Abstract
To calibrate a newly developed condensation particle counter, samples of known chemical composition are needed as the chemistry plays a role in the activation process. For that, we have built a calibration setup and produced ammonium sulfate, sodium chloride, tungsten oxide, silver, alkyl halide, and ionic liquid clusters down to 1 nm in mobility diameter in positive and negative mode. The chemical composition of most negatively charged clusters was solved using high-resolution mass spectrometer and we identified about 70% of the total signal of the mass spectrometer. For the Airmodus Particle Size Magnifier, which was the instrument to be calibrated, we measured cutoff diameters of 1.1, 1.3, 1.4, 1.6, and 1.6–1.8 nm for negative sodium chloride, ammonium sulfate, tungsten oxide, silver, and positive organics, respectively. From the alkyl halide and ionic liquid experiments, we concluded that the composition plays a bigger role than the charge state of the cluster in the activation process. We also showed that relative humidity of the sample flow can change the detection efficiency of the Particle Size Magnifier, which adds some uncertainties to the measured number concentrations.
Copyright 2013 American Association for Aerosol Research
INTRODUCTION
Recent instrumental development has brought aerosol measurements down to the size of individual molecules and molecular clusters (Gamero-Castoño & Fernandez de la Mora Citation2000; Kulmala et al. Citation2007b; Mirme et al. Citation2007; Sipilä et al. Citation2008; Winkler et al. Citation2008a; Jiang et al. Citation2011; Vanhanen et al. Citation2011). Instruments that can detect even the smallest particles are needed for studying atmospheric nucleation (Kerminen et al. Citation2010), and they can have various applications also in the fields of nanotechnology, medicine, etc. Both calibration of these instruments and heterogenous nucleation experiments require being able to produce sufficient amounts of very small particles with known size, shape, charge, and composition. This is especially important when working with condensation particle counters (CPCs), since the composition and charging state of the particles can affect their activation probability, and thus the cutoff size of the instrument (Hering et al. Citation2005; Petäjä et al. Citation2006; Kulmala et al. Citation2007a; Winkler et al. Citation2008a,Citationb; Iida et al. Citation2009; Wang et al. Citation2010; Hakala et al. Citation2013).
Many difficulties arise when producing calibration particles smaller than about 3 nm in diameter. Different kinds of production methods are used in parallel to cover the size range from below 1 nm up to several nanometers. Due to the lack of reference instruments and size selection methods for neutral particles, the produced particles usually need to be charged before size selection. The electric charge coming from the radioactive sources is transferred through collisions with carrier gas molecules to the sample particles and impurities in the carrier gas. The charged gas molecules and impurities are ions with a mobility diameter of 1–1.5 nm, which are here referred as charger ions. These charger ions usually overlap with the sample in a mobility space, which makes the separation of the charger ions and the sample difficult. Even when using a high-resolution differential mobility analyzer (DMA) for achieving a close to mono-mobile size distribution, the dynamics of the size-selected ions, such as clustering, charge transfer, fragmentation, or evaporation, as well as impurities coming from the setup, can affect the final size distribution entering the instruments (Premnath et al. Citation2011; Attoui et al. Citation2013a,Citationb).
We measured the mass and mobility spectra of different kinds of ions produced in a standard laboratory setup for calibrating aerosol instrumentation in order to evaluate the methods and elucidate some of the problems connected to producing calibration aerosol below 3 nm. Combining a high-resolution DMA and a CPC with very low but adjustable cutoff size (Airmodus A09, Particle Size Magnifier [PSM]; Vanhanen et al. Citation2011) to a high-resolution mass spectrometer (atmospheric pressure interface time-of-flight mass spectrometer [APi-TOF]; Junninen et al. Citation2010) makes it possible to explain some of the observed differences in both the detection efficiency and the activation probability of particles by their mass distribution. Our aim was to produce monodisperse aerosol particles in the sizes below 2 nm for calibrating the PSM. When conducting experiments in this size range, one should be aware that there are several artifacts that can affect the composition and final size distribution of the size-selected ions entering the counter, and thus the resulting activation or detection efficiency. Especially, one should take care of the diffusion losses, cleanliness and material of the tubings (Steiner and Reischl Citation2012) and connectors, and the composition, relative humidity (RH), temperature, and volume flow of the carrier gas. In this study, we characterize our calibration sample ions and show the effect of composition on the detection efficiency. We also show that impurities are present in our calibration setup and how they affect the detection efficiency. Finally, we want to demonstrate for the first time how RH, which is an ambient variable, change the detection efficiency of the PSM for samples smaller than 2 nm.
EXPERIMENT DESCRIPTION
The experimental setup is presented in . Solid ammonium sulfate (Merck 99.99%), sodium chloride (Merck 99.99%), and silver (Goodfellow 99.95%) were evaporated to a nitrogen (5.0 grade) flow of 10–15 Lpm in a tube furnace (Carbolite MTF), then cooled down in a water bath at 0°C and charged with an 241Am charger (Scheibel and Porstendorfer Citation1983). High volume flows were used to minimize the diffusion losses in the tubes and to cool down the sample quickly to prevent the growth of the particles. Tungsten oxide (Goodfellow 99.95%) was produced with a hot-wire generator where 0.4-mm-thick tungsten wire is heated with a high current to evaporate particles to the bypass flow (Peineke et al. Citation2006). Tungsten oxide was also evaporated to nitrogen flow and we observed the residual oxygen in the carrier gas to be sufficient to oxidize the tungsten. Alkyl halides (tetra heptyl ammonium bromide [THABr]/propyl ammonium iodide [TPAI]/butyl ammonium bromide [TBABr]; Ude and Fernández de la Mora Citation2005) solved in pure methanol and ionic liquids (1-ethyl-3-methyl imidazolium tetraboroflorate [EMIBF4]/1-ethyl-3-methyl imidazolium nitrodicyanide [EMIN(CN)2]; e.g., Ku and Fernandez de la Mora Citation2009) solved in acetonitrile were generated with an electrospray ion source. The tubes used in the furnace were washed with alcohols and milli-Q water and then heated up to 1200°C for several hours to remove possible contaminants evaporating from the walls. Also, the charger and electrospray needles were cleaned chemically to minimize the amount of contaminants. In the experiments with negatively charged particles, we observed that the charge transfer from the charger ions to the sample was complete and no charger ions were mixed with the sample. On positive polarity, this charge transfer was not complete, and charger ions were observed in addition to the sample ions. When the effect of humidity on detection efficiency was tested, the bypass flow of the electrospray was humidified. With ammonium sulfate, the sample RH was controlled by diluting the sample with humid air between the charger and the DMA. The generated particles were classified with a Herrmann-type high-resolution DMA (Herrmann et al. Citation2000), with a resolving power of approximately 20, and run in a closed-loop sheath air to preserve the sample composition. Since the sheath air volume flow in the DMA was high and unknown, the DMA was calibrated after each experiment with THABr-positive monomer. All data were obtained by scanning the high voltage of the DMA between 100 V and 4500 V in approximately 20 min. The slow scan made it possible to get the mobility, detection efficiency, and average mass spectrum, and also the detection efficiency and mass spectrum at a fixed mobility.
In the end, the size-classified sample was distributed to three detectors operated in parallel. The electrometer (TSI 3068B) measures the current generated by the charged particles and is used as the concentration reference with an inlet flow rate of 5–8 Lpm, depending on the experiment. The PSM activates the particles by mixing a flow saturated with diethylene glycol (DEG; Iida et al. Citation2009) with the sample flow. After the mixing, the sample particles grow to a mean diameter of about 90 nm in the growth section and are then detected with a conventional CPC. The cutoff diameter of the PSM can be varied by changing the saturator volume flow, which enables one to measure the size distribution of the sample between approximately 1 and 2 nm. In this study, we restrict to constant saturator flow of 1 Lpm to investigate the response of the PSM to various ions rather than the PSM scan performance. The third detector, the APi-TOF, is a mass spectrometer that is equipped with an atmospheric pressure interface that can sample from atmospheric pressure and zero-potential environment. The mass spectrometer can detect clusters up to 6000 Th with a mass resolution of 5000, which makes it possible to identify the chemical composition of the clusters lighter than approximately 1000 Th by their exact mass. For example, the charged sulfuric acid monomer has an exact mass of 96.960, and the mass defect −0.040 Th to the unit mass 97 Th can be detected. Complex data from the instrument were mass calibrated using known mass peaks by an empirical equation (calibration means relating measured time of flight of ions to their mass), explained more in detail by Junninen et al. (Citation2010). Instruments’ mass accuracy is better than 10 μTh/Th (uncertainty at mass 100 Th is 0.001 Th, and at mass 1000 Th is 0.01Th). In addition to the exact mass, also isotopic patterns are used for identification, especially sulfur, chlorine, silver, and bromide have a very distinct isotopic patterns. All data analysis of APi-TOF data were performed by the MATLAB toolbox, tofTools (Junninen et al. Citation2010).
RESULTS AND DISCUSSION
Chemical Composition
The first step was to identify the chemical composition of the samples used. Most clusters were identified based on their exact mass and isotopic pattern. Most clusters form distinct series where the clusters grow by an additional neutral molecule, e.g., NaCl, which are noticeable when the mass defect of the clusters is plotted against the integer number of mass. These three, together with the information of the used substance, reveal in most cases the number of elements in the cluster from where the chemical composition can be constructed. Complete lists of the identified clusters are given in the online supplementary material. presents the mass and mobility spectra of the three compounds used in the experiments in negative polarity: ammonium sulfate, sodium chloride, and tungsten oxide. The overlaid electrometer spectra are done by matching the measured mass and mobility of the clusters with a density that fits to the Stokes–Millikan equation. For example, for ammonium sulfate, the observed density shifts from 1.57 to 1.35 g/cm3 between 1 and 1.7 nm. The conversion was done to show the mass and mobility signal of the clusters simultaneously and is not further discussed here. We also want to emphasize in that the electrometer and mass spectrometer signal was not disturbed by the charger ions, thus allowing analyzing also the smallest of generated clusters.
FIG. 2 The average mass spectra of negative ammonium sulfate, sodium chloride, and tungsten oxide with the overlaid electrometer signal. The chemical composition of the clusters is given in the text.
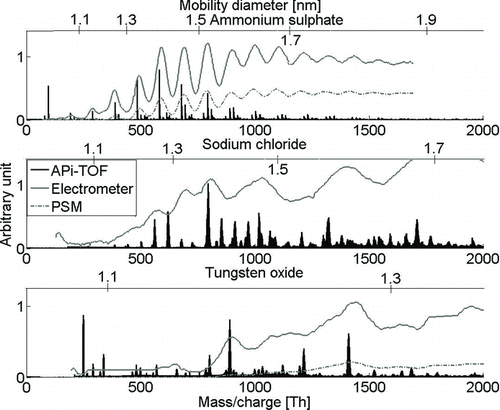
The spectrum from heated ammonium sulfate was identified to contain a series of (HSO4) x (NH3) y SO– 4 and (HSO4) x (NH3) y SO– 5. For all clusters, SO– 4 was the main charge carrier, whereas SO– 5 played a role for the clusters with 1–4 bisulfates. Larger clusters (x ≥ 5) started to include impurities, but the source was unknown. It might have been, for example, the sample, the filter of the sheath gas, tube surfaces, radioactive charger, or the nitrogen gas. C2H7N, dimethyl amine, was observed to attach to the cluster though the intensity of the contaminated clusters was very low. When the sample was more contaminated (), organic impurity C6H8O2 was present in the tetramer and larger clusters with high intensity, although the exact chemical composition of the impurity remains uncertain. Ammonia-to-bisulfate ratio for all clusters was well below 1 throughout the spectrum, e.g., the cluster of 10 bisulfates had the highest intensity with only two ammonias attached to it. The smallest charged bisulfate cluster able to hold one ammonia molecule was the tetramer. Similar behavior has also been observed in the laboratory or chamber experiments, although in these experiments (Junninen et al. Citation2010; Bzdek et al. Citation2011; Kirkby et al. Citation2011), the cluster-forming molecule has been sulfuric acid. The mobility spectrum follows the structure of the mass spectrum, and if calibration experiments with ammonium sulfate are conducted, these peaks in the mobility spectrum are a sign of a well-generated sample. For example, the insufficient resolution of the DMA and impurities in the sample might smear out the peak structure. The observation that ammonium sulfate forms bisulfate clusters in the sub-2-nm range makes it a suitable calibration sample for sulfuric acid experiments as the chemical composition is quite similar (Kirkby et al. Citation2011).
The positive ammonium sulfate spectrum had cluster series (HSO4) x (NH3) y H2SO4 + where ammonia-to-bisulfate ratio was around 1, whereas in the more contaminated experiment, the ratio was the same as with negatively charged clusters. Below about 700 Th (mobility diameter 1.5 nm), the charger ions generated in the 241Am source were present in positive polarity, with all used materials generated with tube furnace and hot-wire generator. Their composition is not known, but from their mass defect (), it is known that they are mostly organic compounds. In negative polarity, we also detected doubly and triply charged clusters that were not observed in positive polarity (). The mass spectrum of sodium chloride on both polarities was mostly explained by series (NaCl) x (C4H10O3) y Cl– and (NaCl) x (C4H10O3) y Na+ in negative and positive polarity, respectively. Again, the chemical composition of the organic molecule is uncertain. In negative polarity, all clusters were observed to carry zero or one C4H10O3, except (NaCl)10Cl– with zero to four impurity molecules, whereas all positively charged clusters were observed to carry zero to six impurity molecules (). The clear difference in the chemical composition between clusters of different polarities has a major effect on PSM detection efficiency, which is shown later on. There is also some difference between the measured mobility and mass distributions, e.g., around 700 Th, which could be due to clusters (NaCl)11Cl– and (NaCl)12Cl– (mass peaks with low signal intensity relative to the electrometer) being more unstable than (NaCl)9Cl– and (NaCl)10Cl–. Thus, NaCl or (NaCl)2 would be evaporated after the DMA or fragmented in APi-TOF and we would get relatively high-mass signals compared with the electrometer for clusters (NaCl)9Cl– and (NaCl)10Cl–. The mass spectrum used in the detection efficiency experiment is different from the one presented here, with two slight differences that are mentioned there.
FIG. 3 Mobility diameter versus mass of negatively charged ammonium sulfate. Bands of doubly and triply charged clusters are observed. (Color figure available online.)
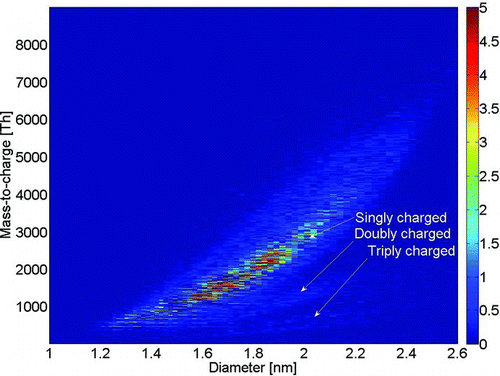
The negative tungsten oxide spectrum consisted of clusters W x O y H z (C4H8O2)– i , where the x-to-y ratio was approximately 1/3 and z was 0–3, and i was 0–2. The clusters with, e.g., two W atoms did not have a fixed number of O atoms, but it varied between 6 and 8. The formula holds quite well up to 1000 Th, after which the fraction of identified clusters gets lower. The mass spectrum on the positive tungsten oxide () up to 1000 Th contained unknown contamination, which made the identification of heavier tungsten oxide clusters very difficult, and in practice, impossible.
FIG. 4 Mass spectra of ammonium sulfate, sodium chloride, tungsten oxide, and silver with a mobility diameter of 1.26 nm in negative polarity. Fragmentation and different apparent densities of clusters are observed.
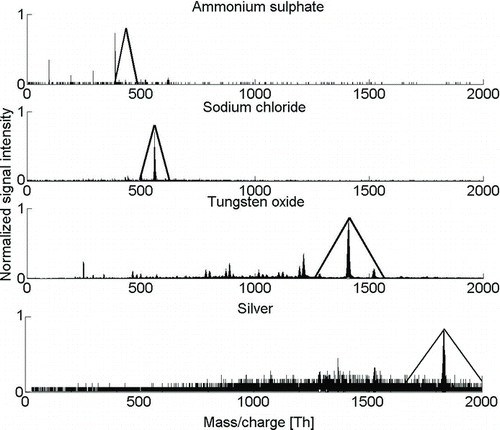
Silver was the most difficult to generate in this size range using the tube furnace, and the worst suitable for calibration experiments in terms of known chemical composition. The silver clusters that were identified in negative polarity were Ag– 7, Ag– 17, and Ag– 19, which were also found by Katakuse et al. (Citation1986). In positive polarity, we identified three series: H x Ag y (charge carrier)+, where y was odd when x was 0, and even when x was 1, and the charge carriers had masses 224, 236, and 340 Th. The rest of the peaks in negative polarity had an isotopic pattern of silver, though they were not identified, which was also the case in positive polarity after the charger ion range. The positive mass defect of the unknown clusters, however, shows that the silver clusters are contaminated with organics. The fact that nearly all silver atoms were clustered with organics suggests that pure silver is very difficult to use as a calibration substance in this size range.
To summarize, clusters of the evaporated material were identified in all the mass spectra, although getting a spectrum completely without impurities proved to be extremely difficult. Ammonium sulfate did not produce ammonium sulfate clusters due to thermal decomposition, but instead formed ammonium bisulfate clusters. Each tested substance contained impurities to the extent that affected the measurements. We can conclude that when studying properties of nanoparticles with different chemical composition in the sub-3-nm range, a high-resolution mass spectrometer is an essential tool for identifying the chemical composition and verifying the purity of the sample.
Mobility Classification
To illustrate the resolution of the DMA on a mass scale and to verify its ability to select clusters from the mobility spectrum, the mass spectra of ions with fixed a mobility diameter of 1.26 nm for ammonium sulfate, sodium chloride, and tungsten oxide is presented in . The theoretical DMA transfer functions are calculated using densities of 1.5, 2.0, and 4.9 g/cm3, respectively. For the pure silver clusters, we observed a density of 6.0 g/cm3. The appearance of smaller (HSO4)0–2SO– 4 clusters when mobility selecting (HSO4)3SO– 4 cluster indicates fragmentation after DMA, probably in the mass spectrometer, and consecutively stability of the clusters.
With the same mobility diameter of 1.26 nm, sodium chloride makes a cluster (NaCl)9Cl–. When compared with ammonium sulfate, less fragmentation is observed, which indicates that this cluster is more stable. For negative tungsten oxide, the mass signal is spread to many clusters due to fragmentation and contaminated clusters with lower observed density but which have the same mobility. The chosen clusters W6O19H1–3 co-appear with various clusters with one to four tungsten atoms. Silver shows slightly similar behavior as tungsten oxide, as there is one main peak, which is Ag– 17, and many lighter clusters that are silver ions clustered with some organics.
In the case of ammonium sulfate, we observed fragmentation affecting the mobility–mass spectrum, because for sodium chloride, no or little fragmentation is observed, and for tungsten oxide and silver spectra, many peaks are observed due to cluster shape, density, and fragmentation. For positively charged clusters in the sub-1.5-nm size range, we observed practically no correlation between mass and mobility, which we could not explain properly. More studies are needed to fully understand the relations between mass, mobility, and composition of ions, and the signal of mass spectrometer in terms of fragmentation and transmission in the sub-3-nm size range.
Sample Contamination
We calculated the purity of the mass spectra showed in as a function of mobility diameter (). Purity is defined here as the signal of an identified pure cluster signal, which includes also the signal of the fragments divided by the total signal at the selected size. Electrical background noise of the mass spectrometer is not subtracted as it is only a very small fraction of the total signal (corresponds to 0.5 ions/cm3 according to Junninen et al. [Citation2010]). We however observed higher noise in the mass regions with higher signal, which will add some uncertainty and lower the observed purities. The peak structure in the ammonium sulfate purity spectrum as a function of mobility diameter corresponds to the same structure in the mobility spectrum in . This is because the ammonium sulfate signal relative to noise and unidentified signal is higher in those peaks than between the peaks. The smallest sodium chloride clusters with a mobility diameter of 1.2 nm were contaminant-free, but with increasing size, the degree of contaminants in clusters and the fraction of unknown clusters increase. Tungsten oxide shows interesting behavior, as between 1.2 and 1.3 nm, the clusters were more contaminated than in smaller and larger sizes. Low purity for the smallest clusters of sodium chloride and tungsten oxide is explained by the absence of generated clusters, whereas after 1.3 nm, the purity is lowered due to unidentified clusters. This holds especially for tungsten oxide as it is about three times heavier than sodium chloride, which makes the cluster identification more difficult because of the transmission and resolution of the APi-TOF. The fraction of the identified clusters’ signal reaches approximately 70% of the total signal with each substance, whereas the purities at 1.5 nm are 69%, 39%, and 22% for ammonium sulfate, sodium chloride, and tungsten oxide, respectively. For positively charged clusters, we did not conduct the calculations because of the poor mass–mobility relation.
Cutoff Diameter
So far, we have shown that negatively charged ammonium sulfate, sodium chloride, and tungsten oxide samples are relatively well characterized, whereas all the other furnace-generated samples are not necessarily suitable for calibration experiments in the sub-2-nm range, since their composition is mostly organic. Cutoff diameters are here given as the diameter where the detection efficiency is half of the observed maximum detection efficiency, since we observed saturation detection efficiencies between 0.6 and 0.8. Because we cannot explain why the detection efficiency does not reach 90% of the electrometer signal where conventional CPCs saturate, we have not scaled the detection efficiencies in any way.
The detection efficiency for clusters of different chemical composition was measured for ammonium sulfate, sodium chloride, tungsten oxide, and silver, and is presented in . The RH of the saturator and sample flow of the PSM were kept close to zero. We observed significantly lower detection efficiencies for the organics, which are in this case positively charge clusters, than for the rest of the samples. The detection efficiencies for the positive samples were more or less the same, with cutoff diameter of 1.6–1.8 nm, indicating similar chemical composition. In negative polarity, the highest detection efficiency is observed for sodium chloride clusters with a mobility diameter below 2 nm, whereas the lowest detection efficiency is observed for silver. The lowest detection efficiency of silver can be accounted to the amount of organics in the clusters. The highest detection efficiency is observed for sodium chloride, which has an anion–cation structure, different from all the others. The two points of the sodium chloride detection efficiency curve, which are significantly lower than the others, are partly organics, which is confirmed by the APi-TOF. Sodium chloride is water soluble like DEG, which might explain its better detection efficiency. Tungsten oxide and ammonium sulfate show somewhat similar detection efficiencies, as well as quite similar structures: S or W surrounded by O. Ammonium sulfate is also water soluble and the clusters contain less organic impurities than tungsten oxide, as shown in , which might explain why it is also detected better than tungsten oxide. However, within this study, we cannot explain explicitly what causes the differences in the detection efficiencies of ammonium sulfate, sodium chloride, tungsten oxide, and organics. The cutoff diameters for negatively charged clusters in dry conditions for sodium chloride, ammonium sulfate, tungsten oxide, and silver were 1.1, 1.3, 1.4, and 1.6 nm, respectively. The saturation detection efficiency also varies significantly, but due to lack of composition information for the larger sizes, we cannot explain the differences.
Effect of Charge State
To investigate the effect of charge state of the clusters on the detection efficiency of the PSM, we generated alkyl halides and ionic liquids with electrospray. Charge state of particles has been earlier claimed to affect the activation efficiency of a CPC in small sizes (Winkler et al. 2008a). Clusters of both the substances form ion pairs with a positive or negative charge carrier, e.g., THABr forms clusters (C28H60NBr) x C28H60N+ and (C28H60NBr) x Br– on positive and negative polarity, respectively. Therefore, THABr dimers are (C28H60NBr)1C28H60N+ and (C28H60NBr)1Br– on positive and negative polarity, respectively. Alkyl halides and imidazolium clusters are chosen for this analysis, as we were able to generate rather clean mobility peaks on both polarities () and study whether consistent activation behavior as a function of charge state is observed. In these experiments, the RH of the PSM saturator flow was not controlled as the measurements were conducted with an open-loop sheath air. This adds some uncertainty, as discussed in the next paragraph, but the experiments were conducted for each substance during 1 h, so detection efficiencies for both polarities of each substance are comparable. The results are shown in . From the results, we can conclude that as a function of mobility diameter, the negative clusters of alkyl halides activate slightly better than the positive ones. However, the positive 1-ethyl-3-methyl imidazolium (EMI) clusters activate better than the negative EMI clusters, which contradicts with all other substances used in the experiments and clearly indicates strong composition dependence for the activation efficiency. This observation claims that one cannot make any conclusion on the effect of charge state on the activation efficiency without directly measuring the composition of the sample. Therefore, we conclude that the chemical composition plays a bigger role on activation than the charge state in the sub-2-nm range. To distinguish the effect of charge state from composition, one needs a cluster with fixed composition but either –1 or +1 charge state, which we did not have in our experiments.
Effect of Sample Flow Humidity
Finally, we measured detection efficiencies as a function of inlet flow RH for THABr positive monomer and dimer with mobility diameters of 1.47 and 1.78 nm, respectively, and for negatively charged ammonium sulfate between 1 and 4 nm (). Since the PSM detects ammonium sulfate better than THABr, we adjusted the PSM temperatures so that we could observe the effect of RH on both substances. For both samples, we observed clear enhancement with higher RH in the detection efficiency. The residence time between the sample humidification and DMA was very short, and we did not detect any significant mobility change in the clusters due to the change in RH. In the experiments, the growth section temperature of the PSM was set to 6.5°C, and if the sample temperature of 23°C is assumed with an RH of 40%, the humidity will not exceed 100% in the mixing section, since the sample is further diluted with the dry saturator flow. This provide a hint that the enhancement is due to the water–DEG interaction, although the effect needs further research. The observation that detection efficiency is RH dependent must be taken into account when calibrations are conducted, and also emphasizes the importance of closed-loop sheath air of the DMA, because with open-loop sheath air, the RH of the sample varies with the humidity of the laboratory.
ATMOSPHERIC IMPLICATIONS
If accurate number concentration of sub-2-nm particles is to be measured, careful substance specific calibrations are needed, preferably in parallel with a mass spectrometer. As shown in , , and , the detection efficiency of 1.3-nm particles can vary from 0 to 0.5, depending on the chemical composition and RH, which means that without proper calibration also, the measured number concentration and cutoff diameter have very high uncertainties. Even if all but sample aerosol could be controlled, the problem of sampling unknown aerosol is that the composition is not known, which in case leads to an ill-defined cutoff diameter. This is because the chemical effects are more important than the mobility diameter, which is used as it is the only way to size select particles. This is to be considered when sub-2-nm CPCs are compared; PSM is used in the scanning mode or cutoff diameters are stated along with CPC measurements in the sub-2-nm range. For measurements in different locations, the changes in cutoff diameters should be considered as the humidity can change a lot between day and night and from place to place.
CONCLUSIONS
We have shown that the modern CPCs need calibrations that are specific to the conditions and preferably also to the sample composition in order to reduce the uncertainties of the measured number concentrations. For this, we generated different samples smaller than 3 nm in the mobility diameter, characterized their chemical composition, and tested the response of the PSM to the generated samples. The composition of the furnace-generated positively charged clusters below 1.5 nm in our system was mostly organic and we suggest that positively charged samples generated using tube furnace or hot-wire generator are not used without a mass spectrometer. We found significant differences in the detection efficiencies for different chemical substances and observed the cutoff diameters of 1.1, 1.3, 1.4, 1.6, and 1.6–1.8 nm for negatively charged sodium chloride, ammonium sulfate, tungsten oxide, silver, and organics, respectively. In addition, we showed that changes in the RH will change the cutoff diameter of the PSM, which is to be considered when using the PSM in ambient measurements. We conclude that doing experiments with the PSM for particles smaller than 2 nm in laboratory or ambient conditions requires control and knowledge of conditions and specific calibrations for the prevailing conditions.
Supplementary Material.zip
Download Zip (338.5 KB)Acknowledgments
This work was partially funded by the Academy of Finland (1251427, 1139656, 141451, 133872, Finnish Centre of Excellence 1141135) and European Research Council (ATMNUCLE).
[Supplementary materials are available for this article. Go to the publisher's online edition of Aerosol Science and Technology to view the free supplementary files.]
REFERENCES
- Attoui , M. , Fernandez-García , J. , Cuevas , J. , Vidal , G. and Fernandez de la Mora , J. 2013 . Charge Evaporation from Nanometer Polystyrene Aerosols . J. Aerosol Sci. , 55 : 149 – 156 .
- Attoui , M. , Paragano , M. , Cuevas , J. and Fernandez de la Mora , J. 2013 . Tandem DMA Generation of Strictly Monomobile 1–3.5 nm Particle Standards . Aerosol Sci. Tech. , doi: 10.1080/02786826.2013.764966
- Bzdek , B. R. , Ridge , D. P. and Johnston , M. V. 2011 . Amine Reactivity with Charged Sulfuric Acid Clusters . Atmos. Chem. Phys. , 11 : 8735 – 8743 .
- Gamero-Castano , M. and Fernandez de la Mora , J. 2000 . A Condensation Nucleus Counter (CNC) Sensitive to Singly Charged Sub-Nanometer Particles . J. Aerosol Sci. , 31 : 757 – 772 .
- Hakala , J. , Manninen , H. E. , Petäjä , T. and Sipilä , M. 2013 . Counting Efficiency of a TSI Environmental Particle Counter Monitor Model 3783 . Aerosol Sci. Tech. , doi: 10.1080/02786826.2013.766666
- Hering , S. , Stolzenburg , M. R. , Quant , F. R. , Oberreit , D. R. and Keady , P. B. 2005 . A Laminar-Flow, Water-Based Condensation Particle Counter (WCPC) . Aerosol Sci. Tech. , 39 : 659 – 672 .
- Herrmann , W. , Eichler , T. , Bernardo , N. and Fernández de la Mora , J. 2000 . Turbulent Transition Arises at Reynolds Number 35,000 in a Short Vienna Type DMA with a Large Laminarization Inlet . Abstract AAAR Conference, 15B5
- Iida , K. , Stoltzenburg , M. R. and McMurry , P. H. 2009 . Effect of Working Fluid on Sub-2 nm Particle Detection with a Laminar Flow Ultrafine Condensation Particle Counter . Aerosol Sci. Tech. , 43 : 81 – 90 .
- Jiang , J. , Chen , M. , Kuang , C. , Attoui , M. and McMurry , P. H. 2011 . Electrical Mobility Spectrometer Using a Diethylene Glycol Condensation Particle Counter for Measurement of Aerosol Size Distributions Down to 1 nm . Aerosol Sci. Tech. , 45 : 510 – 521 .
- Junninen , H. , Ehn , M. , Petäjä , T. , Luosujärvi , L. , Kotiaho , T. Kostiainen , R. 2010 . A High-Resolution Mass Spectrometer to Measure Atmospheric Ion Composition . Atmos. Measure. Tech. , 3 : 1039 – 1053 .
- Katakuse , I. , Ichihara , T. , Fujita , Y. , Matsuo , T. , Sakurai , T. and Matsuda , H. 1986 . Mass Distributions of Negative Cluster Ions of Copper, Silver, and Gold . Int. J. Mass Spectrom. Ion Processes , 74 : 33 – 41 .
- Kerminen , V.-M. , Petäjä , T. , Sipilä , M. , Vehkamäki , H. , Manninen , H. E. Nieminen , T. 2010 . Atmospheric Nucleation: Highlights of the EUCAARI Project . Atmos. Chem. Phys. Disc. , 10 : 16497 – 16549 .
- Kirkby , J. , Curtius , J. , Almeida , J. , Dunne , E. , Duplissy , J. Ehrhart , S. 2011 . Role of Sulphuric Acid, Ammonia and Galactic Cosmic Rays in Atmospheric Aerosol Nucleation . Nature , 476 : 429 – 433 .
- Ku , B. K. and Fernandez de la Mora , J. 2009 . Relation Between Electrical Mobility, Mass, and Size for Nanodrops 1–6.5 nm in Diameter in Air . Aerosol Sci. Tech. , 43 : 241 – 249 .
- Kulmala , M. , Mordas , G. , Petäjä , T. , Grönholm , T. , Aalto , P. P. Vehkamäki , H. 2007a . The Condensation Particle Counter Battery (CPCB): A New Tool to Investigate the Activation Properties of Nanoparticles . J. Aerosol Sci. , 38 : 289 – 304 .
- Kulmala , M. , Riipinen , I. , Sipila , M. , Manninen , H. E. , Petäjä , T. Junninen , H. 2007b . Towards Direct Measurement of Atmospheric Nucleation . Science , 318 : 89 – 92 .
- Mirme , A. , Tamm , E. , Mordas , G. , Vana , M. , Uin , J. Mirme , S. 2007 . A Wide-Range Multi-Channel Air Ion Spectrometer . Boreal Environ. Res. , 12 : 247 – 264 .
- Peineke , C. , Attoui , M. B. and Schmidt-Ott , A. 2006 . Using a Glowing Wire Generator for Production of Charged, Uniformly Sized Nanoparticles at High Concentrations . J. Aerosol Sci. , 37 : 1651
- Petäjä , T. , Mordas , G. , Manninen , H. , Aalto , P. P. , Hameri , K. and Kulmala , M. 2006 . Detection Efficiency of a Water-Based TSI Condensation Particle Counter 3785 . Aerosol Sci. Tech. , 40 : 1090 – 1097 .
- Premnath , V. , Oberreit , D. and Hogan , C. J. Jr. 2011 . Collision-Based Ionization: Bridging the Gap Between Chemical Ionization and Aerosol Particle Diffusion Charging . Aerosol Sci. Tech. , 45 : 712 – 726 .
- Scheibel , H. G. and Porstendorfer , J. 1983 . Generation of Monodisperse Ag- and NaCl-Aerosols with Particle Diameters Between 2 and 300 nm . J. Aerosol Sci. , 14 : 113 – 126 .
- Sipilä , M. , Lehtipalo , K. , Kulmala , M. , Petäjä , T. , Junninen , H. Aalto , P. P. 2008 . Applicability of Condensation Particle Counters to Measure Atmospheric Clusters . Atmos. Chem. Phys. , 8 : 4049 – 4060 .
- Steiner , G. and Reischl , G. 2012 . The Effect of Carrier Gas Contaminants on the Charging Probability of Aerosols Under Bipolar Charging Conditions . J. Aerosol Sci. , 54 : 21 – 31 .
- Ude , S. and Fernández de la Mora , J. 2005 . Molecular Monodisperse Mobility and Mass Standards from Electrosprays of Tetra-Alkyl Ammonium Halides . J. Aerosol Sci. , 36 : 1224 – 1237 .
- Vanhanen , J. , Mikkilä , J. , Lehtipalo , K. , Sipilä , M. , Manninen , H. E. Siivola , E. 2011 . Particle Size Magnifier for Nano-CN Detection . Aerosol Sci. Tech. , 45 : 533 – 542 .
- Wang , X. , Caldow , R. , Sem , G. J. , Hama , N. and Sakurai , H. 2010 . Evaluation of a Condensation Particle Counter for Vehicle Emission Measurement: Experimental Procedure and Effects of Calibration Aerosol Material . J. Aerosol Sci. , 41 : 306 – 318 .
- Winkler , P. M. , Steiner , G. , Vrtala , A. , Vehkamaki , H. , Noppel , M. Lehtinen , K. E. J. 2008a . Heterogeneous Nucleation Experiments Bridging the Scale from Molecular Ion Clusters to Nanoparticles . Science , 7 : 1374 – 1377 .
- Winkler , P. M. , Vrtala , A. and Wagner , P. E. 2008b . Condensation Particle Counting Below 2 nm Seed Particle Diameter and the Transition from Heterogeneous to Homogeneous Nucleation . Atmos. Res. , 90 : 125 – 131 .