Abstract
A microtrap inertial impactor has been developed and characterized for use as an area or personal sampler. The microtrap impactor utilizes a high-density multijet plate to direct airflow and a matched multiwell plate to impact and collect particles for extraction with a reduced pressure drop relative to inertial impactors with fewer jets. Reported here is the characterization of the microtrap impactor using a fluidized bed aerosol generator and a small volume nebulizer to generate particles of Arizona Road Dust, potassium chloride, and oleic acid. Collection efficiency was determined by measuring particle size distributions with an aerodynamic particle sizer. Two geometries of the microtrap were tested suitable for a two-stage coarse particle sampler, with 1–4 μm and a 4–10 μm stages. The 1 μm cut-point microtrap stage has a collection efficiency above 97% for particles greater than 2 μm in diameter (at a 10 L/min flow rate and a pressure drop of 0.12 kPa). This stage's collection efficiency was constant for a period of time up to 10 h under typical ambient conditions without any coating on the impaction surface. The microtrap impactor provides an improvement in area sampling due to its high collection efficiency at a low pressure drop across the device, and its use of an uncoated impaction surface allowing for the extraction and analysis of biological samples.
© 2013 American Association for Aerosol Research
INTRODUCTION
Increased prevalence in diseases like asthma and allergies has been observed in the last four decades (Platts-Mills et al. Citation1997; D’Amato Citation2002; Umetsu et al. Citation2002; Devereux Citation2003). In order to understand the association between diseases and pollutant exposure, it is necessary to properly evaluate personal exposure to such agents (NAEPP/NHLBI Citation1997), including measuring particles in the super-micron range. Common aerosols in that size range include nonviable aeroallergens from cats, dogs, dust mites, and cockroaches (Horner et al. Citation1991; Custovic et al. Citation1997; Montoya and Hildemann Citation1997, Platts Mills et al. Citation1997; Montoya and Hildemann Citation2001) and many viable aerosols such as fungi and bacteria (Dillon et al. Citation1999; Hinds Citation1999). Dividing the thoracic fraction of aerosols (1–10 μm) into respirable and tracheobronchial fractions (1–4 μm and 4–10 μm, respectively) would be especially valuable in personal and area sampling (Cherrie and Aitken 1999). The finer fraction provides a better estimate for the respirable exposure leading to deep lung deposition while the coarser fraction provides information on upper airway and nasal deposition (Lippman Citation2001).
Inertial impactors have long been employed in aerosol sampling; however, they often require long sampling times due to limitations on their sampling rates. Samplers currently available collect airborne particles most often through filtration, and their primary impediment to obtaining the necessary sampling rates are due to the pressure drop across the device. For sample flow rates near 10 L/min, the pressure drop across the filter can be in excess of 1.5 kPa (6 inches of water). Operation also requires a pump weighing 0.5 to 1 kg (including battery), which can have high levels of noise and power consumption (Karlsson et al. Citation2002). For example, the personal environmental monitor from MSP Corp. (model PEM-10–2.5), which collects particles through impaction and filtration has a 2.5 μm size cut at 10 L/min and a pressure drop across the filter of about 3.2 kPa (13 inches of water) (MSP Corporation 2009). To maintain lower pressure drops, many personal samplers are designed to operate at lower samplings rates, which require longer sampling times. The Marple Personal Cascade impactor, for example, operates at a flow rate of 2 L/min with a pressure drop of approximately 0.5 kPa (2 inches of water) for a four-stage configuration that includes an after-filter (Andersen Instruments Citation1999).
Collecting dry solid particles on solid impaction surfaces can be subject to bounce-off and blow-off. Uncollected particles can be impacted on a later collection stage, distorting particle size distribution measurements (Dzubay et al. Citation1976; Markowski Citation1984). The occurrence of particle bounce-off has been found to be also dependent on the nature of the collection substrate (Dzubay et al. Citation1976; Hinds et al. Citation1985). To reduce these events, impactor design is often modified with the use of fibrous filters (Chang et al. Citation1999; Dunbar et al. Citation2005), oiled or greased coatings on flat impaction surfaces (Rao and Whitby Citation1978; Reischl and John Citation1978; Turner and Hering Citation1987), or recessed wells or cavities on the collection surface (Biswas and Flagan Citation1988; Tsai and Cheng Citation1995). The use of oil aids in particle collection not only through impaction but also through capillary action of the oil around the particle (Turner and Hering Citation1987). Characterization of two single-stage impactors, termed the “laboratory impactor” and the “Bureau of Mines impactor” found that the use of either a silicon oil or petroleum jelly impaction surface coating could improve collection efficiency of solid particles from 30–50% to 100% when applied to uncoated glass plates (Rao and Whitby Citation1978). While the use of substrate coatings can improve collection efficiency, they also limit the potential for extraction and analysis of proteins and other biological components (Dunbar et al. Citation2005). Plate coatings are also not ideal for high temperature applications (Biswas and Flagan Citation1988). Consequently, it is sometimes desirable to efficiently collect particles without the use of impaction surface coatings.
The use of conical cavities on impaction surfaces has been shown to improve particle collection efficiency over conventional flat impaction surfaces (Tsai and Cheng Citation1995). Results from the evaluation of a PM1 personal sampler, designed and characterized by Sioutas et al. Citation1999 found that the use of a conical or partially enclosed cavity substrate could increase the collection efficiency of particles larger than 1.2 μm to 80% or higher without the use of coatings (Chang et al. Citation1999). Previous work had shown that applying an oiled coating to a conical cavity-type impaction surface will improve the initial collection efficiency for larger particles; however, an uncoated cavity-type surface will reach the same level of collection efficiency over time and with heavy particle loading (Tsai and Cheng Citation1995). It is important to determine the collection efficiency over longer sampling periods in impactor characterization, as many layers of particles can be loaded onto an impactor substrate during ambient sampling. Collection efficiency may decrease as incoming particles bounce off of previously deposited particles and become re-entrained (Reischl and John Citation1978; Turner and Hering Citation1987; Biswas and Flagan Citation1988). Long-term testing of a particle trap impactor has shown that collection efficiencies remained high over long-term sampling, decreasing only after 24 h of continuous sampling when the cavity was completely filled (Biswas and Flagan Citation1988).
FIG. 1 Computer-aided design (CAD) drawings for components of the microtrap: (a) jet plate, (b) well plate, (c) housing with dimensions in centimeters, and (d) 3D view of housing.
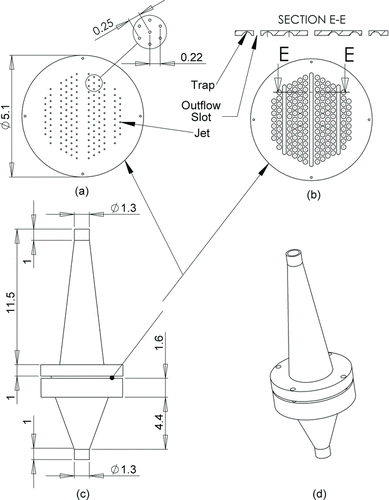
In this article, we report results from the characterization of a new impactor based on a parallel, multijet design incorporating individual particle traps. Experiments conducted include evaluation of the microtrap impactor's collection efficiency for various particle types and flow rates for a single and dual stage collector. The impactor's efficiency over time was also characterized for both uncoated and oil-coated impaction surfaces.
METHODS AND MATERIALS
Instrument Design
The microtrap impactor is a multijet, micro-orifice impactor (Kreisberg and Hering Citation2001) that impacts and collects particles in an array of small hemisphere-shaped wells called microtraps (). The design utilizes chemical etching of thin aluminum plates for both the jet array and the matching particle collection cavities or traps. The jets are arrayed in multiple bands of four jet wide rows with each band in a hexagonal pattern to maximize density with equal jet-to-jet spacing. The jet plate and annular spacer rings were etched from 0.5 mm aluminum sheet while the trap plate was etched from 1.0 mm sheet. The deep etching of the trap plate naturally leads to a highly roughened surface within each trap that aids particle retention. An outflow slot between each band of jets avoids jet-to-jet interference that degrades multijet impactor performance (cross-flow parameter <0.3; Fang et al. Citation1991).
TABLE 1 Stacked two-stage impactor dimensions
Dimensions of the microtrap elements were previously optimized to minimize particle bounce (Kreisberg and Hering Citation2001). Relative to the jet diameter D, the optimized single-stage impactor dimensions were found to be 5D for the jet-to-jet spacing, 4D for the trap diameter, approximately 2D for the trap depth, and 2D for the jet to microtrap plate spacing.
Different microtrap assemblies were tested using an aluminum in-line housing () with a 7° half-angle tapered inlet, two alignment pins, and four clamping screws to secure a stack of jet, spacer, and microtrap plates together. The housing used here is a prototype for characterization of the microtrap, with future plans including development and testing of a reduced size housing suitable for personal sampler use. These components are assembled from top to bottom as follows: jet plate with a maximum of 144 jets (), two ring spacers, and the microtrap plate with a maximum of 144 wells (). For a given total flow rate, the flow rate per jet is adjusted by obscuring jets on the upstream side of the jet plate with tape. To achieve the design's nominal 1.2 μm cut point, the impactor can be operated at 20 L/min with all 144 jets open, 10 L/min with 71 jets open, or 5 L/min with 36 jets open. For these studies, 71-jet and 144-jet configurations were used.
For this study, the microtrap impactor was investigated in a single-stage sampler configuration designed to collect aerosols in the 1–4 μm “respirable” fraction and in a combined two-stage configuration with 1 and 3 μm stages. Dimensions of the microtrap impactor components in the two-stage configuration are given in .
Aerosol Particles
In order to characterize the microtrap impactor, three different particle types were used. These polydisperse particles were chosen to represent the broad range of supra-micron particle types and sizes found in the field. First, AC test dust, a.k.a. “Arizona Road Dust” (AC Delco, Duke Scientific Corp., Palo Alto, CA, USA) with diameters of 1–8 μm and a density of 2.6 grams per milliliter (g/mL) were aerosolized using a fluidized bed aerosol generator (model 3400, TSI Inc., Shoreview, MN, USA). Second, potassium chloride aerosols were generated by nebulizing 3 mL of a 1% or saturated solution using a commercial nebulizer (model 8900, Salter Laboratories, Arvin, CA, USA, or equivalent) at a 2 L/min flow rate. Finally, oleic acid droplets were generated by direct nebulization of 3.5 mL of oleic acid without dilution to maximize size. To achieve the desired flow rates for sampling, dilution air was added to the aerosolized particles as needed. These aerosols were generated and maintained over time at consistent, average concentrations of 550 particles per cubic centimeter, with average geometric mean diameters of 1.0 μm and geometric standard deviations of 1.5 μm. Due to the volume limitations of the nebulizer, and desire to maintain stable particle concentration levels, samples were generated in 20 sample intervals before refilling. To extract particles from the well plate for biological analysis after sampling phosphate buffered saline can be added to each well using a micropipette, and then aspirated and redelivered to the well to foster particle removal. After a final aspiration, the solution can be extracted for analysis.
TABLE 2 Experimental plan for primary microtrap impactor characterization
Impaction Surface Coatings Used
To investigate the effectiveness of applying a coating to minimize particle bounce-off and blow-off of impacted particles, an oleic acid coating was applied to the wells in the impaction plate. The impaction plates were placed in the microtrap impactor and allowed to collect the aerosolized oleic acid (50:50 in water by volume) for 60 min. Loading the well plates in this way assured that the oil was covering the interior of the wells and minimized coating of the flat sections of the collection plate. Because of the high surface tension of oleic acid, coatings are typically applied by coating the surface with a blend of oil and a solvent such as cyclohexane, and drying overnight to evaporate the solvent, leaving behind a uniform thin coat of oil (Rao and Whitby Citation1978; Turner and Hering Citation1987). Oleic acid coatings could also be applied to the microtrap plate in this manner; however, further studies would be needed to determine the effects of residual solvents on biological analyses of collected materials. For these experiments, the use of water was investigated as a way to avoid interference with biological particle analyses.
Experimental Set Up
The experimental set-up is shown in . Clean, dry air was supplied by an in-house source and flow rates were measured using a primary flow calibrator (Gilibrator 2, Sensidyne, St. Petersburg, FL, USA). The aerosols were generated by either a medical nebulizer or a fluidized bed aerosol generator. Dilution airflow rates were adjusted to achieve similar particle concentrations for all aerosols generated. The aerosol was then passed through a custom-made neutralizer unit (Aerosol Dynamics Inc., Berkeley, CA, USA) housing four Polonium 210 Staticmasters (NRD) or a Kr-85 neutralizer (model 3054, TSI Inc., Shoreview, MN, USA). Clean, dry dilution air was added to the aerosol and routed through a small mixing chamber, 30 × 30 × 46 cm. The outlet of the mixing chamber was connected via a “Y” connection to a “bypass” and a “microtrap” lines. The “bypass” line consisted of conductive tubing, leading directly to an aerodynamic particle sizer (APS) Spectrometer (model 3321, TSI Inc., Shoreview, MN, USA). The “microtrap” line routed the aerosol through the microtrap impactor before reaching the APS. The pressure drop across the microtrap impactor device was recorded using a Magnehelic pressure gauge (Dwyer Instruments, Inc., Berkeley, CA, USA). A custom inlet (Aerosol Dynamics Inc., Michigan City, IN, USA) to the APS was used to draw off flow as an azimuthally symmetric sheath in excess of the 5 L/min sample flow drawn by the particle sizer. This excess flow was monitored using a Manostat rotameter (Woodstock, NY, USA), and combined with the fixed APS flow determined the total flow drawn through the microtrap impactor or bypass lines.
EXPERIMENTAL PLAN
The primary characterization experiments were conducted in Troy, NY (RPI entries in ). A total of four sets of experiments were conducted to characterize the original microtrap impactor design. First, the collection efficiency of the impactor was evaluated at flow rates of 7.7, 8.9, and 10 L/min. Second, the collection efficiency was evaluated using three particle types: AC test dust (ACTD), potassium chloride (KCl) particles, and oleic acid (OA) droplets. Third, variations of the collection efficiency over time were measured for sampling periods up to 10 h. Finally, the effects of antibounce coatings on the plates were studied.
To characterize the device under each of these conditions, the experimental setup shown in was used. Microtrap impactor efficiencies were evaluated based on the approach described in John and Kreisberg (Citation1999). Briefly, sequential one-minute samples were measured at the bypass line until particle counts stabilized and the particle concentrations differed by less than 15% between samples. Once the concentrations were deemed stable, sampling commenced. First, a sample from the “bypass” line was recorded. The flow was then diverted to the “microtrap” line, where a one-minute sample was collected. The flow was then returned to the “bypass” line for a new sample, which was only accepted if it was within 15% of the previous “bypass” data recorded. Utilizing only samples within the 15% range ensured that sampling only began once particle concentrations had become stable, and ended before concentration levels began to drop off when the generation devices needed refilling. Alternating samples of the bypass and microtrap lines continued with the goal to collect a sufficient number of samples to reduce the size distribution uncertainty at the highest size bin to a level of uncertainty under 10% based on Poisson statistics. The average of the two “bypass” measurements was used for the calculation of the collection efficiency values:
For inertial impactors like the microtrap, collection efficiency may be expressed as a function of Stokes number to remove an explicit dependence on flow rates (Hinds Citation1999). The dimensionless Stokes number (Stk) was calculated over the selected particle size range for experiments 1 and 2 ().
The definition of the Stokes number can be used to derive the dependence of pressure drop for a multijet impactor on the number of jets N of a stage. The jet velocity (V) = 4Q/NπDj 2, with Q/N equal to the flow rate per jet. For a fixed cut-point dp and Stokes number, ∼dp 2 V/Dj , the jet diameter, Dj , can be eliminated from an expression for the pressure drop Δp ∼ V 2 ∼ (Q/Dj 2N)2 ∼ 1/N 2/3. This relation demonstrates as to how the pressure drop can be reduced by increasing the number of jets (and commensurately reducing the diameter by the factor N 1/3). The microtrap impactor uses this approach to reduce the operating pressure drop.
Effect of Flow Rate on Collection Efficiency
The collection efficiency of the microtrap impactor was evaluated at various flow rates. For Experiment 1 (), ACTD was used and the impactor was operated at flow rates of 10, 8.9, and 7.7 L/min. These flow rates correspond to pressure drops of 0.125 kPa (0.5 inches H2O), 0.100 kPa (0.4 inches H2O), and 0.075 kPa (0.3 inches H2O), respectively. Three sets of 20 one-minute samples were measured by the APS at each flow rate, for a total of 60 samples per flow rate.
Effect of Particle Type on Collection Efficiency
The collection efficiency of the impactor was evaluated for various particle types (Experiment 2, ). For this experiment, measurements were recorded at a flow rate of 10 L/min, with a corresponding pressure drop of 0.125 kPa, using ACTD, KCl, and OA particles. For each particle type, 60 one-minute samples were generated.
Effect of Loading on Collection Efficiency
To characterize the performance of the microtrap impactor over time (Experiment 3, ), the microtrap impactor was operated at 10 L/min with KCl particles for a 10 h time period at total mass concentration levels of 30 mg/m3. Due to the high concentration level used, the 10 h sampling period was representative of a 24 h period at even highly polluted ambient conditions. Microtrap impactor efficiencies were calculated using EquationEquation (1) and the method described above for three selected particle sizes (0.58, 1.2, and 4.1 μm).
Effect of Plate Coatings on Collection Efficiency
The effect of oleic acid antibounce coatings was investigated in Experiment 4 (). The oleic acid coating was applied by aerosolizing the solution, as described above, and running the particles through the microtrap impactor at 10 L/min to directly coat the microtrap wells. Several coating times were tested and 1 h was found to be optimum. Once the plate was coated, collection efficiency was tested using KCl particles.
Two-Stage Impactor Experiments
An independent set of experiments using the same instrumentation and experimental setup, as described above, was conducted in Berkeley (ADI entries in ) to confirm the initial results and investigate a dual-stage configuration. For confirmation of impactor cut-points, tests with nebulized saturated KCl solutions and pure oleic acid were employed to generate larger size distributions relative to those generated by more dilute solutions. These experiments explored a simple modification of the microtrap geometry to achieve larger cut-points while maintaining the same total target flow of 10 L/min. Additionally, this configuration was tested at a larger range of flow rates than the single stage alone (Experiment 1, ) due to the expectation of nonideal impactor behavior from the modified configuration. One jet plate was hand drilled to enlarge the jet diameters from 0.53 mm to 0.79 mm to achieve a 3.3 μm cut-point to capture particles in the 4–10 μm “tracheobronchial” aerosol fraction. No modification was made in the trap plate. By stacking this modified impactor stage on top of the primary one, the combined two-stage configuration allows the collection of particles in the 1–10 μm “thoracic” fraction (Lippmann Citation2001). The collection efficiencies of each of the two stages were tested independently using ACTD generated by fluidized bed aerosol generator for an extended period of sampling. The generator was filled with dust and allowed to run continuously over a period of approximately 24 h while acquiring 60-s upstream samples periodically to estimate the change in aerosol concentration and size distribution during the course of the sampling. The two-stage impactor collection efficiency was evaluated from an initial dust loading of ∼1 μg up to a final loading of 2 mg. The size distribution varied somewhat over the course of the exposure with the mass mean diameter ranging from 2 to 3.5 μm and the geometric standard deviation ranging from 1.3 to 1.6.
With increased proportions of large particles, a shorter sampling protocol could be used in these experiments. Either eight or sixteen 30-s samples were acquired, alternating upstream (“Bypass”) or downstream (“Microtrap”). To avoid sampling bias, two samples were taken consecutively except for the first and last bypass sample so that an equal number of the two streams were used. Collection efficiency was taken to be:
RESULTS AND DISCUSSION
Primary Single-Stage Impactor
Results of tests on the effect of flow rate on the collection efficiency of the microtrap impactor are shown in , where collection efficiencies are plotted as a function of particle size at three different flow rates. At a flow rate of 10 L/min, the pressure drop across the device was 0.12 kPa, and the 50% cut point (D50) was 1.20 μm. At a flow rate of 8.9 L/min, the pressure drop decreased to 0.10 kPa and the D50 shifted to 1.25 μm. A flow rate of 7.7 L/min resulted in a pressure drop across the device of 0.075 kPa, and a D50 of 1.35 μm. All three curves had an index of sharpness of 1.2 defined as the ratio of diameters corresponding to 75% and 25% collection efficiency (i.e., dp (E = 0.75)/dp (E = 0.25). The error bars shown in represent the standard deviation of the collection efficiency over the 60 samples recorded at each flow rate. Minor negative collection efficiencies were seen for solid particles less than 1 μm. This can be attributed in part to high particle concentrations generated and to coarse particles shattering upon impact with the collection substrate, producing multiple smaller particles. Additional experiments using oleic acid showed no such artifacts.
FIG. 3 Collection efficiency variation with particle diameter for ACTD particles on an uncoated impaction surface at various flow rates.
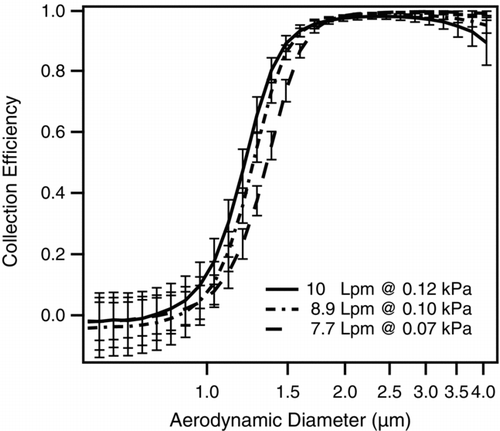
shows the results of this evaluation by plotting collection efficiency as a function of the Stokes number. This figure shows that the microtrap impactor behaves as an ideal inertial impactor, since its collection efficiency is only a function of Stokes number, and is not affected by varying flow rates. The Stokes number for 50% collection efficiency (Stk50) was found to be approximately 0.24 for the ACTD particles, with the square root of the Stokes number (√Stk50) of 0.49. This value matches the experimental √Stk50 value of 0.49 found for ideal inertial impactors (Hinds Citation1999; Kim et al. 2006). The collection efficiency curve for this microtrap impactor was found to be sharper than the efficiency curve of an impactor with an equivalent cut size (1.2 μm) designed and characterized by Sioutas et al. Citation1999.
shows the impactor's collection efficiency as a function of the Stokes number for ACTD, KCl, and OA aerosols at a flow rate of 10 L/min. The Stk50 for all three particle types was found to be 0.24 with a √Stk50 of 0.49. These √Stk50 values match the experimentally determined √Stk50 of 0.49 for circular jet inertial impactors (Hinds Citation1999; Kim et al. 2006).
FIG. 4 Collection efficiency variation with Stokes number for ACTD particles on an uncoated impaction surface at various flow rates.
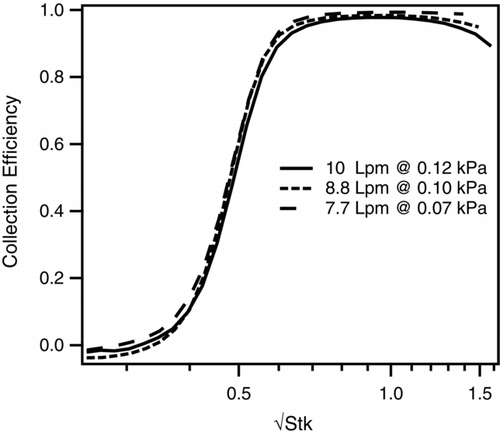
FIG. 5 Collection efficiency as a function of Stokes number for various particle types on an uncoated impaction surface at a 10 L/min flow rate through the microtrap impactor.
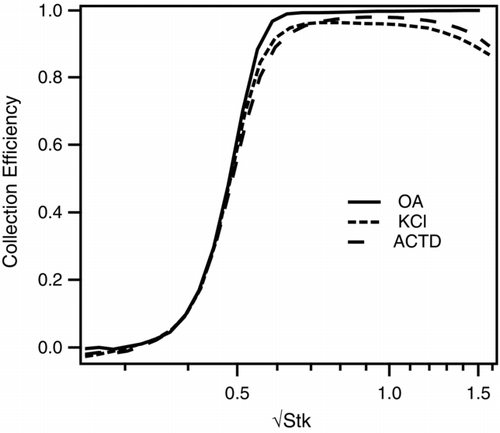
The effect of loading time on collection efficiency was investigated using KCl aerosols at a flow rate of 10 L/min over a 10-h exposure time. Results of these experiments are shown in , where collection efficiencies are plotted as a function of time for three selected particle sizes. For particles less than 1 μm (0.6 μm), collection efficiency remained around zero throughout the experiment, regardless of exposure time. Collection efficiency of 1.2 μm particles initially increased over time as the well plate became loaded with particles, causing the surface area for collection to increase. As loading continued, the collection efficiencies decreased and stabilized at around 50% for 1.2 μm particles. Collection efficiency for 3 μm particles remained approximately 96% through the 10-h exposure period. Similar to the results found by Biswas and Flagan (Citation1988) for a particle trap impactor, collection efficiencies did not decrease with loading as long as the microtrap cavities remained unfilled.
FIG. 6 Collection efficiency as a function of time for KCl particles on an uncoated impaction surface at a 10 L/min flow rate.
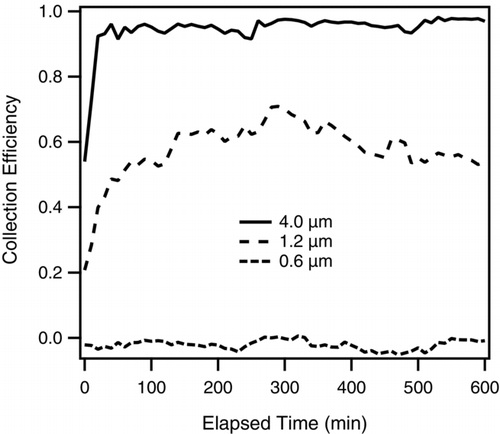
FIG. 7 Collection efficiency dependence of microtrap impactor on jet geometry and total flow rate using polydispersed oleic acid droplets.
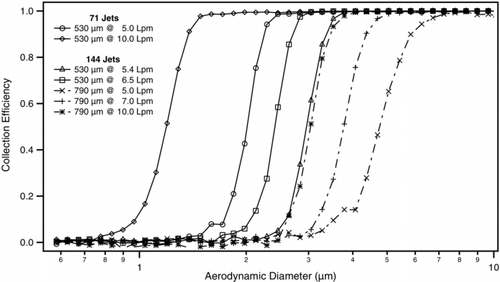
Results from experiments on the effectiveness of oleic acid as an antibounce coating showed that the coating provided small improvements in collection efficiency for solid particles greater than 1.2 μm, and did not affect the impactor cut size. The addition of the oleic acid coating improved collection efficiency of solid KCl particles of 5 μm diameter from around 80% to 88%. Over time, however, an impactor with an uncoated well plate reached higher collection efficiencies (about 97% for 5 μm particles) than a plate coated with oleic acid. These results correspond with previous findings showing that over time and with heavy particle loading an uncoated cavity-type surface will reach the same level of collection efficiency as an oil-coated cavity surface (Tsai and Cheng Citation1995). As particle loading on an uncoated plate increased over a 5-h test period, particle bounce off decreased. It is likely that the collected particles initially functioned as an antibounce coating; however, this effect is expected to disappear as the loading increases and reaches a critical point. Based on the results shown in , this effect is expected to occur beyond a 10-h sampling period under similar conditions.
Dual Stage Impactor
Results of the series of measurements exploring the collection efficiency relative to number of jets, jet diameter, and flow rate using polydispersed OA are shown in . Sigmoidal curve fits to these efficiency curves plus four other curves for KCl (not shown) resulted in a range of √(Stk50) of 0.42–0.52 with an average of 0.46 ± 0.04, which demonstrates the close adherence of the microtrap impactor to ideal impactor performance. With the exception of the 144 × 790 μm jet plate at 5 L/min, all collection efficiency curves are equally sharp with sharpness factor = 1.16 ± 0.003. The excepted curve has a steepness factor of 1.24 owing to the smaller jet Reynolds number of that configuration. As observed with the original single-stage tests, the collection of solid particles occurs efficiently over roughly a factor of two in √(Stk). By combining two impactor stages, bounce-free solid particle collection is expected in the 1–10 μm range.
Results of testing such a dual-stage collector are shown in where the dependence of collection efficiency with dust particle loading is given. After the first ∼1 μg of loading, particle bounce is virtually eliminated and bounce-free collection is maintained thereafter up to a level of approximately 2 mg. At this point, a small amount (∼15%) of large particle bounce is evident above ∼6 um. At 10 L/min, a coarse particle sampler based on this dual stage configuration would have to sample the highly polluted particle concentration of 140 μg /m3 for 24 h before reaching this level of particle loading.
CONCLUSIONS
Results of this characterization showed the microtrap impactor size-cut at 1.2 μm for solid and liquid particles has a collection efficiency above 97% for particles greater than 2 μm in diameter at a 10 L/min flow rate and a pressure drop of 0.12 kPa. The microtrap impactor was shown to behave as an ideal inertial impactor for liquid and solid particles with a √Stk50 of 0.49 for the 1.2 size-cut. Efficiencies were shown to be consistent for lengths of time up to 10 h with an uncoated impaction surface, allowing for the extraction and analysis of biological samples. A dual-stage version was tested to demonstrate the feasibility of a coarse particle collector targeting the respirable (1–4) and tracheobronchial (4–10) aerosol fractions. Loading tests of this configuration indicated that very large loadings (>1 mg) are possible with an uncoated surface and only minimal initial bounce (first 1 μg).
The microtrap impactor's jet and well-plate configuration is designed for operation at a low-pressure drop, enabling low power consumption and quiet operation. Due to the device's small size and pressure drop, there is potential for the microtrap impactor to be adapted as a personal sampler. Future plans for the microtrap impactor include testing the device as personal sampler by incorporating a fan as an air mover instead of the normal personal sampling pump that often weighs as much or more than the sampler itself. Operating at 10 L/min flow rate with only a 0.12 kPa pressure drop, the microtrap impactor could, in theory, be supplied by an integrated miniature DC blower powered by a small battery, thus avoiding the need for a separate air pump. Additionally, the impactor's housing could easily be made compact and lightweight to allow for greater portability as a remote area sampler and potentially as a personal impactor. Further, being 6-mm thick and 52 mm in diameter, the two-stage impactor assembly tested here naturally matches the dimensions of a standard 47 mm filter such that it could be used as both a coarse particle collector and a pre-cut to a fine aerosol backing filter. Adding stages would also be possible. Future plans for the microtrap impactor also include characterizing the recovery of biological aerosols as respirable and tracheobronchial fractions using the dual-stage collector and examining its use as an oil-less pre-cut for 47 mm filter collection of organic aerosols for speciated personal exposure.
Acknowledgments
The authors thank R. Rosario and T. Tablante for their assistance with the CAD drawings of the microtrap impactor.
REFERENCES
- Andersen Instruments . 1999 . Marple Personal Cascade Impactors, 290 Series Operators Manual .
- Biswas , P. and Flagan , R. C. 1988 . A Particle Trap Impactor . J. Aerosol Sci. , 19 : 113 – 121 .
- Chang , M. , Kim , S. and Sioutas , C. 1999 . Experimental Studies on Particle Impaction and Bounce: Effects of Substrate Design and Material . Atmos. Environ. , 33 : 2313 – 2322 .
- Cherrie , J. W. and Aitken , R. J. 1999 . Measurement of Human Exposure to Biologically Relevant Fractions of Inhaled Aerosols . Occup. Environ. Med. , 56 : 747 – 752 .
- Custovic , A. , Green , R. , Fletcher , A. , Smith , A. , Pickering , C. A. , Chapman , M. D. and Woodcock , A. 1997 . Aerodynamic Properties of the Major Dog Allergen, Can f 1: Distribution in Homes, Concentration and Particle Size of Allergen in the Air . Am. J. Respir. Crit. Care Med. , 26 : 1246 – 1252 .
- D’Amato , G. 2002 . Environmental Urban Factors (Air Pollution and Allergens) and the Rising Trends in Allergic Respiratory Diseases . Allergy , 57 ( 72 ) : 30 – 33 .
- Devereux , G. 2003 . The Increase in Allergic Disease: Environment and Susceptibility. . Clin. Exp. Allergy , 33 : 394 – 406 .
- Dillon , H. K. , Miller , J. D. , Sorenson , W. G. , Douwes , J. and Jacobs , R. R. 1999 . Review of Methods Applicable to the Assessment of Mold Exposure to Children . Environ. Health Persp. , 107 : 473 – 480 .
- Dunbar , C. , Kataya , A. and Tiangbe , T. 2005 . Reducing Bounce Effects in the Anderson Cascade Impactor . Int. J. Pharm. , 301 : 25 – 32 .
- Dzubay , T. H. , Hines , L. E. and Stevens , R. K. 1976 . Particle Bounce Errors in Cascade Impactors . Atmos. Environ. , 10 : 229 – 234 .
- Fang , C. P. , Marple , V. A. and Rubow , K. L. 1991 . Influence of Cross-Flow on Particle Collection Characteristics of Multi-Nozzle Impactors . J. Aerosol Sci. , 22 : 403 – 415 .
- Hinds , W. C. 1999 . Aerosol Technology: Properties, Behavior and Measurement of Airborne Particles , New York : John Wiley & Sons, Inc .
- Hinds , W. C. , Liu , W. C. V. and Froines , J. R. 1985 . Particle Bounce in a Personal Cascade Impactor: A Field Evaluation . Am. Ind. Hyg. Assoc. J. , 46 : 517 – 523 .
- Horner , W. E. , Kailas , J. , Stankus , R. P. and Lehrer , S. B. 1991 . Common German Cockroach Whole Body and Fecal Allergens: Immunoprint Inhibition Studies . Int. Arch. Allergy Appl. Immunol. , 78 : 112 – 114 .
- John , W. and Kreisberg , N. 1999 . Calibration and Testing of Samplers with Dry, Polydisperse Latex . Aerosol Sci. Technol. , 31 : 221 – 225 .
- Karlsson , A. S. , Renstrom , A. , Hedren , M. and Larsson , K. 2002 . Comparison of Four Allergen-Sampling Methods in Conventional and Allergy Prevention Classrooms . Clin. Exp. Allergy , 32 : 1776 – 1781 .
- Kim , D. S. , Lee , K. W. and Kim , Y. J. 2006 . Characterization of a Particle Trap Impactor . J. Aerosol Sci. , 37 : 1016 – 1023 .
- Kreisberg , N. and Hering , S. V. Aerosol Dynamics Inc., Assignee, Particle Microtrap Screen, U.S. Patent Number 6,284,025 B1. Sep. 4 . 2001 .
- Lippmann , M. 2001 . “ Size Selective Health Hazard Sampling ” . In Air Sampling Instruments , Edited by: Cohen , B. S and McCammon , C. S. Cincinnati, OH : ACGIH .
- Markowski , G. R. 1984 . Reducing Blowoff in Cascade Impactor Measurements . Aerosol Sci. Technol. , 3 ( 4) : 431 – 439 .
- Montoya , L. D. and Hildemann , L. M. 1997 . Size-Resolved Quantification of Non-Viable Indoor Aeroallergens: A Review . J. Environ. Eng. , 123 : 965 – 973 .
- Montoya , L. D. and Hildemann , L. M. 2001 . Evolution of the Mass Distributions of Resuspended Cat Allergens (Fel d 1) Indoors Following a Disturbance . Atmos. Environ. , 35 : 859 – 866 .
- MSP Corporation . 2009 . Model 200—Personal Environmental Monitor™ (PEM™) Product Information Brochure .
- NAEPP/NHLBI . 1997 . Expert Panel Report II: Guidelines for the Diagnosis and Management of Asthma , Bethesda, MD : National Institute of Health, No 97–4051 .
- Platts Mills , T. A. E. , Vervloet , D. , Thomas , W. R. , Aalberse , R. C. and Chapman , M. D. 1997 . Indoor Allergens and Asthma. Report of the Third International Workshop . Allergy Clin. Immunol. , 100 : S1 – S24 .
- Rao , A. K. and Whitby , K. T. 1978 . Non-ideal Collection Characteristics of Inertial Impactors –i. Single-Stage Impactors and Solid Particles . J. Aerosol Sci. , 9 : 77 – 86 .
- Reischl , G. P. and John , W. 1978 . The Collection Efficiency of Impaction Surfaces . Staub. Reinhalt. Luft. , 38 : 55
- Sioutas , C. , Chang , M. C. , Kim , S. , Koutrakis , P. and Ferguson , S. 1999 . Design and Experimental Characterization of a PM1 and PM2.5 Personal Sampler . J. Aerosol Sci. , 30 : 693 – 707 .
- Tsai , C. J. and Cheng , Y. H. 1995 . Solid Particle Collection Characteristics on Impaction Surfaces of Different Designs . Aerosol Sci. Technol. , 23 : 96 – 106 .
- Turner , J. R. and Hering , S. V. 1987 . Greased and Oiled Substrates as Bounce-Free Impaction Surfaces . J. Aerosol Sci., , 18 : 215 – 244 .
- Umetsu , D. T. , McIntire , J. J. , Akbari , O. , Macaubas , C. and DeKruyff , R. H. 2002 . Asthma: An Epidemic of Dysregulated Immunity . Nature Immunol. , 3 ( 8 ) : 715 – 720 .