Distribution During 2001 ACE-Asia: 1. Network Observations . J. Geophys. Res. , 108 : 4261
- Zhao , T. L. , Gong , S. L. , Zhang , X. Y. and McKendry , I. G. 2003 . Modeled Size-Segregated Wet and Dry Deposition Budgets of Soil Dust Aerosol During ACE-Asia 2001: Implications for Trans-Pacific Transport . J. Geophys. Res. , 108 : 8665