Abstract
Adhesion and bounce of liquid and solid particles upon high-velocity impact with a surface has been investigated using semi-empirical and explicit hydrodynamic simulations. Ammonium nitrate (AN) and sodium chloride (NaCl) were selected as test compounds for the liquid and solid particles, respectively, and tungsten (W) as the target surface. Changes in the shape, temperature, strain, and rebound velocity of these particles upon high-velocity impact are investigated assuming operational conditions (particle diameter, and velocity) of Aerodyne aerosol mass spectrometer (AMS). The simulations show that the AN particles adhere to the W surface, which is consistent with previous experimental studies. In the case of NaCl, the collection efficiencies depend significantly on the stress–strain characteristics of the crystal. Our results suggest that, in addition to particle phase and impact velocity, anisotropy of the elastic properties and brittleness are key factors in controlling the adhesion and bounce of solid particles.
Copyright 2013 American Association for Aerosol Research
1. INTRODUCTION
Particle bounce is an important and problematic phenomenon in aerosol sampling methods that are based on high-velocity impact (Rao and Whitby Citation1978a,Citationb; Jayne et al. Citation2000). Previous studies (Cheng and Yeh Citation1979) have suggested that particles above a certain threshold velocity (or kinetic energy) can bounce off the surface. Furthermore, recent experimental studies using Aerodyne aerosol mass spectrometers (AMS) and low-pressure impactors (LPI) have suggested that the collection efficiency of solid particles is substantially lower than 100% and is dependent on factors such as the relative humidity (RH) of the sampled air, the chemical composition of the particles, and the particle mixing state (Matthew et al. Citation2008; Virtanen et al. Citation2010; Chen et al. 2011). These studies have assumed that the adhesion and bounce of particles from a surface can be characterized solely by the phase (liquid or solid) and mixing state of the aerosol particles. Nonzero collection efficiencies for solid particles (e.g., of Tsai and Cheng Citation1995) have not yet been fully discussed in previous studies.
FIG. 1 Relationships between impact velocities (Vi ) and particle sizes (Da or D va) for various particle impact phenomena (based on the paradigm originally proposed by Klinkov et al. [Citation2005]). Extreme regimes include erosion, cold spray, and hypervelocity impacts (Klinkov et al. Citation2005). Particle geometric diameters for each phenomena given in Klinkov et al. (Citation2005) are converted to Da by assuming that particle density is 1.5 g cm−3. The impact velocity-size relationships in AMS (black circles), MOUDI (open circles), and ELPI (gray circles) systems are shown as examples. Data for AMS, MOUDI, and ELPI were taken from Takegawa et al. (Citation2005), Marple et al. (Citation1991), and Keskinen et al. (Citation1992), respectively. For MOUDI and ELPI, particle diameters correspond to the 50% cut point Da at each stage in MOUDI and ELPI. The area enclosed by thick dashed black lines corresponds to the regime of the aerosol sampling techniques (e.g., Aerodyne AMS).
![FIG. 1 Relationships between impact velocities (Vi ) and particle sizes (Da or D va) for various particle impact phenomena (based on the paradigm originally proposed by Klinkov et al. [Citation2005]). Extreme regimes include erosion, cold spray, and hypervelocity impacts (Klinkov et al. Citation2005). Particle geometric diameters for each phenomena given in Klinkov et al. (Citation2005) are converted to Da by assuming that particle density is 1.5 g cm−3. The impact velocity-size relationships in AMS (black circles), MOUDI (open circles), and ELPI (gray circles) systems are shown as examples. Data for AMS, MOUDI, and ELPI were taken from Takegawa et al. (Citation2005), Marple et al. (Citation1991), and Keskinen et al. (Citation1992), respectively. For MOUDI and ELPI, particle diameters correspond to the 50% cut point Da at each stage in MOUDI and ELPI. The area enclosed by thick dashed black lines corresponds to the regime of the aerosol sampling techniques (e.g., Aerodyne AMS).](/cms/asset/96ff051c-97bc-45af-9a34-af541461bb32/uast_a_763895_o_f0001g.gif)
In order to minimize particle bounce from a surface following impact, the collection surface has been coated with a sticky material such as oil or grease, thus increasing the adhesion energy (McFarland and Zeller Citation1963; Pak et al. Citation1992). This coating method, however, is not suitable for off- and on-line chemical analyses of collected samples. The development of a reliable method for chemical analyses that minimizes particle bounce from a surface will require a more detailed understanding the physical fundamentals of high-velocity solid particles bouncing and adhering to a surface.
Particle impact with surfaces has been extensively studied over a wide range of impact velocities (Vi ) and particle sizes (Klinkov et al. Citation2005, and references therein). Klinkov et al. (Citation2005) summarizes the ranges of impact velocities and particle sizes for several categories of particle impact phenomena (, based on the paradigm originally proposed by Klinkov et al. [Citation2005]). Particle size is based on the aerodynamic (Da ) or vacuum aerodynamic diameters (D va), which is equivalent to Da at high vacuum. Phenomena occurring at the extreme values of Vi and Da include erosion (Vi = 50 to 300 m s−1 and Da = 120 to 2 mm), cold spray (Vi = 300 to 1200 m s−1 and Da = 20 to 0.6 mm), and hypervelocity impacts (Vi > 2 to 3 km s−1) (see Klinkov et al. (Citation2005) for details of these processes). Because of the very high incident kinetic energies of particles in these regimes, the particles very likely deform and break apart upon impact with a surface, regardless of their phase. On the other hand, high-velocity impacts of aerosol particles observed in aerosol sampling regimes using instrumentation such as AMS and LPI appear at values of Vi < ∼300 m s−1 and Da or D va < ∼10 μm and can exhibit different behaviors such as adhesion or bounce. The relationship between Vi and D va of our Aerodyne AMS (Takegawa et al. Citation2005), which incorporates an aerodynamic lens (ADL) (Liu et al. Citation1995), is plotted in . For comparison, that for a MOUDI (micro-orifice uniform deposit impactor) and ELPI (electrical LPI) is also shown.
The microphysical behavior (e.g., particle-surface interactions) of high-velocity impacts of aerosol particles related to aerosol sampling was originally investigated by theoretical and experimental approaches (Dahneke Citation1971, Citation1973, Citation1975). A number of additional studies have since verified, and improved the bounce studies given by Dahneke (Paw U Citation1983; Rogers and Reed Citation1984; Derjaguin et al. Citation1987; Tsai et al. Citation1990). According to energy conservation upon particle impact which was quantitatively investigated by Tsai et al. (Citation1990), two important features have been identified.
1. | For low-velocity impacts (lower than ∼5 m s−1), elastic flattening and surface roughness are key factors for enhancing adhesion energy in determining the collection efficiency of solid particles. | ||||
2. | For high-velocity impacts (higher than 20 to 30 m s−1), solid particles impacting upon the surface can plastically deform, resulting in substantial loss of the incident kinetic energy. |
Although these studies revealed key factors that affect adhesion and bounce of a particle upon impact, there are other processes, such as energy loss due to thermodynamic works and fracturing process resulting from large deformations that were not considered in previous studies. Thermodynamic work, however, can lead to substantial increases in temperature inside particles.
Our primary goal of this study was to understand the particle bounce phenomenon in the Aerodyne AMS, which is now widely used in aerosol researches. Key parameters are therefore set to match the typical operating condition of an AMS. We also aimed to investigate, in general, the important factors that may play an important role when a particle impacts with a surface. For this purpose, we adopted two approaches: the semi-empirical particle bounce model (T1990 model) by Tsai et al. (Citation1990) and explicit hydrodynamic simulations. The T1990 model is used to assess the importance of plastic deformation of solid particles and the effect of anisotropy of their elastic properties upon particle impact, but it cannot be used for simulating adhesion and bounce of the liquid particles. Hydrodynamic simulations are employed to explicitly investigate changes in the physical properties of the liquid and solid particles during the course of high-velocity impact with a surface. We chose ANSYS AUTODYN for these simulations. AUTODYN has been commonly used for the study of a wide variety of explosion and impact processes (Katayama et al. Citation1997). It should be noted that both simulations do not account for interactions between air molecules and particles.
2. CONCEPTUAL DESCRIPTION
We investigated the high-velocity impact of liquid and solid particles on a flat Tungsten (W) surface in high vacuum (no interaction between gas molecules and particles). W is a representative hard metal and has been used for the material of the vaporizer of Aerodyne AMS (Jayne et al. Citation2000). The impact velocity of the submicron particles was chosen based on the typical performance of an ADL (Liu et al. Citation1995; Zhang et al. Citation2004). The temperature of both the submicron particles and W surface was assumed to be room temperature (300 K) in order to investigate impact-induced changes in temperature within the submicron particles. Ammonium nitrate (AN) and sodium chloride (NaCl) were selected as the materials of the liquid and solid particles, respectively. The reasons why we have chosen these compounds are these are ubiquitously found in atmosphere and also the material properties necessary for our simulations, including shock wave data, elastic property, and stress-strain characteristics, are available for these compounds. Previous studies have suggested that once AN deliquesces at the deliquescence relative humidity (DRH), it can exist as a metastable liquid droplet at a lower RH than its DRH (Tang Citation1980; Richardson and Hightower Citation1987; Lightstone et al. Citation2000). We therefore assumed that the AN particles exist as liquid droplets. NaCl is one of the most ubiquitous inorganic aerosols in the atmosphere and is in the form of a solid particle at a lower RH than its crystallization RH (Tang Citation1980). It is important to note that the elastic properties of NaCl crystals are anisotropic (Murri and Anderson Citation1970), allowing the investigation of the effects of elastic anisotropy of crystalline particles during the high-velocity impact.
The T1990 model considers the relationship between contact deformation and contact surface energy. It uses the material density, Young's modulus, Poisson's ratio, Hamakar constant, and stress strain curve as input parameters. Through solving a simple energy conservation equation for a particle with an empirically derived critical velocity for bulk plastic deformation, the T1990 model can calculate the velocity of a particle rebounding from the surface. In contrast, the AUTODYN is a finite volume method-based analysis tool for simulating the nonlinear dynamics of solids, fluids, gases, and their interactions. The AUTODYN considers the equation of state (EOS), material constitutive law, and failure law for each cell of the particle and surface (see Section 4.1 and the online supplementary information for details). While some detailed physical properties (e.g., shock wave data) are needed for the simulations, the AUTODYN can provide information on the time-dependent changes in shape, temperature, pressure, and internal and kinetic energies of particles. The ANSYS AUTODYN cannot deal with molecular interactions between particles and the surface, indicating that this model is not recommended to be used for analyzing the particle impact with lower impact velocities (<∼5 m s−1).
3. SIGNIFICANCE OF PLASTIC DEFORMATION AND ANISOTROPY OF ELASTICITY OF SOLID PARTICLES
Using the T1990 model, it is possible to calculate the velocity of rebounding particles (Vr ) and coefficient of restitution (COR) for a wide range of impact velocities (Vi ). In this section, we describe the deformation processes taking place upon the particle impact using the T1990 model.
The minimum Vi at which bulk plastic deformation can occur, Viy , is calculated using the method given by Tsai et al. (Citation1990) (see the online supplementary information for details). The predicted values of Viy are strongly dependent on the yield stress of the material, σy , where the material yielding processes occur (). Estimated values of σy of NaCl ranged from ∼0.01 to ∼1 GPa (see the following for details) and the predicted values of Viy for NaCl particles were lower than 100 m s−1. Vi of submicron particles in all devices are generally greater than ∼30 m s−1 as shown in . The predicted Viy here using the T1990 model are comparable to or greater than Vi in the impactors and ADL systems. We may need to consider possible influence of the plastic deformation of submicron particles on the impact process for those devices.
FIG. 2 V iy calculated for the impact of NaCl particles on the W surface as a function of their yield stresses.
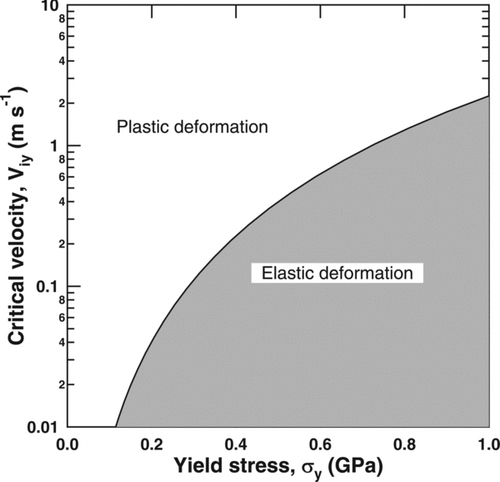
The T1990 model can also simulate Vr and COR using the physical properties of the materials (e.g., Young's modulus) and was thus used to calculate COR for the NaCl particles. The incident and rebound kinetic energies are defined as KEi and KEr , respectively. The COR of the particles upon the impact is defined as the ratio of Vr to Vi . The total energy after impact is the sum of KEr , the energy loss breaking contact surface (EL cs), plastic strain energy (IEp ), and the energy loss due to local asperity deformation (EL asp). Note that IEP is the internal energy stored inside the particles throughout the impact. The following conservation of energy equation is derived:
The failure of the particle structure caused by significant deformation and thermodynamic work done inside the particle are not taken into account in the T1990 model. The T1990 model, therefore, cannot accurately simulate the energy budget for cases involving large deformations which can lead to significant changes in the internal energy comparable to KEi . Despite this limitation, the model can be used for investigating the effect of mechanical properties such as σy during solid particle impact and predicting particle bounce.
The particle diameter was assumed to be 250 nm in all simulations. Values of Vi were determined based on the typical particle velocity-D va relationship in the ADL (). The values of D va (≡ (ρp /χ)·Dp , where ρp is the particle density, χ is the dynamic shape factor (χ ≡ 1 for spherical shape), and Dp is the geometric diameter, DeCarlo et al. [Citation2004]) for the 250-nm AN and NaCl particles were calculated to be 433 and 540 nm, respectively, using those particle densities (1.73 and 2.16 g cm−3, respectively). Spherical morphology of the particle was also assumed. Intermediate Vi values of 90 m s−1 were estimated using data in .
In the simulation, the Hugoniot elastic limit (P HEL) was used to estimate the order of σy for NaCl through the following equation:
TABLE 1 COR predicted by the T1990 model as a function of σy
TABLE 2 Summary of the hydrodynamic simulations of liquid and solid particle impact
The values of COR predicted by T1990 model as a function of σy were listed in . For higher σy values (0.5 and 1.0 GPa), predicted values of COR were larger than zero, indicating that the NaCl particles bounced off the W surface under these conditions. COR at σy = 1.0 GPa was 0.154, so the particle bounce was not only elastic but also plastic. COR was close to zero at σy = 0.5 GPa, suggesting almost all of KEi was converted to be IEP . For lower values of σy (0.02 and 0.1 GPa), predicted values of COR were zero, indicating that the NaCl particles adhered to the W surface. According to these simulation results, significant plastic deformation during impact leads to a large loss of kinetic energy (98% loss with σy = 1.0 GPa and 100% loss with the lower σy ). Therefore, the collection efficiency for solid particles was strongly dependent not only on their elasticity (e.g., Young's modulus) but also on their material yield strength, σy .
4. HYDRODYNAMIC SIMULATIONS
4.1. Simulation Details
Two-dimensional axisymmetry, completely flat surface, in high vacuum, and spherical shape of particles before impact were assumed in order to simplify the model system. The detail of the coordinate system of the AUTODYN simulation is given in the online supplementary information. A Lagrange solver was used for the particles and the W surface to clearly delineate their boundary.
FIG. 5 Simulation results of the impact of NaCl particles. (a; left panel) Temporal evolutions of fractional contribution of KE (thick solid lines) and IE (thin dashed lines) for the cases NaCl_case1 (σy = 1.0 GPa). (a; right panel) Temporal evolutions of fractional contribution of ThW (thick solid lines) and IEP (thin dashed lines) for the cases NaCl_case1. (b; left panel) Temporal evolutions of fractional contribution of KE (solid lines) and IE (dashed lines) of the NaCl particle for the cases NaCl_case3 (σy = 0.1 GPa) (black) and NaCl_case4 (σy = 0.02 GPa) (shaded). (b; right panel) Temporal evolutions of fractional contribution of ThW (thick solid lines) and IEP (thin dashed lines) for the cases NaCl_case3 (black) and NaCl_case4 (gray).
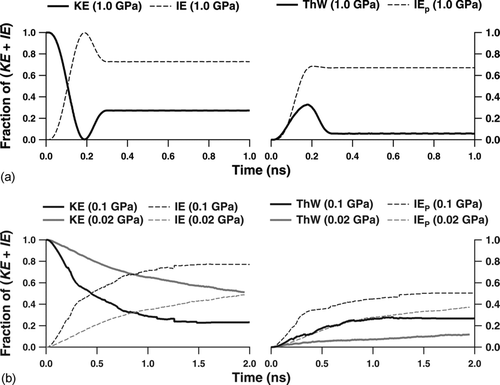
FIG. 6 Simulated profiles of (a) pressure, (b) temperature, (c) effective plastic strain, and (d) material status at t = 0.25 and 1.0 ns for the case of NaCl_case1 (σy = 1.0 GPa). The black area in the material status map is defined as the region that underwent failure due to large deformations.
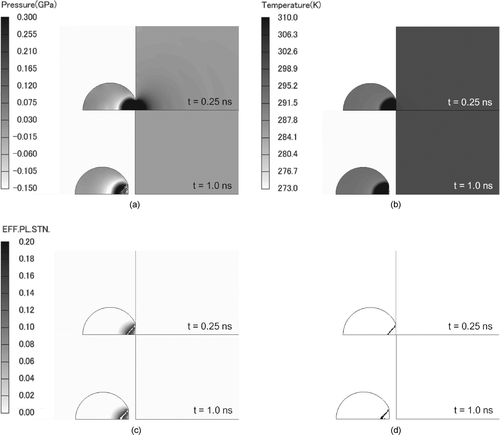
An EOS is needed to describe the relationship between hydrostatic pressure, local density, and local specific energy (i.e., temperature). We chose the Mie-Grüneisen form of EOS based on the shock Hugoniot for the particle and the W surface. The Steinberg–Guinan model was used as the material constitutive law for the W surface (Steinberg et al. Citation1980). We assume that the W surface does not fail from the particle impact. Details of the Mie-Grüneisen EOS and Steinberg–Guinan model are shown in the online supplementary information. For the NaCl particles, the elastic-perfectly-plastic (von Mises) model was used as the material constitutive law. We did not apply the material constitutive and failure laws for simulating the AN particles, because they are assumed to be liquid. In the von Mises model, changes in the stress, σ, are regulated by σy
and maximum plastic strain, (). Effects of a work hardening after the yielding point are neglected, because those are not important for ionic crystals like NaCl (Black and Kohser Citation2007). The linear relationship between σ and the strain, ϵ, where σ < σy
follows the Hooke's law, and the slope of the stress–strain relationship is the Young's modulus. When ϵ of the particle exceeds
during the impact process, the particle undergoes failure ().
The conditions assumed in the hydrodynamic simulations were summarized in . Values of σy
used in the cases from NaCl_case1 to NaCl_case4 were 1.0, 0.5, 0.1, and 0.02 GPa, respectively, for the same reason presented in Section 2. The effects of anisotropy of elastic property on the particle impact were investigated under these conditions. The sensitivity of the incident velocity was also tested by doubling incident velocity to 180 m s−1 (NaCl_case5 in ). It should be noted that changes in strain rates caused by changes in Vi
can affect the stress–strain characteristics (Tanimura et al. Citation2006). Here, we assumed that the stress-strain characteristics of NaCl were not altered by changes in Vi
. Furthermore, a lower value of , was used to investigate the importance of bulk failure of the solid particles, which can mimic the brittle fracture of solid particles upon impact (NaCl_case6 and _case7 in ).
4.2. Simulation Results
4.2.1. AN Particle (Liquid Particle)
Simulation results from the case AN_case1 in are discussed in this section. We show the temporal evolution of the energy budget (i.e., breakdown of energies of particles) of an AN particle during the course of the high-velocity impact, which is helpful for interpreting the particle impact (). The AN particle did not immediately lose kinetic energy (KE) and the internal energy (IE) of AN particle slowly increased. Such evolutions are typically associated with the fluidic motion of materials. The AN particle continued in the fluidic motion along the W surface during the entire simulation period of 1 ns, and did not bounce off the W surface within 1 ns. Although simulations were not performed after 1 ns, the AN particle will very likely spread over the W surface and never bounce off. The AN particle showed no elastic deformation and there was little conversion of KE to IE. Owing to this negligible conversion to IE, no large increase in pressure and temperature inside the AN particle () was observed ().
4.2.2. NaCl Particle (Solid Particle)
The temporal evolution of the energy budget of NaCl particles for the case of NaCl_case1 is given in . NaCl particles under this condition bounced off the W surface, namely COR > 0 as shown in . After contact with the W surface, the KE of the NaCl particle immediately decreased and was converted to its IE. At t = 0.2 ns, almost all of the KE were converted into IE. Subsequently, IE was reconverted into KE. The value of COR in the case NaCl_case1 was ∼0.5 (), indicating that the bounce was not only elastic and but also plastic. For solid particles, the IE can be separated into two contributions: plastic strain energy (IEP
) and thermodynamic work (ThW). Here, ThW can be calculated by integrating ∫Pdv inside the particle. The fractional contribution of IEP
and ThW to the total energy for the case of NaCl_case1 indicates that elastic and plastic deformation occurred inside the NaCl particle (). The IEP
of the particle contributed to ∼70% of the total energy at t = 1 ns. The value of COR in the case of NaCl_case2 was 0.112 () reflecting the plastic deformation of the particle and significant loss (∼99%) of the KE. Note that the NaCl particle did not bounce off the W surface during the simulation period (2 ns) in spite of the positive value of COR. As discussed in Section 2, the T1990 model estimated COR for the case of NaCl_case2 to be 0.015 (), thus the T1990 model overestimated the loss of IEP
. This discrepancy is likely the result of differences in the energy loss processes considered by both models (details in Section 2). Profiles of P and T on the particle and surface were calculated at t = 0.25 and 1.0 ns for the case NaCl_case1 (, respectively). The maximum IE, which occurred at t = 0.25 ns, led to a significant increase in the P and T inside the NaCl particle. The largest increases in the P and T (ΔT
max) were greater than ∼1.0 GPa and ∼120°C, respectively. The largest P increase was higher than the σy
(1.0 GPa) assumed in the simulation, resulting in significant plastic strain in the high-P region inside the NaCl particle (). The rebounding NaCl particle at t = 1.0 ns had a plastically deformed region, corresponding to the increased T region (). The effective plastic strain of zero was found in the NaCl particle (indicated by a white wedge-shape area in ), which was caused by bulk fail associated with a deformation exceeding . In order to better understand the bulk fail inside the particles, material status maps for t = 0.25 and 1.0 ns are also shown in . The bulk failed region at t = 1.0 ns corresponds to 0.4% of mass of the NaCl particle ().
FIG. 7 Simulated profiles of (a) pressure and temperature, and (b) effective plastic strain and material status at t = 1.0 ns for the case NaCl_case4 (σy = 0.02 GPa). The black area in the material status map is defined as the region that underwent failure due to large deformations.
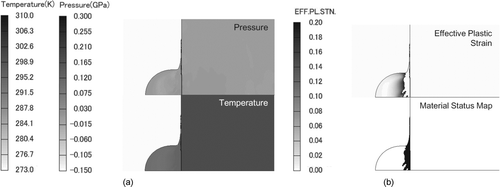
The behavior of the NaCl particles with lower σy (the cases of NaCl_case3 and NaCl_case4) were notably different from those for the cases with higher σy (NaCl_case1). NaCl particles were captured by the W surface in the cases with lower σy . Gradual decreasing trends of KE for these cases indicated that NaCl particles continued moving for the 2 ns duration of calculation (). The remaining KE could be attributed to motions associated with the impact and in the horizontal direction (parallel to the W surface). Since there was no notable conversion of KE to IE, little change in T was observed. depicts the profiles of P and T inside the NaCl particle at t = 1 ns for the case of NaCl_case4. Bulk fail occurred inside the NaCl particles under these cases. Effective plastic strain and material status map were given in . Significant portions (27% by mass at t = 1 ns) of the NaCl particles were failed due to large deformations, compared to other cases (). The fracturing resulting from high-velocity impacts is expected to be an important process hindering the reconversion of IE to KE and subsequent loss of KE in the rebound direction. Even though the assumed particle phase of NaCl was solid, particles with a lower σy (especially, σy = 0.02 GPa) can adhere to the W surface upon the high-velocity impact, similar to what is observed for liquid particles (Section 4.2.1).
4.2.3. Sensitivity of Incident Velocity and
to the High-Velocity Impact of Solid Particles
Results from doubling the incident velocity (NaCl_case5) were similar to those for the case of NaCl_case2 (). The NaCl particle having two times higher velocity lost almost all of its kinetic energy (COR ∼ 0.02) and kept been captured by the W surface during the simulation period. Significant plastic deformations (maximum effective plastic strain exceeded ) were observed inside the particle, which resulted in a notable increase in T (ΔT
max ∼130°C). The degree of kinetic energy loss of the solid particles seems to be dependent on the incident kinetic energies for high-velocity impact: The higher the incident velocity of solid particles, the lower the COR values. These simulations confirm this point, which was also suggested in Dahneke (Citation1975) and Tsai et al. (Citation1990) and emphasize the importance of defining the incident velocity and diameter of solid particles when studying the particle bounce and adhesion.
FIG. 8 Dependence of COR on σy
calculated using AUTODYN and T1990 model. Filled markers indicate the AUTODYN simulation results assuming 0.2 of

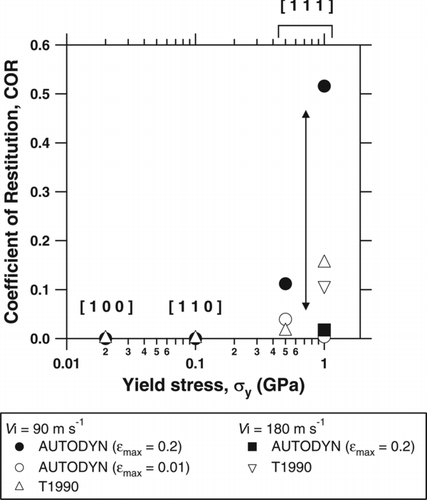
The simulation having a smaller (NaCl_case6 and _case7) showed the similar result as the case NaCl_case2 (). According to the von Mises model, solid particles with smaller
are inhibited from undergoing significant plastic deformation. The region with large strain inside the particle was failed for the case of NaCl_case6 and _case7. The predicted failure of the NaCl particle resulted in large losses of the KE in the rebound motion and a lower COR (<∼0.04) than that (∼0.5) for NaCl_case1. This result further illustrates the importance of the fracturing process in kinetic energy loss through the high-velocity impact process.
5. WHAT IS THE CONTROLLING FACTOR AFFECTING THE PARTICLE BOUNCE?
The relationship between COR and σy
for NaCl particles is summarized in . As described in section 4.2.2 (NaCl_case1–case4), the COR values were strongly dependent on the assumed σy
values, which represent the importance of the anisotropy of elasticity. Even though the COR-σy
relationship in T1990 model was quantitatively different from that in AUTODYN, both simulations showed a similar tendency of the COR-σy
relationships. The crystal surface direction-dependent σy
for ionic crystals can be an essential factor to largely affect the solid particle impact, that is, adhesion (zero-COR) or bounce (nonzero-COR). We conducted additional simulations to investigate the influence of Vi
and brittleness (i.e., ), and found that both parameters are important for kinetic energy loss processes. Large changes in these parameters can definitively affect the solid particle bounce as indicated by the vertical two-way arrow of .
Common features found in two models are that NaCl particles never bounce off the W surface for lower σy cases (0.02, 0.1 GPa) and that they tend to bounce off the W surface for higher σy cases (0.5, 1.0 GPa) under various simulation conditions. The difference in the model and changes in Vi and brittleness can alter the absolute values of COR in the latter case. These analyses lead to a hypothesis that σy of solid particles is a primary important factor affecting the solid particle bounce at least under the conditions assumed in this study. Further studies are needed to draw more general conclusions under a wide variety of particle materials and impact conditions.
6. CONCLUSIONS
Bounce of high-velocity particles impacting upon a surface are an important process in particle sampling including Aerodyn AMS and other LPIs, and was investigated in detail using the T1990 model and AUTODYN. AN and NaCl were selected as test compounds for the liquid and solid particles, respectively. A semi-empirical model, the T1990 model was used to assess the significance of the plastic deformation of solid particles during the high-velocity impacts. Furthermore, hydrodynamic simulations using AUTODYN were employed to explicitly analyze changes in the shape, temperature, strain, and velocity of these particles upon the high-velocity impact. The AN particle were shown to collapse during impact, as have been suggested by a number of previous studies. Pressure and temperature inside the AN particle did not increase significantly because no elastic deformation occurred. For the NaCl particles, anisotropy of the elastic properties affected the particle adhesion and bounce due to variations in the stress–strain characteristics. For higher σy values, the NaCl particles bounced off the W surface (COR > 0) and rebound velocities were lowered by plastic deformation and fracture inside the particles. ΔT max for these cases reached higher than ∼100°C and were dependent upon the values of σy and Vi used for the simulations. NaCl particles with lower σy adhered to the W surface (COR ∼ 0) because of large kinetic energy losses inside the particles. These results suggest that a single impact process is not sufficient for breaking and capturing all of solid crystalline particles. Furthermore, the effect of elastic anisotropy and brittleness could be a significant consideration for impact process involving other atmospherically important particles. When particles impact upon a surface, values of σy at the contact area can be stochastically different among particles because of their anisotropic elasticity. Our simulation results show for the first time that anisotropy of the elastic properties of ionic crystalline materials is a possible cause for nonzero collection efficiencies of solid particles. It is difficult to investigate material properties of solid particles (e.g., elasticity, brittleness, and crystal state) only by measuring COR or collection efficiencies.
Supplemental Information.zip
Download Zip (47.6 KB)Acknowledgments
This study was funded by the SENTAN program of the Japan Science and Technology Agency (JST). The authors would like to thank the editor and two anonymous reviewers for their valuable comments and suggestions to improve the quality of this article.
[Supplementary materials are available for this article. Go to the publisher's online edition of Aerosol Science and Technology to view the free supplementary files.]
Notes
a“Note” depicts general features for each simulation run.
bThe unit of “Fracture” is the percent by mass of fractured fraction of particles.
REFERENCES
- Black , J. T. and Kohser , R. A. 2007 . DeGarmo's Materials and Processes in Manufacturing , 10th ed , Wiely, Hoboken, NJ .
- Chen , S. C. , Tsai , C. J. , Chen , H. D. , Huang , C. Y. and Roam , G. D. 2011 . The Influence of Relative Humidity on Nanoparticle Concentration and Particle Mass Distribution Measurements by the MOUDI . Aerosol Sci. Technol. , 45 ( 5 ) : 596 – 603 .
- Cheng , Y. S. and Yeh , H. C. 1979 . Particle Bounce in Cascade Impactors . Environ. Sci. Tech. , 13 : 1392 – 1396 .
- Dahneke , B. 1971 . The Capture of Aerosol Particles by Surfaces . J. Colloid Interface Sci. , 37 : 342 – 353 .
- Dahneke , B. 1973 . Measurements of Bouncing of Small Latex Spheres . J. Colloid Interface Sci. , 45 : 584 – 590 .
- Dahneke , B. 1975 . Further Measurements of the Bouncing of Small Latex Spheres . J. Colloid Interface Sci. , 51 : 58 – 65 .
- Davison , L. and Shahinpoor , M. 1997 . High-Pressure Shock Compression of Solids III , Springer, New York .
- DeCarlo , P. , Worsnop , D. R. , Slowik , J. G. , Davidovits , P. and Jimenez , J. L. 2004 . Particle Morphology and Density Characterization by Combined Mobility and Aerodynamic Diameter Measurements . Aerosol Sci. Technol. , 38 ( 12 ) : 1185 – 1205 .
- Derjaguin , B. V. , Muller , V. M. and Mikhovich , N. S. 1987 . Effect of Contact Deformations on the Adhesion of Particles . J. Colloid Interface Sci. , : 118553 – 563 .
- Jayne , J. T. , Leard , D. C. , Zhang , X. F. , Davidovits , P. , Smith , K. A. Kolb , C. E. 2000 . Development of an Aerosol Mass Spectrometer for Size and Composition Analysis of Submicron Particles . Aerosol Sci. Technol. , 33 ( 1–2 ) : 49 – 70 .
- Katayama , M. , Toda , S. and Kibe , S. 1997 . Numerical Simulation of Space Debris Impacts on the Whipple Shield . Acta Aston. , 12 : 859 – 869 .
- Keskinen , J. , Pietarinen , K. and Lehtimäki , M. 1992 . Electrical Low Pressure Impactor . J. Aerosol Sci. , 23 : 353 – 360 .
- Klinkov , S. V. , Kosarev , V. F. and Rein , M. 2005 . Cold Spray Deposition: Significance of Particle Impact Phenomena . Aerospace Sci., Technol. , 9 : 582 – 591 .
- Lightstone , J. M. , Onasch , T. B. , Imre , D. and Oatis , S. 2000 . Deliquescence, Efflorescence, and Water Activity in Ammonium Nitrate and Mixed Ammonium Nitrate/Succinic Acid Microparticles . J. Phys. Chem. A , 104 ( 41 ) : 9337 – 9346 .
- Liu , P. , Ziemann , P. J. , Kittelson , D. B. and McMurry , P. H. 1995 . Generating Particle Beams of Controlled Dimensions and Divergence: II. Experimental Evaluation of Particle Motion in Aerodynamic Lenses and Nozzle Expansions . Aerosol Sci. Technol. , 22 : 314 – 324 .
- Marple , V. A. , Rubow , K. L. and Behm , S. M. 1991 . A Microorifice Uniform Deposit Impactor (MOUDI), Description, Calibration, and Use . Aerosol Sci. Technol. , 14 : 434 – 446 .
- Matthew , B. M. , Middlebrook , A. M. and Onasch , T. B. 2008 . Collection Efficiencies in an Aerodyne Aerosol Mass Spectrometer as a Function of Particle Phase for Laboratory Generated Aerosols . Aerosol Sci. Technol. , 42 : 884 – 898 .
- McFarland , A. R. and Zeller , H. W. 1963 . Study of a Large-Volume Impactor for High-Altitude Aerosol Collection . General Mills, Inc., Electronics Division, Rep. 2391, Contract AT(11–1)-401. Available online at: http://www.osti.gov/energycitations/product.biblio.jsp?osti_id=4685775
- Murri , W. J. and Anderson , G. D. 1970 . Hugoniot Elastic Limit of Single-Crystal of Sodium Chloride . J. Appl. Phys. , 41 ( 8 ) : 3521 – 3525 .
- Pak , S. S. , Liu , B. Y. H. and Rubow , K. L. 1992 . Effect of Coating Thickness on Particle Bounce in Inertial Impactors . Aerosol Sci. Technol. , 16 : 141 – 150 .
- Paw , UK. T. 1983 . The Rebound of Particles from Natural Surfaces . J. Colloid Interface Sci. , 93 : 442 – 452 .
- Rao , A. K. and Whitby , K. T. 1978a . Non-Ideal Collection Characteristics of Inertial Impactors-I. Single-Stage Impactors and Solid Particles . J. Aerosol. Sci. , 9 ( 2 ) : 77 – 86 .
- Rao , A. K. and Whitby , K. T. 1978b . Non-Ideal Collection Characteristics of Inertial Impactors-I. Cascade Impactors . J. Aerosol. Sci. , 9 ( 2 ) : 87 – 100 .
- Richardson , C. B. and Hightower , R. L. 1987 . Evaporation of Ammonium Nitrate Particles . Atmos. Environ. , 21 ( 4 ) : 971 – 975 .
- Rogers , L. N. and Reed , J. 1984 . The Adhesion of Particles Undergoing an Elastic-Plastic Impact with a Surface . J. Phys. D: Appl. Phys. , 17 : 577 – 689 .
- Steinberg , D. J. , Cochran , S. G. and Guinan , M. W. 1980 . A constitutive Model for Metals at High-Strain Rates . J. Appl. Phys. , 51 ( 3 ) : 1498 – 1504 .
- Takegawa , N. , Miyazaki , Y. , Kondo , Y. , Komazaki , Y. , Miyakawa , T. and Jimenez , J. L. 2005 . Characterization of an Aerodyne Aerosol Mass Spectrometer (AMS): Intercomparison with other Aerosol Instruments . Aerosol Sci. Technol. , 39 : 760 – 770 .
- Tang , I. N. 1980 . “ Deliquescence Properties and Particle Size Change of Hygroscopic Aerosols, ” . In Generation of Aerosols and Facilities for Exposure Experiments , Edited by: Willeke , K. Ann Arbor , MI : Ann Arbor Science Publishers, Inc .
- Tanimura , S. , Hayashi , H. and Yamamoto , T. 2006 . A Practical Constitutive Model Covering a Wide Range of Strain Rates and a Large Region of Strain . J. Phys. IV France , 134 : 55 – 61 .
- Tsai , C. J. and Cheng , Y. 1995 . Solid Particle Collection Characteristics on Impaction Surfaces of Different Designs . Aerosol Sci. Technol. , 23 ( 1 ) : 96 – 106 .
- Tsai , C. J. , Pui , D. Y. H. and Liu , B. Y. H. 1990 . Capture and Rebound of Small Particles Upon Impact with Solid Surfaces . Aerosol Sci. Technol. , 12 ( 3 ) : 497 – 507 .
- Virtanen , A. , Joutsensaari , J. , Koop , T. , Kannosto , J. , Yli-Pirilä , P. and Leskinen , J. 2010 . An Amorphous Solid State of Biogenic Secondary Organic Aerosol Particles . Nature , 467 : 824 – 827 .
- Zhang , X. , Smith , K. A. , Worsnop , D. R. , Jimenez , J. L. , Jayne , J. T. and Kolb , C. E. 2004 . Numerical Characterization of Particle Beam Collimation: Part II Integrated Aerodynamic-Lens-Nozzle System . Aerosol Sci. Technol. , 38 : 619 – 638 .