Abstract
Cavity ring-down spectroscopy (CRDS) is a technique that is commonly used to measure the extinction of light by aerosol particles in situ. This extinction, when normalized to particle concentration, yields the extinction cross section, a measure of a single particle's ability to scatter and absorb light. The complex index of refraction can then be retrieved by comparison of the extinction cross sections at several particle diameters with those predicted by Mie theory. This approach requires accurate determination of particle diameter and concentration as well as the length of the extinction region in the cavity, but it is often difficult to quantify the systematic errors in the measurements of these quantities. Here, we introduce a calibration technique using particles of a reference compound to account for these systematic errors. The two calibration parameters are: Cf
, which scales the measured extinction cross sections, and Δd, which shifts the particle diameters. It is found that Cf
correlates strongly with the condensation particle counter (CPC) used to measure particle concentration and that Δd is associated with the differential mobility analyzer (DMA) used to select particle diameters. Calibration is shown to reduce errors of subsequently-measured extinction cross sections of a test aerosol from 11% to with a concomitant improvement in the accuracy of the retrieved complex index of refraction and corresponding atmospheric radiative forcing estimates.
Copyright 2013 American Association for Aerosol Research
1. INTRODUCTION
The development of the cavity ring-down spectroscopy (CRDS) technique by O’Keefe and Deacon (Citation1988) precipitated rapid progress in atomic and molecular absorption measurements. CRDS has been widely reviewed (Zalicki and Zare, Citation1995; Scherer et al. Citation1997; Wheeler et al. Citation1998; Busch and Busch, Citation1999), and the key advantages of this direct absorbance technique originate from the long effective pathlengths (>1000 m) and insensitivity to shot-to-shot intensity fluctuations. CRDS has received considerable attention in several fields for its ability to achieve absorption sensitivity better than 10−8 cm−1 with a relatively simple optical setup (Busch and Busch, Citation1999). It has been used to measure scattering and absorption by aerosol particles (Sappey et al. Citation1998; Vander Wal and Ticich, Citation1999; Bulatov et al. Citation2002; Thompson et al. Citation2002; Pettersson et al. Citation2004), as well, and is unique in atmospheric studies because of its ability to measure real-time, in situ optical extinction.
Recently, several groups have used Aerosol CRDS (A-CRDS) to probe internal and external particle mixing (Riziq et al. Citation2007; Freedman et al. Citation2009; Khalizov et al. Citation2009; Lack et al. Citation2009; Radney et al. Citation2009; Attwood and Greenslade, Citation2012), effects of relative humidity (Beaver et al. Citation2008; Attwood and Greenslade, Citation2012; Brem et al. Citation2012; Trainic et al. Citation2012) and particle reactivity (Lu et al. Citation2011; Zarzana et al. Citation2012). Agreement between extinction cross section measurements and those calculated with Mie theory is often promising, typically on the order of 2–12 % (Pettersson et al. Citation2004; Baynard et al. Citation2007; Riziq et al. Citation2007; Freedman et al. Citation2009; Lang-Yona et al. Citation2009; Radney et al. Citation2009; Miles et al. Citation2010b; Li et al. Citation2011; Mellon et al. Citation2011). It is even possible to determine the complex index of refraction of an aerosol by comparing measurements to Mie theory predictions, though Miles et al. (Citation2010a) have pointed out that accuracy can be limited by uncertainties in the particle-light interaction length, the particle counting efficiency and particle diameter. In fact, several studies have demonstrated that it can be difficult to retrieve accurate indices of refraction even of aerosols containing only a single species. For example, A-CRDS experiments with purely scattering ammonium sulfate particles (Lang-Yona et al. Citation2009; Bluvshtein et al. Citation2012) and polystyrene latex spheres (PSL's) (Lang-Yona et al. Citation2009) have yielded measured indices of refraction erroneously containing significant absorbing components, (e.g., m=1.517+0.018i for ammonium sulfate and m=1.60+0.01i for PSL's). Incorrectly determined indices of refraction could lead to drastic mis-estimation of aerosol radiative forcing in the troposphere, for example, indicating much less of a cooling effect than there really is. Clearly, more accurate A-CRDS measurements are needed if the retrieved indices of refraction are to be useful in estimating radiative properties of ambient aerosols.
The general approach to retrieving an index of refraction is to first measure the extinction of light by particles (in units of cm−1, for example), and while this can be measured absolutely using A-CRDS, the length of interaction between the light and the particles in the cavity must be determined separately. This length is generally shorter than the length between the mirrors, and uncertainty in the ratio of these two lengths can propagate to uncertainty in the measured extinction. Next, extinction per particle, the extinction cross section (in units of cm2/particle, for example), is calculated by dividing the measured extinction by the particle concentration (in units of particles/cm3, for example). Of course, uncertainty in the concentration measurement contributes an additional source of uncertainty to the cross section measurement. Extinction cross sections measured for aerosols of several different diameters can then be compared to those predicted by Mie theory for spherical, homogeneous particles with a specific complex index of refraction, m=n+ik, where n is the real, nonabsorbing component and k is the imaginary, absorbing component. Fitting routines can then be used to retrieve the best-fit value of the index of refraction of the particles by comparing experiment to theory, but uncertainties in the selected particle diameters introduce additional uncertainty in the measured index of refraction.
Here, we describe a calibration technique that reduces the uncertainty on the index of refraction retrieved from A-CRDS measurements. Extinction cross sections of a calibration standard with a known index of refraction, squalane, are measured for several diameters and compared to cross sections predicted by Mie theory. The measured cross sections are scaled by an adjustable calibration factor, Cf , and the selected diameters are shifted by an adjustable offset, Δd, to minimize the differences between the measured and calculated cross sections. The Cf term accounts for systematic errors in both the particle counting associated with a condensation particle counter (CPC) and estimation of the length of interaction between the particles and the light, whereas the Δd term accounts for the systematic error in the particle diameters selected with a differential mobility analyzer (DMA). As a test of the calibration, these parameters are applied to subsequent extinction cross section measurements of an aerosol generated from a different liquid with a known bulk refractive index (Cargille immersion liquid, IL 1160), and it is found that the calibration leads to improved agreement with Mie theory predictions and accuracy in the retrieved index of refraction. Finally, the impact that more accurate measurement of aerosol optical properties has on estimated direct radiative forcing is discussed.
2. MATERIALS AND METHODS
2.1. Chemicals and Gases
Squalane (CAS #111-01-3) was purchased from Sigma-Aldrich and used without further purification. It was chosen as the calibration standard because it is a low-volatility liquid (vapor pressure ∼10−7 Torr at room temperature [Köhler et al. Citation2005]) that is stable at room temperature when exposed to air. Additionally, its index of refraction has been measured throughout the UV and visible regions of the spectrum: Painter et al. (Citation1984) ( in that paper) measured a value of n=1.472 at 355 nm, while our fit of the Cauchy dispersion equation to the data of Sörensen et al. (Citation1951) at visible wavelengths yields an extrapolated value of n=1.470 at 355 nm. In the present work, the value measured by Painter et al. is used. The immersion liquid standard, Cargille IL 1160, is a mixture of phthalate esters purchased from Cargille Laboratories; its index of refraction at 355 nm is calculated to be n=1.515 using the UV-visible Cauchy equation coefficients supplied by the manufacturer. Nitrogen gas was taken from a liquid nitrogen dewar purchased from Airgas.
FIG. 1 Schematic diagram of the pulsed, 355 nm A-CRDS setup for measuring extinction cross sections of aerosol particles. WW: Wedged window, L1: lens with focal length f=25 mm, A1: 100 μm aperture, L2: lens with focal length f=50 mm, A2: 1 mm aperture, CPC: condensation particle counter, DMA: differential mobility analyzer, PMT: photomultiplier tube, L: length between mirrors, and ℓ: distance occupied by aerosol particles. Particles are mixed and diluted in two different configurations indicated by the labels A and B. (Color figure available online.)
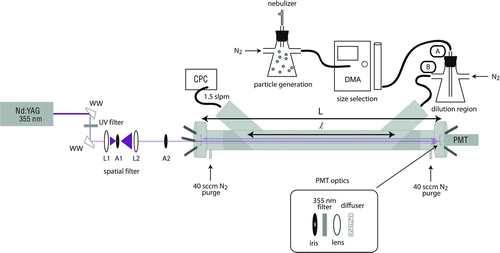
2.2. Aerosol Generation, Size Selection, and Counting
A schematic diagram of the experimental setup is shown in . Pure liquid samples were aerosolized by a concentric nebulizer (Type A, Environmental Express) with 0.30–0.35 SLPM (standard liters per minute) of nitrogen flow controlled by a mass flow controller. This method of generating aerosols has a few advantages over the more-commonly used atomizer and dryer method, including that a wider range of species can be used since solubility in a solvent is not necessary and that potential artifacts associated with solvent remaining on the particles are eliminated. The nebulizer is also simpler and less expensive to set up and use than an atomizer/dryer system. Of course, the nebulizer cannot aerosolize solid species unless they are dissolved in a solvent. The nebulizer produced a broad distribution of particle diameters between 50 and 1000 nm that peaked at approximately 300 nm. This polydisperse aerosol was mixed with a 0.1–0.6 SLPM nitrogen flow in a 1 L flask and then drawn through an inertial impactor (0.0508 cm orifice) at the entrance of the differential mobility analyzer (DMA; TSI 3080L Electrostatic Classifier) at a flow rate of 0.270 SLPM. The dilution made it possible to vary the particle concentration and reduce clogging of the impactor orifice. The excess flow was exhausted from the mixing flask through a filtered vent at atmospheric pressure. The calculated d 50 cut-point (diameter at which only 50% of the particles penetrate the impactor) for these conditions is 964 nm (Hinds Citation1999). The orifice was cleaned with methanol daily, and the impaction plate was wiped clean between each measurement.
Inside the DMA, the aerosol passed through a bipolar charger containing a 10 mCi 85Kr radioactive source (TSI 3077A) that established an equilibrium charge distribution on the particles (Wiedensohler Citation1988; Kim et al. Citation2005). The DMA then selected particles of a specified electrical mobility with a constant sheath flow of 2.7 SLPM resulting in the transmission of a narrow range of mobility diameters, dm
. Since the particles are liquid and presumed to be spherical, the physical diameter, d, and the mobility diameter, dm
, are equivalent. For the remainder of this work, the term “diameter” and the symbol d refer to the physical diameter, but it is understood that it is equivalent to the mobility diameter. The distribution of diameters selected by the DMA is assumed to be log-normal described by a geometric mean diameter, , (which is also the median diameter) and a geometric standard deviation, σ
g
. The geometric standard deviation was taken to be σ
g
=1.05, which is similar to values reported previously for this type of DMA (Hering and McMurry Citation1991; Spindler et al. Citation2007; Anttila et al. Citation2009; Harmon et al. Citation2010). Particles with d> 600 nm were selected such that contributions by doubly-charged particles transmitted by the DMA accounted for less than 1% of the measured extinction as estimated from the distribution of diameters produced by the nebulizer, the charging efficiency of the 85Kr source and DMA transfer function.
The selected aerosol was mixed in a 250 mL flask and further diluted with 1.10 SLPM of nitrogen before flowing through the CRD cell. The sample flow rate into the DMA was measured with a flow meter (TSI 4140) between each measurement, and the final dilution was adjusted accordingly to achieve a consistent 0.270 SLPM aerosol sample flow into the DMA. Particle losses inside the CRD cell due to gravitational settling, inertial deposition, and diffusion are estimated as <1% for 600–900 nm particles (Baron and Willeke Citation2001), and this was confirmed by measuring average particle concentrations at the entrance and exit of the cell. A butanol condensation particle counter (CPC; Model 3775, TSI) was used to measure the particle concentration exiting the cavity, typically 300–800 cm−3. The flow pulled by the CPC is nominally 1.50 SLPM, though when attached to the A-CRDS system with the DMA and flow-limiting impactor this flow was measured to be only 1.45 SLPM. Consequently, the concentration reported by the CPC is systematically low by approximately 3%, but this error is corrected by use of the calibration parameter Cf described in this work. The manufacturer's specifications quote ±10% accuracy for the measured particle concentration (TSI Citation2010).
2.3. CRD Apparatus
The CRD sampling cell was constructed of a 1 m long, 3/4'' o.d. stainless steel tube with inlet and outlet ports at a 45° angle to reduce turbulence in the cell. Teflon plugs with a 1/4'' diameter hole drilled through them and angled at 45° were inserted into the cell near the inlet and outlet ports. An optical cavity was formed from two highly-reflective concave mirrors (Los Gatos Research, Inc., reflectivity = 99.97% at 365 nm, 6 m radius of curvature) held in mirror mounts fitted with micrometer adjustment screws. A typical ring-down time of the cavity filled with nitrogen was τ0=2900 ns corresponding to a mirror reflectivity of 99.88% at 355 nm ignoring the minor extinction due to Rayleigh scattering by N2 (0.007% per pass). A fast fourier transform (FFT) of the ring-down decay trace revealed a resonating longitudinal mode and a free spectral range of Δν=145.37 MHz corresponding to a distance between the mirrors of L= 103.1 cm (Δν=c/2L; c= speed of light) (Berden and Engeln Citation2009). The length in the cell occupied by the aerosols was assumed to be the distance between the Teflon plugs at the inlet and exit ports, which was measured to be ℓ= 88.2 cm. The corresponding ratio of lengths was RL =L/ℓ=1.17. A 40 sccm (standard cubic centimeters per minute) purge flow of nitrogen was introduced at the face of each mirror to limit degradation of the mirror reflectivity from aerosol deposition, and calculated particle concentrations in the cell accounted for dilution by this flow.
A Nd:YAG laser (Spectra-Physics, PRO-250) was used to generate 355 nm (third harmonic) pulsed (8 ns) light at a 10 Hz repetition rate. The laser pulses passed through a spatial filter consisting of a focusing lens (focal length = f = 25 mm), a 100 μm pinhole aperture, and a collimating lens (f = 50 mm), and then through a 1 mm aperture before entering the CRD cell. Typically, the pulse energy entering the cell was 15 μJ. The light exiting the cell passed through an adjustable iris, a 355 nm optical bandpass filter, a focusing lens and a diffuser before being detected by the photomultiplier tube (PMT; Hamamatsu 6533). Ten decay transients were sampled at 200 MSa/s (megasamples per second) and averaged on the oscilloscope (HP 5483, 600 MHz, 50 Ω termination, 8 bit ADC). The digitized signal was transferred to a personal computer for exponential fitting with the Linear Regression of the Sum (LRS) algorithm (Halmer et al. Citation2004) in a custom LabVIEW program. The LRS algorithm was selected because of its computational efficiency and reported noise immunity (Everest and Atkinson Citation2008). Multi-exponential decay originating from off-axis alignment was evident in the FFT of the decay transient (Lee et al. Citation2002) and was minimized during cavity alignment. Additionally, the mono-exponential nature of the decay was monitored in real time by comparing decay times measured by fitting several subregions of the full decay.
The extinction due to particle scattering and absorption losses, αext, was determined by comparing the particle-free cavity decay time, τ0, to the decay time with the aerosol sample, τsamp:


3. RESULTS AND DISCUSSION
3.1. Calibration by Comparing to Mie Theory
The A-CRDS instrument was calibrated using particles of squalane, which has a well-characterized index of refraction of m=1.472+0.000i at 355 nm. The measured extinction cross sections (σext) were compared to the effective calculated Mie extinction cross sections () weighted by the log-normal distribution of diameters, n(d), selected by the DMA:
Extinction cross sections were measured for several different geometric mean diameters, , selected by the DMA in the range 600–900 nm. Two calibration parameters, Cf
and Δd, were adjusted to achieve the best fit between the measured and effective Mie cross sections according to a chi-squared, χ2, method:

An example of a calibration using squalane is shown in . The measured σext data are shown by the open circles, and the adjusted data scaled and shifted by the best-fit values of Cf and Δd, respectively, are shown by the solid squares. The best-fit values of Cf and Δd are those that minimize the value of χ2 indicating the best agreement between the adjusted cross-section data and the effective Mie cross-section curve (solid line) calculated using n=1.472, the index of refraction of squalane. A compilation of many such calibrations with squalane performed over several months is shown in in which it can be seen that the Cf parameter is generally less than one suggesting that the measured particle extinction cross sections are systematically too large. Likewise, the Δd parameter is always greater than 0 nm indicating that the diameters selected by the DMA are systematically smaller than the actual diameters.
FIG. 2 Example of calibration using squalane particles. A χ2 minimization was performed in which the σext (open circles) values were scaled by Cf
(=0.935) and the diameters were shifted by Δd (=17 nm) (solid squares) to obtain the best fit between the measured cross sections and the calculated effective Mie cross sections,
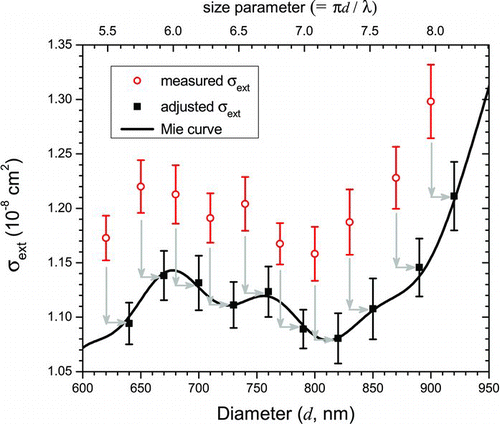
FIG. 3 Best-fit parameters Cf and Δd obtained from squalane calibrations over the course of several months. Dashed lines indicate values if no corrections were necessary. Little correlation between the parameters Cf and Δd suggests that they represent independent errors associated with the CPC for particle concentration measurements and the DMA for particle size selection. (Color figure available online.)
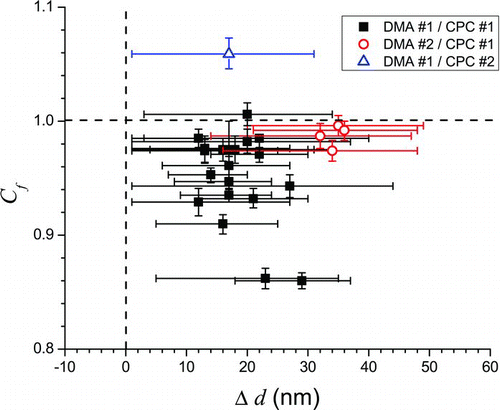
In general, the calibration is more sensitive to the Cf parameter than it is to the Δd parameter, as highlighted by the comparatively smaller error bars on the Cf values in . The Cf parameter accounts for most of the observed day-to-day variation from the calculated Mie extinction curve for squalane particles; simply scaling the cross sections by the Cf factor for each trial in reduces the absolute error in σext from 5.4 to 1.4%. Further refinement of the fits using the best-fit Δd value for each trial, as well, results in additional improvement in the absolute error from 1.4 to 0.5%. Consequently, it would be practical to reduce the time required for daily calibrations by assuming a value for Δd based on previous calibrations and fitting only for Cf . In that case, the calibration could be performed with only one selected particle diameter in as little as about 20 min, whereas a more complete calibration using 4–10 selected diameters could take 2–4 h. If such a one-diameter calibration is carried out, though, it would be beneficial to choose a diameter at which the extinction cross section varies minimally with diameter (for example, 730 nm for squalane, ) to minimize any error in the assumed value of Δd. There is a very weak correlation between the Cf and Δd parameters (R 2 = 0.18 for a linear fit) indicating that they likely represent distinct sources of systematic error, the physical bases for which are discussed in the following sections.
3.2. Calibration Factor, Cf
The Cf parameter could represent several sources of systematic error, including over- or under-counting of particle concentration by the CPC, error in the measurement of RL , particle losses inside and after the CRD cell, and inhomogeneity of the aerosol sample within the sampling volume. As it is applied, this calibration factor is independent of particle size and cannot account for any potential size dependence of the errors.
The largest contribution to uncertainty in the A-CRDS measurements of extinction cross section is associated with the particle concentration measurement using the CPC. The manufacturer's stated uncertainty is ±10% which translates directly into an uncertainty on the measured cross section of ±10%. As Miles et al. (Citation2010a) have pointed out, it can be difficult to reduce this uncertainty since the CPC is calibrated using an electrometer, which demonstrates significant errors at concentrations below 1000 particles/cm3, precisely the concentration range typically employed in A-CRDS measurements.
A systematic error in measurement of the particle concentration can also be introduced if the flow into the CPC is not correct. In the present work, this is the case as the concentration reported by the CPC is calculated based on a flow of 1.50 SLPM while the measured flow when connected to the A-CRDS system was only 1.45 SLPM. Consequently, the particle concentration was undercounted by approximately 3% which led to a systematic overestimation of the extinction cross sections and reduction of Cf . To the extent that the flow into the CPC was stable, however, the measured Cf parameter accounts for this error in the concentration measurement.
To test the importance of the CPC particle counting efficiency in determining the value of Cf , two different CPC's (each a TSI Model 3775) were used in measuring squalane extinction cross sections on the same day with the same DMA and all other conditions being the same (). The results of the calibration fits to these trials clearly show a difference (9%) in the values of Cf : 0.974 for CPC #1 and 1.059 for CPC #2. This test provides strong evidence that deviations of Cf from unity are dominated by systematic errors associated with the operation of the CPC. It is noteworthy that in this comparison the values of the best-fit Δd's are similar (+13 nm, or ∼1.7%, for CPC #1 and +17 nm, or ∼2.3%, for CPC #2) demonstrating the independence of the two calibration parameters.
FIG. 4 Squalane extinction cross sections measured on the same day with two different CPC's. The solid line is the calculated Mie curve (m=1.472+0.000i, geometric standard deviation = 1.05), while the dashed lines represent the CPC manufacturer's quoted ±10% uncertainty. The best fit Cf is 0.974 for CPC #1 (circles) and 1.059 for CPC #2 (triangles). (Color figure available online.)
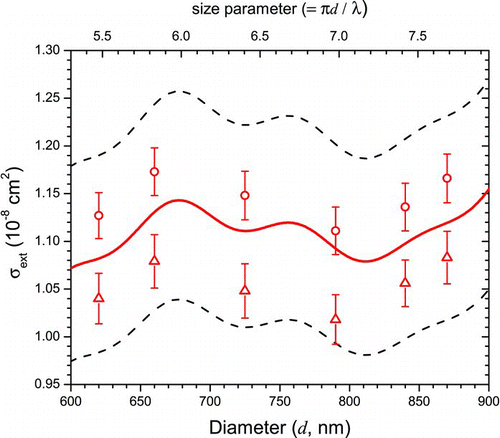
Another potential source of error in calculating the extinction from the measured ring-down decay constants using Equation (1) is the estimation of RL , the ratio of the distance between the mirrors to the distance over which the particles interact with the light. As mentioned previously, the distance between the mirrors can be calculated from the measured free spectral range derived from the FFT of the ring-down decay, and for this system it is L=103.1 cm. The distance over which the particles and light interact is more difficult to measure and may vary depending on aerosol flow conditions and inlet/outlet geometry. Miles et al. (Citation2010a) identify the ratio of these lengths as a major source of uncertainty in retrieving indices of refraction from A-CRDS measurements contributing as much as 2.5% error. Since the Cf calibration parameter scales the measured cross sections, it corrects for any uncertainty on the ratio of these lengths in addition to the uncertainty on the particle concentration.
To investigate how changes in the sample flow could influence Cf
, particles were mixed and diluted in two different ways before they were introduced into the CRD cell (). In configuration A, the particles were fed into the dilution flask through a o.d., 6″ long tube before being carried out near the top of the flask. In configuration B, the flows were reversed such that the particles entered the flask near the top and then were carried out through the tube. The squalane extinction cross sections for several diameters were measured using each configuration with the same DMA and CPC and all other factors remaining unchanged. The resulting best-fit parameters for these trials, both conducted on the same day, reveal the effect that the flow conditions can have on the measurements: Cf
was observed to differ by 4%, the largest same-day variation measured using a single CPC, while Δd was similar for both trials (27 nm ∼3.6% vs. 20 nm ∼2.7%). It should also be noted that changes in the nitrogen purge flows over the mirrors affected the Cf
parameter in a systematic manner so they were maintained at 40 sccm in all experiments.
Clearly, the calibration factor, Cf , is correlated to the aerosol flow conditions, but exactly why is not clear. Perhaps the flow mixing conditions in the flask affect turbulence at the cell inlet thereby influencing the homogeneity of the particle concentration throughout the cell or the length of interaction between the particles and the light. Though the physical reason for the observed dependence on flow dilution and mixing conditions remains unclear, these tests demonstrate that it is necessary to consider and account for them when highly-accurate extinction measurements are to be made with A-CRDS.
3.3. Selected Diameter Correction, Δd
The nominal particle diameter for a σext measurement is the geometric mean diameter, , of the distribution of diameters specified to be selected by the DMA. The fitting parameter, Δd, shifts this distribution of diameters by a constant amount for all measurements accounting for potential systematic errors in the selection of geometric mean diameters. For most trials, the calibration fitting algorithm consistently converged to a best-fit value for Δd of +10 to +30 nm (), which was not correlated with the magnitude of Cf
. Such an error in the distribution of selected diameters, if not corrected, can result in a non-negligible error, which can be either positive or negative, in the calculated effective Mie cross section,
. Consequently, when the index of refraction is retrieved by comparing measured cross sections to those calculated with Mie theory, significant errors can result; for example, Miles et al. (Citation2010a) estimate that errors of only ±(4−7) nm (∼±[1−2]%) in the diameter of PSL's can lead to a 2.9% error in the retrieved index of refraction.
There are many possible sources of the observed offset in diameter. The DMA, itself, may be mis-calibrated because of a mis-calibrated sheath flow. In the present work, however, the sheath flow was measured and calibrated regularly and is not believed to be a significant source of error. Additionally, it has been suggested that the nonuniform field lines at the entrance and exit of the DMA could be responsible for an error in the selected particle diameter (Kinney et al. Citation1991). Finally, it is possible that the DMA does not select the correct diameter when using hydrocarbon liquids, such as are used in the present work, since the DMA software calculates the slip correction factor based on parameters derived from measurements on PSL's.
To confirm the correlation between the Δd parameter and the operation of the DMA, eight trials were conducted with two different DMA's (each a TSI 3080L) using the same flow conditions and the same CPC. The average best-fit value of Δd for DMA #1 was +18 nm (ranging from +12 nm [∼1.6%] to +29 nm [∼3.9%]), while for DMA #2 it was +34 nm (ranging from +32 nm [∼4.3%] to +36 nm [∼4.8%]) (). This difference between the average values of Δd for the two DMA's is statistically significant at the 99% confidence level. On the other hand, the best-fit values of Cf for the two DMA's differed by less than 1% within the same day. Thus, the Δd parameter appears to account for the systematic errors in the operation of the DMA but is independent of the simultaneously-fit Cf parameter.
FIG. 5 (a) Calculated Mie extinction cross-section curves for m=1.472+0.000i with a narrow distribution of sizes typical of PSL's (light [red] curves) and a broader distribution of sizes typical of a DMA (dark [black] curves). The dashed lines represent shifts in diameter (Δd) of ±5 nm. (b) The percent difference in
![FIG. 5 (a) Calculated Mie extinction cross-section curves for m=1.472+0.000i with a narrow distribution of sizes typical of PSL's (light [red] curves) and a broader distribution of sizes typical of a DMA (dark [black] curves). The dashed lines represent shifts in diameter (Δd) of ±5 nm. (b) The percent difference in Display full size for ±5 nm size shifts. Note that the broader distribution reduces the effect of the uncertainty on the diameter. (Color figure available online.)](/cms/asset/e1ae41c1-eecf-4c8d-8520-90b20b541c19/uast_a_805875_o_f0005g.jpg)
3.4. Calibrating with Size-Selected Particles
One of the interesting consequences of calibrating the A-CRDS instrument with particles size-selected by the DMA, as opposed to using PSL's, is that uncertainties in the particle diameter have less of an impact on index of refraction retrieval. Aqueous suspensions of PSL's are often used to validate an A-CRDS system because they have certified tolerances obtained by direct comparison to National Institute of Standards and Technology Standard Reference Materials (Yamada et al. Citation1985). The monodisperse distribution (geometric standard deviation, σ g , ∼1.01) of aerosolized PSL's is attractive as it can be narrower than the output of a DMA. The standard deviations of PSL diameter and size tolerances are typically 1% of the mean diameter for submicron particles limited by uncertainty in the transmission electron microscope measurements and variability of the standard (Duke and Layendecker, Citation2003; Mulholland et al. Citation2006). With such narrow size distributions, it would seem to be straightforward to calibrate an A-CRDS instrument with PSL's, but in fact the narrow size distribution makes the measurements much more sensitive to the uncertainty in the particle diameter. Conversely, the broader distribution of a DMA (geometric standard deviation, σ g , ∼1.05) effectively smooths the rapid variation of the cross section with diameter thereby reducing errors associated with uncertainties on the selected diameter.
This difference can be seen in in which calculated Mie extinction cross sections are shown for particles with m=1.472+0.000i as a function of diameter for two cases: a narrower distribution (light [red] curve, σ
g
= 1.01) representative of PSL's, and a broader distribution (dark [black] curve, σ
g
= 1.05) representative of output from a DMA. Also shown for each case is the extinction cross section curve shifted by ±5 nm (dashed lines) representing an uncertainty on the selected diameter. As seen in , the effect of this uncertainty on can clearly be greater for the narrower distribution; in that case, the calculated
can be in error by as much as 3%, whereas the error is <1% for the broader distribution.
A large error is incurred using the narrower distribution because, at points, the derivative of with respect to d is much greater than for the broader distribution. Such large errors could make the use of PSL's for the precise calibration of an A-CRDS instrument impractical. Additionally, the error in each diameter of PSL sphere used is uncorrelated to the others making it difficult to correct with a single calibration parameter such as Δd. Furthermore, the use of PSL's for calibration is complicated by the facts that: (1) each lot of PSL's could have a different size distribution, (2) separate PSL solutions must be used for each diameter, and (3) solvent or surfactant could remain on the PSL's. The use of a DMA to size-select particles, instead, provides a systematic offset (i.e., Δd) and consistent distribution of diameters (i.e., geometric standard deviation) across the range of sizes as well as a faster and more flexible way to select diameters from a polydisperse source.
3.5. Test of Index of Refraction Retrieval Using Calibration
3.5.1. Measuring the Index of Refraction of a Standard, Cargille IL 1160
The real test of the A-CRDS calibration procedure described in this work is in its ability to improve the accuracy of measurements for aerosols other than the calibration standard, squalane. Toward this end, the complex index of refraction of a commercially-available index-matching standard, Cargille IL 1160, was retrieved from extinction measurements before and after calibration. Cargille IL 1160 was used because it is a liquid at room temperature, has a low volatility, and its bulk index of refraction has been characterized carefully by the manufacturer. The real part of the index of refraction at 355 nm is estimated to be n = 1.515 using the Cauchy equation coefficients supplied by Cargille Industries which were derived from measurements at wavelengths from 308 to 15.5 μm. The imaginary part at 355 nm is interpolated as k=5×10−7 from transmission data provided by the manufacturer at 337 nm and 365 nm.
The complex index of refraction of the Cargille IL 1160 aerosol was retrieved for both the uncalibrated and calibrated cross sections by comparison with Mie theory. The calibration, performed immediately before the measurements, yielded the parameters Cf =0.910 and Δd=16 nm in this example. The results are shown in in which the open (red) circles are the uncalibrated measurements and the filled (black) squares are the calibrated measurements. The calibration reduces the average error of the cross sections from 10.6% for the uncalibrated data to only 1.6% for the calibrated data.
FIG. 6 The measured σext for Cargille IL 1160 without calibration (circles [red]) and with calibration (squares [black]). The light (red) and dark (black) lines are the best-fit Mie curves for the uncalibrated (m=1.496+0.018i) and calibrated (m=1.517+0.000i) data, respectively. The shaded areas represent the uncertainty on each fit. The dashed line is the Mie curve for m=1.515+0.000i, the manufacturer's specified index of refraction for IL 1160. (Color figure available online.)
![FIG. 6 The measured σext for Cargille IL 1160 without calibration (circles [red]) and with calibration (squares [black]). The light (red) and dark (black) lines are the best-fit Mie curves for the uncalibrated (m=1.496+0.018i) and calibrated (m=1.517+0.000i) data, respectively. The shaded areas represent the uncertainty on each fit. The dashed line is the Mie curve for m=1.515+0.000i, the manufacturer's specified index of refraction for IL 1160. (Color figure available online.)](/cms/asset/92fc3b4f-008c-4600-aa52-b08803e934a6/uast_a_805875_o_f0006g.jpg)
In determining the best-fit index of refraction for each data set, the Mie cross sections were calculated for values of n spanning the range 1.300–1.700 and k spanning the range 0.000–0.200 and were weighted to account for the size distribution selected by the DMA with an assumed geometric standard deviation of σ g =1.05. The combination of n and k that yielded the lowest χ2 value when compared to the measured cross sections (using Equation (4)) was deemed to be the best fit. The best fit for the uncalibrated data is m=1.496(±0.006)+0.018i(+0.009i −0.007i ) and is represented in by the light (red) curve, while the best fit for the calibrated data is m=1.517(+0.004 −0.005)+0.000i(+0.001i −0.000i ), represented by the dark (black) curve. The dashed line represents the Mie curve for the manufacturer's specified index of refraction, m=1.515+0.000i. In each case, the uncertainties are the 95% confidence intervals and comprise all values of m for which χ2<(χ2 0+3.84), where χ2 0 is the value of χ2 for the best fit. The ranges of cross sections included by these uncertainties are represented by the shaded regions. It is worth noting that the quoted uncertainties on the fit to the calibrated data do not include uncertainties on the calibration parameters, Cf and Δd, or on the index of refraction of the squalane calibration standard. Propagating these uncertainties through to the calculated Mie cross sections is not straightforward and will be treated in a separate paper.
It is clear that the calibration leads to much more accurate cross sections and retrieved index of refraction. Without calibration—in essence assuming that the CPC counting efficiency was 100%, that the value of RL used in Equation (1) was correct, and that the geometric mean diameter selected by the DMA was accurate—the measured cross sections (open [red] circles) are an average of 10.6% larger than the Mie calculated cross sections using the manufacturer's specified index of refraction (dashed curve). With calibration, the cross sections (filled [black] squares) are only an average of 1.6% different from the Mie calculations (dashed curve). This improvement in accuracy leads to a more accurate retrieved index of refraction; using the calibrated data, the best fit, m=1.517+0.000i, is in very good agreement with the manufacturer's specified value, m=1.515+0.000i. On the contrary, without calibration the best fit is m=1.496+0.018i. Despite the inaccurate retrieval of m, the agreement between the uncalibrated σext and the corresponding best-fit Mie curve (light [red] line) is reasonable with an average absolute difference of only 2.2%. In other words, it is possible to obtain a good fit to the uncalibrated cross sections with an incorrect index of refraction. What is more, the observed level of agreement with the incorrect fit is at least as good as many of the fits published previously using A-CRDS in which errors range from 2% to 11% (Pettersson et al. Citation2004; Lang-Yona et al. Citation2009; Radney et al. Citation2009; Miles et al. Citation2010a). As this example illustrates, it is important to identify the sources of systematic errors and account for them with a calibration technique such as described here.
3.5.2. Impact on Atmospheric Radiative Forcing Estimates
Accurate calculations of radiative forcing by aerosols require that their indices of refraction be known accurately. In this section, the results from the analysis of Cargille IL 1160 in the preceding section are used to estimate the effect that A-CRDS measurement errors can have on radiative forcing calculations. Of course, atmospheric particles do not contain Cargille IL 1160, but it is useful here as a model for ambient aerosols.
In the preceding section, the systematic errors in the A-CRDS measurements, especially those related to the sizing and counting of particles, led to an incorrect best fit for the complex index of refraction which indicates that the IL 1160 particles are absorbing (k=0.018) even though they are known to be purely scattering. As a result of such an error, the calculated single scattering albedo, , is too small; for example, for 100 nm particles Ω = 0.76 instead of 1.00. A significant error in the calculated radiative forcing by such particles (if they were to exist in the atmosphere) would result. Using the approach of Haywood and Shine (Haywood and Shine Citation1995) as others have done (Andrews et al. Citation2006; Bond and Bergstrom Citation2006; Dinar et al. Citation2008), the magnitude of this impact can be estimated by calculating the radiative forcing efficiency (RFE) at the top of the atmosphere, defined as the radiative forcing (ΔF) normalized by aerosol optical depth (τ):
With this approach it is possible to assess the potential effect that an error in the measured index of refraction of Cargille IL 1160 has on predicted radiative forcing. For example, if the uncalibrated data are used (m=1.496+0.018i) instead of the calibrated data (m=1.517+0.000i), the RFE for 100 nm particles is estimated to be −28.1 W/m2 instead of −40.5 W/m2. Thus, both calculations would predict the aerosols to have a cooling effect, but the uncalibrated data lead to significantly less predicted cooling in the atmosphere. In making these RFE estimates, the spectral dependence of aerosol optical properties (i.e., Ω and β) has been neglected, and only the values at a single wavelength (355 nm) have been used. Though it omits important wavelength dependence, this approach has been used by many others (Anderson et al. Citation1999; Sheridan and Ogren Citation1999; Dinar et al. Citation2008) to provide a zero-order estimate of radiative forcing efficiency. Further, it has been assumed that the particles contain no water; inclusion of water will reduce the RFE through changes in both the effective index of refraction and the particle diameter. Nonetheless, the use of Equation (5) makes it possible to evaluate how errors in aerosol index of refraction, and ultimately errors in measurements of the extinction cross sections made using A-CRDS, propagate into errors in estimating climate forcing.
Similarly, errors in the indices of refraction of species that are prevalent in atmospheric particles will propagate into radiative forcing calculations. For example, Rudich and coworkers (Bluvshtein et al. Citation2012) recently used A-CRDS with particles of ammonium sulfate, a common constituent of ambient aerosols, and derived an index of refraction of m=1.517+0.018i at 532 nm even though it is known to be minimally absorbing (e.g., k⩽10−7 at 535 nm [Toon et al. Citation1976]). As with the Cargille IL 1160 results, the error in the retrieved index of refraction leads to significant underestimation of the cooling in the atmosphere; for 100 nm particles a RFE of −15.1 W/m2 is calculated compared to a RFE of −35.9 W/m2 if the accepted index of refraction of ammonium sulfate (m=[1.52 – 1.54]+0.00i at 535 nm) (Toon et al. Citation1976) is used.
In each of these examples, the effect that an error in the retrieved index of refraction has on the RFE can be estimated because the index of refraction has been determined accurately previously with other methods. However, for more complex particles representing ambient aerosols, such as those containing humic-like substances (HULIS) (Dinar et al. Citation2008) or Suwannee River fulvic acid (Lang-Yona et al. Citation2009), or even ambient aerosols, themselves, the index of refraction is not known a priori. Consequently, it is difficult to assess the impact that errors in the A-CRDS cross-section measurements have on the retrieved index of refraction and the estimated radiative forcing. Use of a calibration procedure as outlined in this work can reduce such systematic errors.
4. CONCLUSION
A novel technique has been developed to calibrate and reduce the systematic errors in extinction cross section measurements using A-CRDS. Squalane was selected as a convenient calibration liquid for this application because of its low volatility and the fact that its index of refraction has been measured previously at 355 nm. The possibility of using another liquid as a calibration substance is only limited by the ability to make a stable aerosol and the uncertainty in its known refractive index. Measuring extinction cross sections of the calibration aerosol permits the simultaneous determination of two calibration parameters, Cf and Δd, accounting for instrument calibration errors and daily changes in aerosol flow conditions. It was found that the Cf parameter, which scales the measured σext data, can be attributed mainly to the CPC counting efficiency error and the aerosol flow. The other parameter, Δd, is associated primarily with the operation of the DMA. Consequently, this A-CRDS calibration procedure provides a way to account for systematic errors in the operation of these instruments for which calibration methods are not readily available.
The improved accuracy in measuring the optical properties of substances afforded by this technique will be particularly beneficial for laboratory A-CRDS studies in which small, yet significant, differences in extinction are to be resolved; for example, investigations of minimally-absorbing substances, coated particles, or other internally-mixed aerosols. Future studies will consider the uncertainty in the index of refraction of the calibration standard (e.g., squalane) and how it propagates to the two calibration factors, Cf and Δd, and ultimately to the retrieved complex index of refraction of other samples.
Acknowledgments
The authors gratefully acknowledge support for this research from the National Science Foundation (AGS-0547011). They would also like to thank Professor Ephraim Woods, III of Colgate University for assistance in constructing the cavity ring-down cell, Professor Glenn Smith of the Georgia Institute of Technology for helpful discussions regarding cavity ring-down spectroscopy, and Jeff Baker from TSI, Inc., for loan of a CPC.
REFERENCES
- Anderson , T. L. , Covert , D. S. , Wheeler , J. D. , Harris , J. M. , Perry , K. D. Trost , B. E. 1999 . Aerosol Backscatter Fraction and Single Scattering Albedo: Measured Values and Uncertainties at a Coastal Station in the Pacific Northwest . J. Geophys. Res. , 104 : 26,793 – 26,807 .
- Andrews , E. , Sheridan , P. , Fiebig , M. , McComiskey , A. , Ogren , J. Arnott , P. 2006 . Comparison of Methods for Deriving Aerosol Asymmetry Parameter . J. Geophys. Res. , 111 : D05S04 doi:10.1029/2004JD005734
- Anttila , T. , Vaattovaara , P. , Komppula , M. , Hyvarinen , A. P. , Lihavainen , H. and Kerminen , V. M. 2009 . Size-Dependent Activation of Aerosols Into Cloud Droplets at a Subarctic Background Site During the Second Pallas Cloud Experiment (2nd Pace): Method Development and Data Evaluation . Atmos. Chem. Phys. , 9 : 4,841 – 4,854 .
- Attwood , A. and Greenslade , M. 2012 . Deliquescence Behavior of Internally Mixed Clay and Salt Aerosols by Optical Extinction Measurements . J. Phys. Chem. A , 116 : 4,518 – 4,527 .
- Baron , P. A. and Willeke , K. 2001 . Aerosol Measurement: Principles, Techniques, and Applications (2nd ed.) , New York : Wiley .
- Baynard , T. , Lovejoy , E. R. , Pettersson , A. , Brown , S. S. , Lack , D. Osthoff , H. 2007 . Design and Application of a Pulsed Cavity Ring-down Aerosol Extinction Spectrometer for Field Measurements . Aerosol Sci. Technol. , 41 : 447 – 462 .
- Beaver , M. R. , Garland , R. M. , Hasenkopf , C. A. , Baynard , T. , Ravishankara , A. R. and Tolbert , M. A. 2008 . A Laboratory Investigation of the Relative Humidity Dependence of Light Extinction by Organic Compounds from Lignin Combustion . Environ. Res. Lett. , 3 : 045003 doi:10.1088/1748-9326/3/4/045003
- Berden , G. and Engeln , R. 2009 . Cavity Ring-Down Spectroscopy: Techniques and Applications , Oxford : Wiley .
- Bluvshtein , N. , Flores , J. M. , Riziq , A. A. and Rudich , Y. 2012 . An Approach for Faster Retrieval of Aerosols’ Complex Refractive Index Using Cavity Ring-Down Spectroscopy . Aerosol Sci. Technol. , 46 : 1,140 – 1,150 .
- Bohren , C. F. and Huffman , D. R. 1998 . Absorption and Scattering of Light by Small Particles , New York : Wiley .
- Bond , T. C. and Bergstrom , R. 2006 . Light Absorption by Carbonaceous Particles: An Investigative Review . Aerosol Sci. Technol. , 40 : 27 – 67 .
- Born , M. and Wolf , E. 1999 . Principles of Optics: Electromagnetic Theory of Propagation, Interference and Diffraction of Light (7th ed.) , Cambridge , , UK : Cambridge University Press .
- Brem , B. T. , Gonzalez , F. C. M. , Meyers , S. R. , Bond , T. C. and Rood , M. J. 2012 . Laboratory-measured Optical Properties of Inorganic and Organic Aerosols at Relative Humidities up to 95% . Aerosol Sci. Technol. , 46 : 178 – 190 .
- Bulatov , V. , Fisher , M. and Schechter , I. 2002 . Aerosol Analysis by Cavity-ring-down Laser Spectroscopy . Anal. Chim. Acta , 466 : 1 – 9 .
- Busch , K. W. and Busch , M. A. 1999 . Cavity Ringdown Spectroscopy: An Ultratrace-Absorption Measurement Technique , Washington , DC : Oxford University Press .
- Dinar , E. , Riziq , A. A. , Spindler , C. , Erlick , C. , Kiss , G. and Rudich , Y. 2008 . The Complex Refractive Index of Atmospheric and Model Humic-like Substances (HULIS) Retrieved by a Cavity Ring Down Aerosol Spectrometer (CRD-AS) . Faraday Discuss , 137 : 279 – 295 .
- Duke , S. D. and Layendecker , E. B. 2003 . Technical note tn-010.02a: Internal standard method for size calibration of sub-micrometer spherical particles by electron microscopy Technical report. Thermo Scientific, Freemont, CA, USA
- Everest , M. A. and Atkinson , D. B. 2008 . Discrete Sums for the Rapid Determination of Exponential Decay Constants . Rev. Sci. Instrum. , 79 : 023108 doi:10.1063/1.2839918
- Freedman , M. A. , Hasenkopf , C. A. , Beaver , M. R. and Tolbert , M. A. 2009 . Optical Properties of Internally Mixed Aerosol Particles Composed of Dicarboxylic Acids and Ammonium Sulfate . J. Phys. Chem., A , 113 : 13584 – 13592 .
- Halmer , D. , von Basum , G. , Hering , P. and Murtz , M. 2004 . Fast Exponential Fitting Algorithm for Real-time Instrumental Use . Rev. Sci. Instrum. , 75 : 2,187 – 2,191 .
- Haltrin , V. I. 2002 . One-parameter Two-term Henyey-Greenstein Phase Function for Light Scattering in Seawater . Appl. Opt. , 41 : 1,022 – 1,028 .
- Harmon , C. W. , Grimm , R. L. , McIntire , T. M. , Peterson , M. D. , Njegic , B. Angel , V. M. 2010 . Hygroscopic Growth and Deliquescence of NaCl Nanoparticles Mixed with Surfactant SDS . J. Phys. Chem. B , 114 : 2,435 – 2,449 .
- Haywood , J. and Shine , K. 1995 . The Effect of Anthropogenic Sulfate and Soot Aerosol on the Clear-sky Planetary Radiation Budget . Geophys. Res. Lett. , 22 : 603 – 606 .
- Hering , S. V. and McMurry , P. H. 1991 . Optical Counter Response to Monodisperse Atmospheric Aerosols . Atmos. Environ., Part A , 25 : 463 – 468 .
- Hinds , W. C. 1999 . Aerosol Technology: Properties, Behavior, and Measurement of Airborne Particles , New York : Wiley .
- Khalizov , A. F. , Xue , H. X. , Wang , L. , Zheng , J. and Zhang , R. Y. 2009 . Enhanced Light Absorption and Scattering by Carbon Soot Aerosol Internally Mixed with Sulfuric Acid . J. Phys. Chem. A , 113 : 1,066 – 1,074 .
- Kim , J. H. , Mulholland , G. W. , Kukuck , S. R. and Pui , D. Y. H. 2005 . Slip Correction Measurements of Certified PSL Nanoparticles Using a Nanometer Differential Mobility Analyzer (nano-DMA) for Knudsen Number from 0.5 to 83 . J. Res. Natl. Inst. Stand. Technol. , 110 : 31 – 54 .
- Kinney , P. D. , Pui , D. Y. H. , Mulholland , G. W. and Bryner , N. P. 1991 . Use of the Electrostatic Classification Method to Size 0.1 μm SRM Particles—a Feasibility Study . J. Res. Natl. Inst. Stand. Technol. , 96 : 147 – 176 .
- Köhler , S. , Allan , M. , Kelso , H. , Henderson , D. and McKendrick , K. 2005 . The Effects of Surface Temperature on the Gas-Liquid Interfacial Reaction Dynamics of O(3P) Plus Squalane . J. Chem. Phys. , 122 : 024712 doi:10.1063/1.1835268
- Lack , D. A. , Cappa , C. D. , Cross , E. S. , Massoli , P. , Ahern , A. T. Davidovits , P. 2009 . Absorption Enhancement of Coated Absorbing Aerosols: Validation of the Photo-acoustic Technique for Measuring the Enhancement . Aerosol Sci. Technol. , 43 : 1,006 – 1,012 .
- Lang-Yona , M. , Rudich , Y. , Segre , E. , Dinar , E. and Abo-Riziq , A. 2009 . Complex Refractive Indices of Aerosols Retrieved by Continuous Wave-cavity Ring Down Aerosol Spectrometer . Anal. Chem. (Washington, DC, U. S.) , 81 : 1,762 – 1,769 .
- Lee , D. H. , Yoon , Y. , Kim , B. , Lee , J. Y. , Yoo , Y. S. and Hahn , J. W. 2002 . Optimization of the Mode Matching in Pulsed Cavity Ringdown Spectroscopy by Monitoring Non-degenerate Transverse Mode Beating . Appl. Phys. B: Lasers Opt. , 74 : 435 – 440 .
- Li , L. , Chen , J. M. , Chen , H. , Yang , X. , Tang , Y. and Zhang , R. Y. 2011 . “Monitoring Optical Properties of Aerosols with Cavity Ring-down Spectroscopy . J. Aerosol Sci. , 42 : 277 – 284 .
- Lu , J. W. , Flores , J. M. , Lavi , A. , Abo-Riziq , A. and Rudich , Y. 2011 . Changes in the Optical Properties of Benzo[a]pyrene-coated Aerosols Upon Heterogeneous Reactions with NO2 and NO3 . Phys. Chem. Chem. Phys. , 13 : 6,484 – 6,492 .
- Mellon , D. , King , S. J. , Kim , J. , Reid , J. P. and Orr-Ewing , A. J. 2011 . Measurements of Extinction by Aerosol Particles in the Near-infrared Using Continuous Wave Cavity Ring-down Spectroscopy . J. Phys. Chem. A , 115 : 774 – 783 .
- Miles , R. E. H. , Rudic , S. , Orr-Ewing , A. J. and Reid , J. P. 2010a . Influence of Uncertainties in the Diameter and Refractive Index of Calibration Polystyrene Beads on the Retrieval of Aerosol Optical Properties Using Cavity Ring Down Spectroscopy . J. Phys. Chem. A , 114 : 7,077 – 7,084 .
- Miles , R. E. H. , Rudic , S. , Orr-Ewing , A. J. and Reid , J. P. 2010b . Measurements of the Wavelength Dependent Extinction of Aerosols by Cavity Ring Down Spectroscopy . Phys. Chem. Chem. Phys. , 12 : 3,914 – 3,920 .
- Mulholland , G. W. , Donnelly , M. K. , Hagwood , C. R. , Kukuck , S. R. , Hackley , V. A. and Pui , D. Y. H. 2006 . Measurement of 100 nm and 60 nm Particle Standards by Differential Mobility Analysis . J. Res. Natl. Inst. Stand. Technol. , 111 : 257 – 312 .
- O’Keefe , A. and Deacon , D. A. G. 1988 . Cavity Ring-down Optical Spectrometer for Absorption Measurements Using Pulsed Laser Sources . Rev. Sci. Instrum. , 59 : 2,544 – 2,551 .
- Painter , L. R. , Attrey , J. S. , Hubbell , H. H. and Birkhoff , R. D. 1984 . Vacuum Ultraviolet Optical Properties of Squalane and Squalene . J. Appl. Phys. , 55 : 756 – 759 .
- Pettersson , A. , Lovejoy , E. R. , Brock , C. A. , Brown , S. S. and Ravishankara , A. R. 2004 . Measurement of Aerosol Optical Extinction at 532 nm with Pulsed Cavity Ring Down Spectroscopy . J. Aerosol Sci. , 35 : 995 – 1,011 .
- Press , W. H. , Teukolsky , S. , Vetterling , W. and Flannery , B. 2007 . Numerical Recipes: The Art of Scientific Computing (3rd ed.) , New York : Cambridge University Press .
- Radney , J. G. , Bazargan , M. H. , Wright , M. E. and Atkinson , D. B. 2009 . Laboratory Validation of Aerosol Extinction Coefficient Measurements by a Field-deployable Pulsed Cavity Ring-down Transmissometer . Aerosol Sci. Technol. , 43 : 71 – 80 .
- Riziq , A. A. , Erlick , C. , Dinar , E. and Rudich , Y. 2007 . Optical Properties of Absorbing and Non-absorbing Aerosols Retrieved by Cavity Ring Down (CRD) Spectroscopy . Atmos. Chem. Phys. , 7 : 1,523 – 1,536 .
- Robock , A. 1980 . The Seasonal Cycle of Snow Cover, Sea Ice and Surface Albedo . Mon. Wea. Rev. , 108 : 267 – 285 .
- Sappey , A. D. , Hill , E. S. , Settersten , T. and Linne , M. A. 1998 . Fixed-frequency Cavity Ringdown Diagnostic for Atmospheric Particulate Matter . Opt. Lett. , 23 : 954 – 956 .
- Scherer , J. J. , Paul , J. B. , OKeefe , A. and Saykally , R. J. 1997 . Cavity Ringdown Laser Absorption Spectroscopy: History, Development, and Application to Pulsed Molecular Beams . Chem. Rev. (Washington, DC, U. S.) , 97 : 25 – 51 .
- Sheridan , P. J. and Ogren , J. A. 1999 . Observations of the Vertical and Regional Variability of Aerosol Optical Properties Over Central and Eastern North America . J. Geophys. Res. , 104 : 16793–16805
- Sörensen , N. A. , Gillebo , T. , Holtermann , H. and Sorensen , J. S. 1951 . Studies Related to Pristane.6. Synthesis of Digeranyl with Some Remarks on the Physical Constants of Crocetane . Acta Chem. Scand. (1947–1973) , 5 : 757 – 765 .
- Spindler , C. , Riziq , A. A. and Rudich , Y. 2007 . Retrieval of Aerosol Complex Refractive Index by Combining Cavity Ring Down Aerosol Spectrometer Measurements with Full Size Distribution Information . Aerosol Sci. Technol. , 41 : 1,011 – 1,017 .
- Thompson , J. E. , Smith , B. W. and Winefordner , J. D. 2002 . Monitoring Atmospheric Particulate Matter Through Cavity Ring-down Spectroscopy . Anal. Chem. (Washington, DC, U. S.) , 74 : 1,962 – 1,967 .
- Toon , O. B. , Pollack , J. B. and Khare , B. N. 1976 . The Optical Constants of Several Atmospheric Aerosol Species: Ammonium Sulfate, Aluminum Oxide, and Sodium Chloride . J. Geophys. Res. , 81 : 5733–5748
- Trainic , M. , Riziq , A. A. , Lavi , A. and Rudich , Y. 2012 . Role of Interfacial Water in the Heterogenous Uptake of Glyoxal by Mixed Glycine and Ammonium Sulfate Aerosols . J. Phys. Chem. A , 116 : 5,948 – 5,957 .
- TSI, Inc. 2010 . CPC Operation and Service Manual (Rev. G) Model 3775. Shoreview, MN, USA
- Van de Hulst , H. 1957 . Light Scattering by Small Particles , New York : John Wiley & Sons Inc. .
- Vander Wal , R. L. and Ticich , T. M. 1999 . Cavity Ringdown and Laser-induced Incandescence Measurements of Soot . Appl. Opt. , 38 : 1,444 – 1,451 .
- Wheeler , M. D. , Newman , S. M. , Orr-Ewing , A. J. and Ashfold , M. N. R. 1998 . Cavity Ring-down Spectroscopy . J. Chem. Soc., Faraday Trans. , 94 : 337 – 351 .
- Wiedensohler , A. 1988 . An Approximation of the Bipolar Charge-distribution for Particles in the Sub-micron Size Range . J. Aerosol Sci. , 19 : 387 – 389 .
- Wiscombe , W. J. and Grams , G. W. 1976 . The Backscattered Fraction in Two-stream Approximations . J. Atmos. Sci. , 33 : 2,440 – 2,451 .
- Wriedt , T. Mie Type Codes in Electromagnetic Scattering Programs . Retrieved March 19, 2013, from http://diogenes.iwt.uni-bremen.de/vt/laser/wriedt/Mie_Type_Codes/body_mie_type_codes.html
- Yamada , Y. , Miyamoto , K. and Koizumi , A. 1985 . Size Determination of Latex Particles by Electron Microscopy . Aerosol Sci. Technol. , 4 : 227 – 232 .
- Zalicki , P. and Zare , R. N. 1995 . Cavity Ring-down Spectroscopy for Quantitative Absorption Measurements . J. Chem. Phys. , 102 : 2,708 – 2,717 .
- Zarzana , K. J. , De Haan , D. O. , Freedman , M. A. , Hasenkopf , C. A. and Tolbert , M. A. 2012 . Optical Properties of the Products of α-dicarbonyl and Amine Reactions in Simulated Cloud Droplets . Environ. Sci. Technol. , 46 : 4,845 – 4,851 .