Abstract
The importance of the aerosol mode for transmission of influenza is unknown. Understanding the role of aerosols is essential to developing public health interventions such as the use of surgical masks as a source control to prevent the release of infectious aerosols. Little information is available on the number and size of particles generated by infected persons, which is partly due to the limitations of conventional air samplers, which do not efficiently capture fine particles or maintain microorganism viability. We designed and built a new sampler, called the G-II, that collects exhaled-breath particles that can be used in infectivity analyses. The G-II allows test subjects to perform various respiratory maneuvers (i.e., tidal breathing, coughing, and talking) and allows subjects to wear a mask or respirator during testing. A conventional slit impactor collects particles greater than 5.0 μm. Condensation of water vapor is used to grow remaining particles, including fine particles, to a size large enough to be efficiently collected by a 1.0 μm slit impactor and be deposited into a buffer-containing collector. We evaluated the G-II for fine particle collection efficiency with inert particle aerosols and evaluated infective virus collection using influenza A virus aerosols. Testing results showed greater than 85% collection efficiency for particles greater than 50 nm and influenza virus collection comparable with a reference SKC BioSampler®. The new design will enable determination of exhaled infectious virus generation rate and evaluate control strategies such as wearing a surgical-type mask to prevent the release of viruses from infected persons.
Copyright 2013 American Association for Aerosol Research
INTRODUCTION
There is uncertainty regarding the relative importance of the aerosol mode for transmission of influenza. However, understanding the role of aerosols is essential to developing public health interventions and protective measures for health care workers that will be effective early in pandemics when vaccines are not available (IOM Citation2009). One approach to identifying the importance of aerosols is to develop mathematical models of transmission on a micro scale. Unfortunately, that approach is plagued by a lack of data from which to confidently specify input parameters, resulting in vast ranges of uncertainty (Nicas and Jones Citation2009). A major source of uncertainty is the unknown size distribution and the rate of release of droplets containing infectious viruses from infected persons.
Infectious aerosol collection during respiratory maneuvers has previously been studied to a limited degree (Fennelly et al. Citation2004; Fabian et al. Citation2008; Lindsley et al. Citation2010; Fennelly et al. Citation2012). In pulmonary tuberculosis patients, Fennelly et al. (Citation2004) used the Cough Aerosol Sampling System (CASS) to measure respirable, infectious mycobacterial aerosols and demonstrated that quantification of cough-generated aerosols was feasible. Lindsley et al. used a spirometer to collect cough-associated aerosols from subjects infected with influenza. Subjects coughed into a spirometer, which was then sampled for influenza virus RNA. Much of the influenza RNA detected from these samples was in respirable-sized particles. Fabian et al. suggest that influenza RNA may be contained in fine particles during tidal breathing of subjects infected with influenza. Thus, influenza virus is likely associated with respirable aerosols, which can be measured in exhaled breath.
If the aerosol route was shown to be a major mode of influenza transmission, a second problem would arise because the use of appropriate personal respiratory protection in health care would present tremendous expense and logistical hurdles. Thus, alternative approaches to health care worker protection would be needed. From a traditional occupational hygiene perspective, engineering controls to contain or capture the hazardous exposure at the source would be preferable to personal protective equipment for the workers (Rose Citation2003). Although a variety of intervention options could be implemented if aerosols were shown to be an important route of transmission, the use of surgical masks by infected persons is a potential, simple approach to control transmission at the source. At the point of exit from the respiratory tract, respiratory droplets will be at their largest size, since they have not had the opportunity to lose water by evaporation, and are moving at their highest velocities prior to deceleration into room air. Thus, the inertia of these particles will be the highest (compared to after exit from the respiratory tract) and contributes to aerosol removal by masks worn on the aerosol source. The use of masks is currently included by the Centers for Disease Control and Prevention as an infection control strategy for seasonal influenza in health care centers (CDC Citation2010) and was part of the World Health Organization interim guidance statement during the (H1N1) 2009 pandemic (WHO Citation2009).
Mask testing has been done previously with artificially generated aerosols using mannequins (Diaz and Smaldone Citation2008; Noti et al. Citation2012). Diaz and Smaldone (Citation2008), using chamber testing with inert aerosols and mannequins, conditionally concluded that masks worn at the source achieved far greater levels of protection than any mask on the receiver and that mask filtration at the source or receiver did not play a significant role in reducing exposure. However, such studies do not reflect the real world conditions of mask use with respect to mask fit, particle size and number, breathing patterns, droplet composition, and so forth. Johnson et al. (Citation2009) used subjects infected with influenza to show N-95 respirators and surgical masks were effective in preventing deposition of polymerase chain reaction (PCR) detectable influenza virus from infected sources onto petri dishes. However, the findings of the study were limited since the methods used did not allow evaluation of infectivity, were biased toward detecting only large droplets and did not evaluate leakage around the sides of the masks (Johnson et al. Citation2009).
Simply testing aerosols by reverse transcription, quantitative-polymerase chain reaction (RT-qPCR) for detection of viral nucleic acid would not be sufficient to demonstrate that the viruses in fine particles remain infectious. Given the extensive debate in the literature (Tellier Citation2006; Brankston et al. Citation2007) and the likelihood that a large percentage of viral copies detected by molecular methods are defective (Fabian et al. Citation2009a; Noti et al. Citation2012), it would be important for new studies to quantify infectious virus and not merely measure the total viral RNA copy numbers.
Our goal was to design and evaluate the performance of an exhaled-breath sampling device that can characterize infectious influenza aerosols emitted from infected persons who are wearing masks and performing various respiratory maneuvers (i.e., tidal breathing, talking, and coughing). Based on expected low exhaled-breath generation of influenza virus (Fabian et al. Citation2008), the sensitivity of the device requires maximization by collecting all exhaled breath and allowance for extended sampling times (30 min). To capture the majority of exhaled breath, the sampling rate should exceed peak flow during tidal breathing and the inlet of the collection device should offer capacitance during peak flow/short duration respiratory maneuvers such as cough. The interface between the device and the test patient must maintain comfort with a subject who is suffering from the flu. Considering that “naked” influenza virus is about 120 nm in size, the device must efficiently collect submicron size particles. Since virus-containing droplets expelled from the respiratory tract are likely to range widely in size, and since particle size will impact mask efficiency (in addition to other airborne transmission factors), the aerosol characterization needs to discriminate between “coarse” and “fine” particles and be able to determine whether fine particles represent infectious virus. In this paper, we describe a device designed to meet these requirements and evaluate its performance.
DESCRIPTION OF THE SAMPLING SYSTEM
The device was designed, built, and is shown in . It is called the Gesundheit II (G-II) to acknowledge the pioneering work of Knight and colleagues on whose cough collection device the word Gesundheit can be seen in a photograph published with their work (Gerone et al. Citation1966).
FIG. 1 Diagram of G-II exhaled breath bioaerosol collection system (U = upstream sampling location; D = downstream sampling location; T = temperature sensor; M = Magnehelic® pressure gauge; RH/T = relative humidity and temperature sensor; V = voltage controller; F = flow controller).
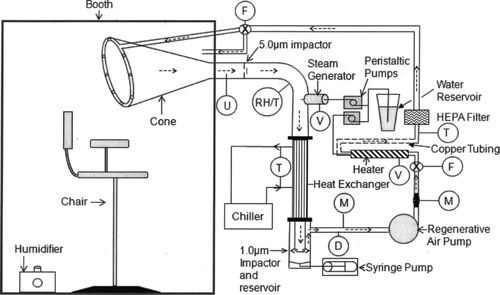
Inlet
We loosely based the design of the sampling inlet of the G-II on a chamber for the collection of sneeze and cough described by Gerone et al. Citation1966. Test subjects sit in a booth supplied with HEPA-filtered air maintained at approximately 80% relative humidity (RH) and face into a truncated cone-shaped inlet. The cone draws in air at a total flow rate of 130 L/min. Humidified air is supplied to the perimeter of the cone (approximately 60% of total flow) to provide a sheath flow along the cone walls and minimize particle loss. The remainder of the air is drawn into the cone around the subjects’ head, much like a capture type ventilation hood, to minimize fugitive emissions from the cone. The design of the cone allows test subjects to comfortably perform various breathing maneuvers (i.e., tidal breathing, coughing, and talking) and also allows subjects to wear a mask or respirator during testing. The cone design also allows test subjects to quickly remove their head from the device and avoids imparting a claustrophobic effect on those who are ill with influenza.
“Coarse” Fraction Collector
After entering the cone, exhaled breath travels through a slit impactor. The impactor was designed to collect particles greater than 5.0 μm aerodynamic diameter (note that all particle sizes herein are expressed as aerodynamic diameter unless otherwise noted) and is fitted with a Teflon® impaction substrate. After sample collection, the Teflon substrate is removed and rinsed. The rinsate is then analyzed by RT-qPCR to determine the amount of influenza virus RNA associated with particles greater than 5.0 μm.
Condensation Growth Unit
The condensation growth unit design was based on components of the Harvard Ultrafine Concentrated Ambient Particle System (HUCAPS) (Gupta et al. Citation2004). The condensation growth unit consists of two components: saturator and condenser (supersaturator). The saturator is a 7.6 cm diameter, 24.5 cm long pipe fitted with a steam injection tube. The steam injector tube is perpendicular to the tangent of the pipe circumference. Steam is mixed with air as it enters the saturator. Steam is generated by pumping water (approximately 2 mL/min) through a heated tube fitted with a high temperature cartridge heater. The steam generation rate is controlled by adjusting the pump flow rate and cartridge heater voltage. The sample air exits the saturator at close to saturation conditions. Subsequently, the saturated air enters the condenser where it is cooled and becomes super saturated. The condenser is a shell and tube, coolant to air heat exchanger (SSCF, ITT Standard, Buffalo, NY, USA) in which the air flows through the tubes countercurrent to the coolant in the outer shell. The coolant is circulated and maintained at 0°C using an external refrigeration unit (chiller) (VWR Chiller 1173PD, VWR, West Chester, PA, USA) containing a glycol–water mixture.
“Fine” Fraction Collector
Particles grown by condensation are subsequently drawn through a 1.0 μm slit impactor. The impactor is sealed into a reservoir into which particles and condensate are collected (). There is ample room between the bottom of the impactor and the bottom of the reservoir to allow condensate to collect without interfering with the operation of the impactor. The outlet from the reservoir, which leads to a vacuum pump, is located at the top of the reservoir to prevent re-aerosolization of condensate. The bottom of the reservoir is fitted with a valve that allows addition of buffer solution during sample collection and extraction of collected liquid.
System Exhaust and Supply Air
Air is recirculated through the G-II system using a regenerative pump. The vacuum side of the pump draws air out of the fine fraction collector and then the displacement side of the pump resupplies the air to the G-II cone and sampling booth. Because air removed from the fine fraction collector has very low moisture content, the supply air is rehumidified prior to flow into the inlet cone and the booth. The supply air is heated to about 48°C to facilitate evaporation of water, which is dripped into an evaporation chamber. The air is then cooled to approximately 28°C as it passes through copper tubing routed to a HEPA filter prior to returning to the booth. Supplemental humidity is added to the booth to maintain approximately 80% RH via 2 Vicks V790-N “Germ Free” warm mist humidifiers (Kaz USA Inc., Southborough, MA, USA).
OPERATIONAL EVALUATION
The conventional slit inertial impactor design used in the coarse fraction collector has been well characterized (Marple and Willeke Citation1976). Here, we use fluorescent polystyrene latex spheres (PSLs) to demonstrate that particles introduced into the cone are efficiently captured by the coarse and fine fraction collection stages of the G-II. Submicron particle collection efficiency was evaluated using either nebulized (polydisperse) ammonium sulfate aerosols or influenza virus aerosols. Experiments with ammonium sulfate allowed us to characterize submicron particle collection efficiency as a function of particle size, while experiments with influenza virus evaluated the ability of the G-II to maintain virus infectivity during the sampling process. Tests of virus recovery were made by molecular assay and culture of fine particle virus aerosol captured in the reservoir and compared with a reference sampler. Because previous work (Fabian et al. Citation2009a) suggested that recovery of infectious virus from the Teflon substrate would be low relative to total recovery for molecular assay, no attempt was made to culture from the Teflon substrate.
Polystyrene Latex Sphere Collection Efficiency Measurements
Compressed N2 was released to aerosolize solutions containing deionized water (DI) and PSL fluorescent spheres (Thermo Scientific, Barrington, IL, USA). A six-jet, Collison nebulizer (BGI Inc., Waltham, MA, USA) was used for aerosolization of spheres with dp = 1.0 μm, while a Hudson UpDraft nebulizer (Hudson, Research Triangle Park, NC, USA) was used for spheres with dp = 4.8 and 9.9 μm. Aerosols were passed through a diffusion dryer prior to being directed to either the G-II or a liquid impinger. In order to count the total number of PSL spheres aerosolized and released into the G-II, the aerosol output was also collected with a liquid impinger. Based on the sampler collection efficiency, the SKC BioSampler (SKC Inc., Eighty Four, PA, USA) was used for 1.0 μm PSL (Macher Citation1997), while the AGI-30 (ACE Glass Inc., Vineland, NJ, USA) was used for 4.8 and 9.9 μm PSL (Lin et al. Citation1999). DI water was used as the collection medium of both impingers.
Collection efficiency of the G-II was characterized by comparing the number of PSL spheres collected by the coarse fraction collector or by the fine fraction collector to the total number of particles that were aerosolized as estimated by collection in the impingers (EquationEquations (1) and Equation(2), respectively):
Collection efficiency of coarse fraction collector:
When collection of the PSL was complete, the impactor substrate was removed, placed in a vial containing DI water, and then vortexed for 1 min to remove deposited PSL particles. Condensate liquid from the fine fraction collector was removed directly from the reservoir with a syringe and transferred to a 50 mL centrifuge tube.
All PSL samples were analyzed using a BD FACSCanto II flow cytometer (BD Biosciences, San Hose, CA, USA). PSL sphere size was determined as described by Sakaguchi and Ekhara (Citation2011) with a reported measurement uncertainty of 4.4%, while counting was performed indirectly based on the total number of particles counted by adding a known concentration of counting beads into the sample.
Prior to counting and sizing, 50 mL of PSL samples were concentrated by spinning at 3000 rpm for 60 min using a Heraeus Instruments Megafuge 2.0R (Heraeus Instruments, South Bend, IN, USA). After spinning, the supernatant was removed for a final volume of 1 mL and 1 μL of counting beads, which were added to the sample (5.2 × 104 particles/μL). For a subset of samples, the supernatant was also analyzed by the flow cytometry to ensure efficient concentration of the PSL samples. We calibrated the flow cytometer with 1.0, 4.8, and 9.9 μm PSL spheres and counting beads and counted a total of 20,000 particles from each sample. The area of detection, including side and forward scatter, for each respective size was marked and used as a calibration surface to detect the presence of the PSL beads, counting beads, and potential agglomeration in each of the samples.
Sulfate Aerosol Collection Efficiency Measurements
The particle collection efficiency of the G-II was evaluated with sulfate aerosol produced using a high-output extended aerosol respiratory therapy (HEART®) (Westmed, Tucson, AZ, USA) nebulizer containing 3.00 mM ammonium sulfate. The aerosol from the nebulizer was mixed with dry air in a 7.5 L mixing chamber and subsequently delivered into the cone of the G-II.
Ammonium sulfate aerosol was collected using 47 mm, 2.0 μm pore size, TefloTM filters (PALL Corporation, Ann Arbor, MI, USA) housed in a single-stage PFA-Teflon filter assembly (Savillex, Minnetonka, MN, USA). An upstream sample was collected at 2 lpm prior to aerosol delivery to the cone and a downstream sample was collected at 5 lpm at the outlet port of the collection reservoir. Upstream and downstream samples (in duplicate) were collected concurrently over a 15-min period. The hydrophobic PTFE Teflon membrane filters were wet with 0.15 mL of absolute ethanol and extracted with 5 mL of 0.0015 N NaOH, and analyzed for sulfate using a DX-120 ion chromatograph (Dionex, Bannockburn, IL, USA). The air concentration of sulfate, both upstream and downstream, was determined from the volume of air collected and the mass of sulfate. Collection efficiency was calculated by comparing upstream and downstream concentrations.
At the same upstream and downstream ports used for filter samples, a scanning mobility particle analyzer (SMPS) (Model 3080/3785, TSI Inc., Shoreview, MN, USA) was used to measure the particle number concentration as a function of mobility diameter for particle sizes between 0.025 and 0.750 μm with 110 size bins. The DMA scan time was set to 180 s and ten successive scans were collected upstream and downstream from the G-II for a total of five upstream and downstream sets. The collection efficiency was calculated for each mobility diameter size bin for successive upstream and downstream scans and the mean collection efficiency for each size bin was computed from the five upstream and downstream data pairs. The mobility diameter was converted to aerodynamic diameter using the TSI Data Merge Software Module (TSI Inc., Shoreview, MN, USA).
Submicron Particle Losses
Submicron particle losses were evaluated using naturally occurring (room air) submicron particles. Upstream measurements were made at the entrance to the G-II within the cone and downstream measurements were made after the 1.0 μm impactor at the exit from the reservoir. Samples (n = 12) were integrated over 10 s with a 1-min interval between upstream and downstream sample sets. Submicron particle count concentrations were measured using a P-Trak® Ultrafine Particle Counter 8525 (TSI Inc., Shoreview, IL, USA).
Influenza Aerosol Testing
The G-II was compared to a commercially available sampler, the SKC BioSampler (SKC Inc., Eighty Four, PA, USA), to evaluate maintenance of virus infectivity. Influenza aerosols were generated by adding 0.025 mL of undiluted Influenza A/PR/8/34 H1N1 (Advanced Biotechnologies Inc., Columbia, MD, USA) and 25 mL of virus buffer (Dulbecco's phosphate buffered saline with calcium and magnesium [Hyclone Laboratories, Logan, UT, USA] containing 0.1% bovine serum albumin [SeraCare, Milford, MA, USA]) into a HEART nebulizer. The nebulizer output was mixed with dry air in a 7.5 L chamber prior to delivery to either the BioSampler or the G-II. The HEART nebulizer, mixing chamber, and BioSampler were housed within a Class IIA biological safety cabinet, while the G-II was housed in a negative pressure room with exhaust through a HEPA filter. Polyethylene sample delivery lines to the BioSampler and G-II were matched in terms of diameter and length. The reservoir of the BioSampler was filled with 20 mL of virus buffer prior to sampling and the volume remeasured after sampling. Concentrated virus buffer (10×) was pumped into the G-II reservoir with a syringe pump at approximately 4.0 mL/min at the start of sampling. The upper torso and head of a mannequin were positioned at the cone entrance of the G-II to simulate the presence of a test subject. The polyethylene tubing delivering the aerosol protruded from the mannequins’ mouth about 2 cm. Samples of the influenza aerosol were collected successively with either the G-II or BioSampler over 15 min periods. Experiments were conducted on three separate days with the order of samplers alternated each day.
Infectivity Analysis
Samples were analyzed for infectivity using a focus reduction assay, which has been described elsewhere (Rudnick et al. Citation2009). Briefly, triplicate wells on a 96-well plate containing monolayers of Madin Darby Canine Kidney (MDCK) cells (ATTC # CCL-34) were infected with 50 μL of collection buffer from each sampler and allowed to incubate for approximately 8 h. The resulting infected cells containing influenza A nucleoproteins were labeled with mouse monoclonal antibody [AA5H] to influenza A virus nucleoprotein (Abcam, Cambridge, MA, USA) and subsequently labeled with rhodamine-labeled goat anti-mouse IgG (Jackson ImmunoResearch Laboratories, West Grove, PA, USA). The number of cells having a resulting fluorescent foci, which are referred to as fluorescent focus units (FFU), was then counted at 200× total power using an Olympus CKX-41 inverted fluorescent microscope (Olympus, Center Valley, PA, USA). Each well was scanned in a standard pattern with ten fields chosen at random for counting (about 30% of the well). For samples with less than two FFU per viewing field, the entire well was counted. Counts were volume adjusted based on the volume of collection buffer in each sampler.
RNA Analysis
RNA extraction in Trizol–chloroform, reverse transcription, and quantitative PCR were performed as previously described (Fabian et al. Citation2009a; Fabian et al. Citation2009b). Quantitative PCR was performed using an Applied Biosystems Prism 7300 detection system (Foster City, CA, USA). Duplicate samples were analyzed using influenza A primers and probe as previously described (van Elden et al. Citation2001). A standard curve was constructed in each assay with cDNA extracted from a stock of influenza A/PR/8/34 with a concentration of 3.0 × 1011 virus particles per milliliter. Results are expressed as the total number of virus particles by reference to the standard curve and are rounded to the closest integer value.
RESULTS AND DISCUSSION
Collection efficiency of the combined coarse and fine fraction collectors for collecting 1.0–9.9 μm particles was evaluated with fluorescent PSL. Efficiency of the fine fraction collector for submicron particles was evaluated with sulfate aerosols. Influenza virus aerosols were generated to evaluate preservation of virus infectivity by the fine fraction collector.
A theoretical efficiency curve for the 5.0 μm impactor was computed based on methods of Marple and Willeke (Citation1976) and is shown in . These calculations predict that particles with dp < 4.6 μm will not be collected, while particles with dp > 7.0 μm will be collected with 100% efficiency. Additionally, shows the collection efficiency (with reference to impinger collection) of the coarse fraction collector (5.0 μm impactor) and the fine fraction collector (saturator/condenser and 1.0 μm impactor). The results obtained with PSL spheres agree well with the theoretical predictions. We recovered 91% (SD = 8%) of 1.0 μm spheres from the fine collector stage and 1% (SD = 1%) from the coarse fraction collector. For dp = 4.8 μm PSL spheres, we recovered 37.7% (SD = 6%) from the coarse stage and 51% (SD = 7%) in the fine stage's reservoir. Ninety-nine percent (SD = 1%) of the PSL spheres with dp = 9.9 μm were collected by the coarse stage's impactor, and none of these PSL spheres were detected in the samples from the fine stage's reservoir. Thus, our experimental results are in agreement with the predicted collection efficiency.
FIG. 2 G-II collection efficiency for 1.0, 4.8, and 9.9 μm PSL spheres as measured by flow cytometry by comparison with liquid impingement (error bars = SD) and theoretically predicted efficiency (computation based on Marple and Willike [Citation1976]: S/W = 1, Re = 8242, d 50 = 5.0 μm) for the coarse fraction collector.
![FIG. 2 G-II collection efficiency for 1.0, 4.8, and 9.9 μm PSL spheres as measured by flow cytometry by comparison with liquid impingement (error bars = SD) and theoretically predicted efficiency (computation based on Marple and Willike [Citation1976]: S/W = 1, Re = 8242, d 50 = 5.0 μm) for the coarse fraction collector.](/cms/asset/fe685e6b-ee37-44cd-b6a6-0d515cef1c98/uast_a_762973_o_f0002g.gif)
FIG. 3 Sulfate aerosol collection efficiency of the G-II, as a function of aerodynamic diameter, measured by DMA/CPC.
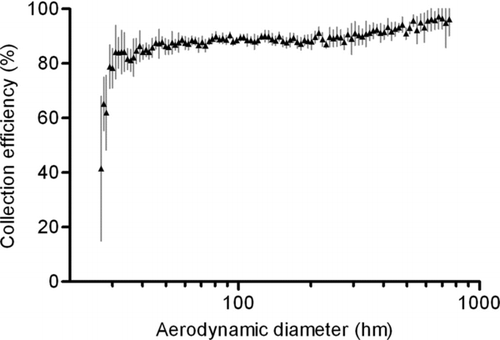
Filter testing using upstream and downstream filter collection of sulfate aerosol showed a collection efficiency of 96%. Further testing was done using the DMA/CPC to determine collection efficiency as a function of aerodynamic particle size. Particles were measured over a range from 0.026 to 0.750 μm aerodynamic equivalent diameter. As shown in , the collection efficiency exceeds 85% for particles greater than about 50 μm and exceeds 90% for particles greater than 300 μm. The nominal size of “naked” influenza virus is considered to be about 80–120 nm (Stanley Citation1944). As such, the G-II would be expected to efficiently collect influenza at its smallest size. Since influenza virus is likely exhaled in droplets containing salts and proteins associated with the respiratory tract, particle sizes may exceed this size range after evaporation (Morawska Citation2006) and be collected at higher efficiencies.
To evaluate submicron-sized particle losses in the collection system, the G-II was operated without the addition of humidity to the air and with the chiller turned off. Under these conditions, there should have been no particle growth or removal of particles less than 1.0 μm. Based on CPC counts using the P-Trak, there were no statistically significant differences between upstream and downstream concentrations of submicron particles (mean upstream = 944 #/cc, = SD 28.9; mean downstream = 937 #/cc, SD 32.7; p = 0.56). Potential particle losses within the G-II prior to collection by the impactor were not directly accounted for in the sulfate aerosol testing experiments, but were likely minor based on the P-Trak and the PSL experiments.
Using impaction or centrifugation to remove submicron particles from an airstream would require very high velocity airstreams and large/noisy pumps to accommodate the associated high-pressure drops. To circumvent these issues, submicron particles were grown to supermicron size to accommodate removal via impaction with moderate pressure drop across the orifice. The technology used to collect the submicron particles, impaction following growth by condensation, was used by Gupta et al. (Citation2004) as part of the HUCAPS (Gupta et al. Citation2004) and by Kidwell and Ondov (Citation2001) as part of the Semicontinuous Elements in Air Sampler (SEAS) (Kidwell and Ondov Citation2001). The HUCAPS system was designed to collect large volumes of air, concentrate ultrafine particles via condensation and virtual impaction, and return the concentrated aerosol to its original size distribution. For the purpose of our research, we did not need to return the aerosol to its original size; rather, our goal was to grow the particles to allow easy removal. Using condensation to grow particles to greater than 1.0 μm allowed efficient removal of particles down to 50 nm with a moderate pressure drop across the orifice (<10″ H2O). Similarly, Kidwell and Ondov (Citation2001) developed the SEAS to collect ambient aerosols for chemical analysis using particle growth via condensation. After growth through condensation, the SEAS concentrates aerosols using a virtual impactor and collects particles in a liquid slurry with a conventional impactor. However, the collection efficiency of the SEAS was reported as only 40% for particles less than 0.5 μm. Orsini et al. (Citation2008) developed a sampler using a similar particle growth strategy for use with viruses. Their particle-into-liquid sampler (PILS) combines the air sample with a turbulent flow of steam, rapid adiabatic cooling of the saturated air by the sample air, and collection of grown particles in a wet-walled cyclone (Orsini et al. Citation2008). This system was designed to have a 16 lpm flow rate and maintained an average collection efficiency of 90% down to 50 nm. However, a flow rate of 16 lpm would be insufficient to capture the majority of exhaled breath during tidal breathing and even less during peak exhaled events such as coughing. As a result, virus detection sensitivity would be severely limited using the PILS system for our research aims.
Total virus copy number was determined by RT-qPCR. Since RT-qPCR relies on amplifying nucleic acid, both infective viruses and inactivated virus are measured (referred to as total virus). The infectivity data measure viruses that are able to infect MDCK mammalian cells (used in the assay) after being collected by the respective samplers. The total virus copy number by RT-qPCR and culturable virus per sample collected by the G-II (mean total copy number/sample = 1.8 × 108, SD = 3.3 × 107; mean culturable number/sample = 3.0 × 105, SD = 5.6 × 104) was not significantly different from that collected by the SKC Biosampler® (mean total copy number/sample = 1.5 × 108, SD = 1.6 × 107; mean culturable number/sample = 3.1 × 105, SD = 6.4 × 104) and is shown in .
FIG. 4 Comparison of influenza A virus aerosol measured by focus assay infectivity analysis and by quantitative PCR for the G-II and SKC BioSampler®. The T/I ratio is the ratio of total virus RNA particles (from qPCR analysis) to infectious virus particles (from the infectivity assay).
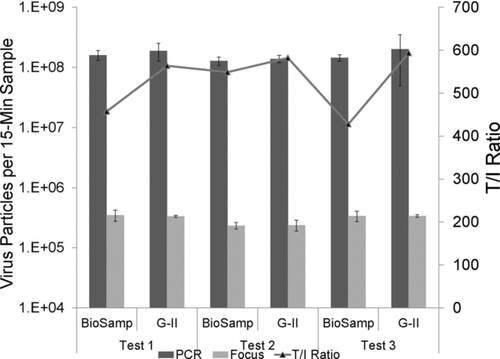
Measured virus concentrations can be used to calculate a ratio of total virus RNA copies (from RT-qPCR analysis) to infectious virus particles (from the infectivity assay), and is referred to as the T/I ratio – the number of RNA copies per culturable virus. A lower T/I ratio correlates to a higher proportion of viruses collected that remain infective. The T/I ratios for the G-II (mean = 5.8 × 102, SD = 1.5 × 101) and BioSampler (mean = 4.8 × 102, SD = 6.3 × 101) are also shown in . Although the G-II did tend to have higher T/I ratios than the BioSampler, these T/I ratios were comparable and not significantly different (p = 0.11); the G-II collected 83% as much virus that remained culturable as compared to the BioSampler. The use of “liquid based” samplers such as impingers have been reported to maintain infectivity of collected viruses better than “dry” type samplers such as filters or impactors. The T/I ratio for the G-II compared favorably to commonly used, traditional air sampling methods and was between 2.3 and 4.8 times lower than those reported by Fabian et al. Citation2009a for filter samplers and a polyurethane foam impactor.
Some loss of virus infectivity might be expected because of using a high RH environment for the sample collection and adding steam. However, residence time from release in the cone to collection is at most about 5 s. Less than 4 L of steam (approximately 2.3 g of water per minute) is added to 130 L of air and the residence time in the saturator is about 0.4 s. As a result, based on previous work (McDevitt et al. Citation2010), minimal biological decay would be expected under these conditions with such short exposures.
During operation, the G-II grows particles by condensing water vapor from the air onto the particles and onto the walls of the system. The water-laden particles from the air stream are removed in the fine particle collector by the impactor. Condensed water deposited on the walls also drains through the fine particle collector due to gravity and airflow through the G-II. As such, the G-II acts as a dehumidifier and removes moisture from the air. This moisture eventually ends up in the reservoir along with collected influenza virus. The condensate is essentially pure water, and not an ideal environmental for virus survival. For this reason, concentrated buffer was added to the reservoir through a valve at the base of the reservoir with a syringe pump to yield a final, 1× concentration of buffer/sample solution in the reservoir. To minimize the total volume of liquid accumulated in the reservoir, a 10× buffer was used. For example, if the G-II condenses 4 mL of water per minute, then 10× buffer was added at the rate of 0.4 mL/min to yield approximately a 1× final concentration. Matching the feed rate and buffer concentration to water condensed by the sampler is critical to optimal preservation of virus infectivity.
In our virus aerosol testing experiments, we introduced the aerosol through the mouth of a mannequin to simulate turbulence produced at the cone by the presence of the head of a test subject. However, this test apparatus did not simulate normal homeostatic breathing patterns or high-velocity events such as coughing or sneezing. Peak flow rates during cough have been measured and generally range from 200–500 lpm (Gupta et al. Citation2009; Lindsley et al. Citation2010) with durations of less than 0.5 s (Gupta et al. Citation2009). For reasons of practicality, our system flow rate was designed for 130 lpm. A cough has a very short duration at peak flows (typically less than 0.5 s) and would be projected forcibly forward into the cone. Thus, the large volume of the cone provides a capacitance for the brief excess airflow and the small fraction of air displaced from the cone would likely be “clean air.” Computational fluid dynamic analysis of the cone inlet and testing with more advanced breathing mannequins are areas for future characterization and system optimization.
Existing samplers did not meet our requirements for operating at high flow rates (≥130 lpm), maintaining virus infectivity, and efficiently collecting submicron particles. Since viruses associated with human infection require mammalian cells to infect and replicate, culture methods used to collect and grow bacteria and fungi directly onto culture media cannot be used for viruses. Thus, samplers such as the Andersen N-6 impactor or SAS sampler that simply impact organisms onto a synthetic growth medium could not be considered, and their flow rates are much too low (maximum of 28.3 lpm). Liquid impingers, such as the AGI-30 and the SKC BioSampler, have been shown to effectively collect viruses and maintain infectivity, but their flow rates are also much too low (12 lpm). Filters can efficiently remove submicron particles and can be used at high flow rates, albeit with either high-pressure drops or large surface areas, but influenza viruses quickly lose infectivity on filters (Fabian et al. Citation2009a). Wetted-wall cyclones are used to collect and concentrate bioaerosols in a liquid and typically have flow rates in excess of 250 lpm and are suited for maintaining biological survival (McFarland et al. Citation2010). However, reported submicron collection efficiency has been low for wet-walled cyclones (Kesavan et al. Citation2008; McFarland et al. Citation2010). The G-II addresses each of the problems inherent in legacy bioaerosol sampling devices, achieves high flow rates, is efficient for submicron particle collection, and preserves infectiousness of viruses.
Acknowledgments
This study was funded by the Centers for Disease Control and Prevention cooperative agreements 1U01CI000446 and IU01P000497, NIH grant RC1AI086900, the U.S. Federal Aviation Administration (FAA) Office of Aerospace Medicine through the Air Transportation Center of Excellence for Airliner Cabin Environment Research (Cooperative Agreement 04-C-ACE-HU and 07-C-RITE-HU) via a subcontract from Auburn University (#06-ACER-207814).
REFERENCES
- Brankston , G. , Gitterman , L. , Hirji , Z. , Lemieux , C. and Gardam , M. 2007 . Transmission of Influenza A in Human Beings . Lancet Infect. Dis. , 7 : 257 – 265 .
- CDC . 2010 . Prevention Strategies for Seasonal Influenza in Healthcare Settings , Atlanta , GA : Centers for Disease Control and Prevention . Available online at: http://www.cdc.gov/flu/professionals/infectioncontrol/healthcaresettings.htm (accessed 14 November 2012)
- Diaz , K. T. and Smaldone , G. C. 2008 . Quantifying Exposure Risk: Surgical Masks and Respirators . Am. J. Infect. Control , 38 : 501 – 508 .
- Fabian , P. , McDevitt , J. J. , DeHaan , W. H. , Fung , R. O. , Cowling , B. J. Chan , K. H. 2008 . Influenza Virus in Human Exhaled Breath: An Observational Study . PLoS One , 3 : e2691
- Fabian , P. , McDevitt , J. J. , Houseman , E. A. and Milton , D. K. 2009a . Airborne Influenza Virus Detection with Four Aerosol Samplers Using Molecular and Infectivity Assays: Considerations for a New Infectious Virus Aerosol Sampler . Indoor Air. , 19 : 433 – 441 .
- Fabian , P. , McDevitt , J. J. , Lee , W. M. , Houseman , E. A. and Milton , D. K. 2009b . An Optimized Method to Detect Influenza Virus and Human Rhinovirus from Exhaled Breath and the Airborne Environment . J. Environ. Monit. , 11 : 314 – 317 .
- Fennelly , K. P. , Jones-Lopez , E. C. , Ayakaka , I. , Kim , S. , Menyha , H. Kirenga , B. 2012 . Variability of Infectious Aerosols Produced during Coughing by Patients with Pulmonary Tuberculosis . Am. J. Respir. Crit. Care Med. , 186 : 450 – 457 .
- Fennelly , K. P. , Martyny , J. W. , Fulton , K. E. , Orme , I. M. , Cave , D. M. and Heifets , L. B. 2004 . Cough-Generated Aerosols of Mycobacterium Tuberculosis: A New Method to Study Infectiousness . Am. J. Respir. Crit. Care Med. , 169 : 604 – 609 .
- Gerone , P. J. , Couch , R. B. , Keefer , G. V. , Douglas , R. G. , Derrenbacher , E. B. and Knight , V. 1966 . Assessment of Experimental and Natural Viral Aerosols . Bacteriol. Rev. , 30 : 576 – 588 .
- Gupta , J. K. , Lin , C. H. and Chen , Q. 2009 . Flow Dynamics and Characterization of a Cough . Indoor Air , 19 : 517 – 525 .
- Gupta , T. , Demokritou , P. and Koutrakis , P. 2004 . Development and Performance Evaluation of a High-Volume Ultrafine Particle Concentrator for Inhalation Toxicological Studies . Inhal. Toxicol. , 16 : 851 – 862 .
- IOM . 2009 . Respiratory Protection for Healthcare Workers in the Workplace Against Novel H1N1 Influenza A: A Letter Report , Washington , DC : The National Academies Press .
- Johnson , D. F. , Druce , J. D. , Birch , C. and Grayson , M. L. 2009 . A Quantitative Assessment of the Efficacy of Surgical and N95 Masks to Filter Influenza Virus in Patients with Acute Influenza Infection . Clin. Infect. Dis. , 49 : 275 – 277 .
- Kesavan , J. , Bottiger , J. R. and McFarland , A. R. 2008 . Bioaerosol Concentrator Performance: Comparative Tests with Viable and with Solid and Liquid Nonviable Particles . J. Appl. Microbiol. , 104 : 285 – 295 .
- Kidwell , C. B. and Ondov , J. M. 2001 . Development and Evaluation of a Prototype System for Collecting Sub-Hourly Ambient Aerosol for Chemical Analysis . Aerosol Sci. Tech. , 35 : 596 – 601 .
- Lin , X. , Reponen , T. A. , Willeke , K. , Grinshpun , S. A. , Foarde , K. K. and Ensore , D. S. 1999 . Long-Term Sampling of Airborne Bacteria and Fungi Into a Non-Evaporating Liquid . Atmos. Environ. , 33 : 4291 – 4298 .
- Lindsley , W. G. , Blachere , F. M. , Thewlis , R. E. , Vishnu , A. , Davis , K. A. Cao , G. 2010 . Measurements of Airborne Influenza Virus in Aerosol Particles from Human Coughs . PLoS One , 5 : e15100
- Macher , J. M. 1997 . Evaluation of Bioaerosol Sampler Performance . Appl. Occ. Env. Hygiene , 12 : 730 – 736 .
- Marple , V. A. and Willeke , K. 1976 . Impactor Design . Atmos. Environ. , 10 : 891 – 896 .
- McDevitt , J. J. , Rudnick , S. N. , First , M. W. and Spengler , J. D. 2010 . The Role of Absolute Humidity on the Inactivation of Influenza Viruses on Surfaces at Elevated Temperature . Appl. Environ. Microbiol. , 76 : 3943 – 3947 .
- McFarland , A. R. , Haglund , J. S. , King , M. D. , Hu , S. , Phull , M. S. Moncla , B. W. 2010 . Wetted Wall Cyclones for Bioaerosol Sampling . Aerosol Sci. Tech. , 44 : 241 – 252 .
- Morawska , L. 2006 . Droplet Fate in Indoor Environments, or Can We Prevent the Spread of Infection? . Indoor Air , 16 : 335 – 347 .
- Nicas , M. and Jones , R. M. 2009 . Relative Contributions of Four Exposure Pathways to Influenza Infection Risk . Risk Anal. , 29 : 1292 – 1303 .
- Noti , J. D. , Lindsley , W. G. , Blachere , F. M. , Cao , G. , Kashon , M. L. Thewlis , R. E. 2012 . Detection of Infectious Influenza Virus in Cough Aerosols Generated in a Simulated Patient Examination Room . Clin. Infect. Dis. , 54 : 1569 – 1577 .
- Orsini , D. A. , Rhoads , K. , McElhoney , K. , Schick , E. , Koehler , D. and Hogrefe , O. 2008 . A Water Cyclone to Preserve Insoluble Aerosols in Liquid Flow – An Interface to Flow Cytometry to Detect Airborne Nucleic Acid . Aerosol Sci. Tech. , 42 : 343 – 356 .
- Rose , V. E. 2003 . “ History and Philosophy of Industrial Hygiene ” . In The Occupational Environment: Its Evaluation, Control, and Management , Edited by: DiNardi , S. R. 2 – 10 . Farifax : AIHA Press .
- Rudnick , S. N. , McDevitt , J. J. , First , M. W. and Spengler , J. D. 2009 . Inactivating Influenza Viruses on Surfaces Using Hydrogen Peroxide or Triethylene Glycol at Low Vapor Concentrations . Am. J. Infect. Control , 37 : 813 – 819 .
- Sakaguchi , T. and Ehara , K. 2011 . Primary Standard for the Number Concentration of Liquid-Borne Particles in the 10 to 20 μm Diameter Range . Measurement Science & Technology , 22 ( 2 )
- Stanley , W. M. 1944 . The Size of Influenza Virus . J. Exp. Med. , 79 : 267 – 283 .
- Tellier , R. 2006 . Review of Aerosol Transmission of Influenza A Virus . Emerg. Infect. Dis. , 12 : 1657 – 1662 .
- van Elden , L. J. , Nijhuis , M. , Schipper , P. , Schuurman , R. and van Loon , A. M. 2001 . Simultaneous Detection of Influenza Viruses A and B Using Real-Time Quantitative PCR . J. Clin. Microbiol. , 39 : 196 – 200 .
- WHO . 2009 . Infection Prevention and Control During Health Care for Confirmed, Probable, or Suspected Cases of Pandemic (H1N1) 2009 Virus Infection and Influenza-Like Illnesses , World Health Organization . Available online at: http://www.who.int/csr/resources/publications/cp150_2009_1612_ipc_interim_guidance_h1n1.pdf (accessed 14 November 2012)