Abstract
Laboratory experiments were conducted to assess the suitability of photoacoustic spectroscopy (PAS) for aerosol absorption measurement at high relative humidity (RH). Initial experiments characterized the PAS sensitivity that increased strongly by ∼1.25 between dry conditions and 90% RH. Correction procedures were validated by measuring RH-independent absorption for hydrophobic absorbing particles. Absorption measured by PAS for a range of hygroscopic particles, including different morphologies, hygroscopicities, and absorbing entities, showed strong low biases at high RH (down to 0.4 at 90% RH). The bias was due to water evaporation impacting the PAS signal. Cooling samples to lower absolute humidity while maintaining a constant RH did not significantly reduce the bias magnitude within the temperature range 11–25°C. The magnitude and RH dependence of the bias were not reproduced well using a model of PAS response incorporating coupled heat and mass transfer in the transition regime. This was attributed, in part, to uncertainty related to changes in the water mass accommodation coefficient with RH. Given our inability to correct for evaporation-induced bias effects accurately, or reduce their magnitude experimentally, we conclude that PAS is not a technique well suited to the measurement of absorption at high RH. In order to minimize RH-related errors in PAS measurements made under notionally “dry” conditions, we recommend operation in the RH range 10–30%.
Copyright 2013 American Association for Aerosol Research
1. INTRODUCTION
Atmospheric particles significantly impact the climate system through direct absorption and scattering of solar radiation (IPCC Citation2007; Stier et al. Citation2007; Ramanathan and Carmichael Citation2008). A clear understating of the optical behavior of atmospheric aerosol is therefore important for accurate climate prediction (Schwartz Citation2004). At a fundamental level, the extent to which a particle absorbs radiation at a particular wavelength is determined by its refractive index, size, and morphology (Bohren and Huffman Citation1983). As particles take up water in response to elevated relative humidity (RH), these properties are modified, which can impact optical behavior significantly.
During the last four decades, many different groups have examined the RH dependence of aerosol scattering through laboratory and field-based work (Covert et al. Citation1975; Rood et al. Citation1987; Carrico et al. Citation2003; Malm et al. Citation2005; Baynard et al. Citation2006; Jeong et al. Citation2007). These studies provided much of the information needed to represent the impact of water uptake on scattering in atmospheric models (Quinn et al. Citation2005; Ghan and Zaveri Citation2007). In contrast, little effort has been directed toward measuring changes in aerosol absorption at high RH, due in part to a lack of capable instrumentation. Atmospheric models most often assume that absorption is independent of RH despite incomplete evidence to support this conclusion. Indeed, idealized calculations for particles representative of aged black carbon (BC; the most strongly absorbing aerosol in the atmosphere; Ramanathan and Carmichael Citation2008) predict significant absorption enhancements at high RH. For example, Redemann et al. (Citation2001) predicted absorption by aged BC to increase by 1.35 between dry conditions and 95% RH. The mechanism for this enhancement is one in which water behaves as a lens to focus radiation into the absorbing BC core (Jacobson Citation2001; Bond et al. Citation2006). For morphologies other than a coated core, such as one might expect for homogenous mixing of a partially soluble absorbing entity (e.g., “brown carbon”) with water, enhanced absorption is also predicted by simple Mie-based calculations (Bohren and Huffman Citation1983).
In recent years, there has been growing interest in the experimental investigation of aerosol absorption at high RH. This research has primarily used indirect methods to determine absorption. For example, Brem et al. (Citation2012) deduced absorption as a function of RH from the difference in extinction and scattering measured for smoke generated from wood pyrolysis. They found that absorption at 530 nm was enhanced by 2.7 ± 1.2 between 32 and 95% RH. Khalizov et al. (Citation2009) also employed an extinction–scattering difference-based method to infer absorption in laboratory studies on sulfuric acid-coated flame soot. They measured absorption enhancements of up to 1.4 at 80% RH compared to dry conditions. Flores et al. (Citation2012) used cavity ringdown extinction measurements to derive the complex refractive index of laboratory-generated, size-selected absorbing aerosol at high RH. Their measured complex refractive indices agreed well with predictions derived for the composite mixtures using the volume-weighted mixing rule (Chylek et al. Citation1988).
Methods for the direct measurement of aerosol absorption at high RH, namely, photoacoustic spectroscopy (PAS), have thus far been less successfully demonstrated. Arnott et al. (Citation2003) conducted field measurements using PAS at the Southern Great Plains Cloud and Radiation Testbed Site, OK, USA. Results indicated a systematic low bias of absorption measured at high RH, which was tentatively attributed to the effects of water evaporation on the photoacoustic signal. Lewis et al. (Citation2009) conducted PAS measurements on biomass emissions from controlled burns and found a similar low-biased absorption at high RH. These authors suggested that the low bias might have been due to water evaporation effects or a change in BC morphology with water uptake (fractal core collapsing). Lack et al. (Citation2009) used PAS to measure the RH dependence of absorption by Saharan mineral dust that had undergone long-range transport to the Gulf of Mexico. They measured absorption enhancements of up to 1.5 between 25 and 75% RH and estimated that the effects of water evaporation caused a low bias of 7–30%.
Several theoretical studies have examined the impact of water evaporation on PAS aerosol measurements (Arnott et al. Citation2003; Raspet et al. Citation2003; Murphy Citation2009). Of particular relevance to this work, Murphy (Citation2009) developed a theoretical framework to estimate the magnitude of the bias by solving the requisite coupled heat and mass transfer equations in the transition flow regime. His work showed a complex dependence on both the particle properties (e.g., size, water mass accommodation coefficient αM ) and ambient environmental conditions (e.g., pressure, absolute humidity). Importantly, it indicated that absorption measurements at high RH may not be significantly biased by evaporation effects if the absolute (as opposed to relative) humidity is kept sufficiently low.
The work presented here sets out to provide the first experiment-based evaluation of the PAS technique for aerosol absorption measurements at high RH. The study is intended to complement the theoretical work that has preceded it by providing direct observations of absorption by well-characterized aerosol exposed to a variety of environmental conditions. Particular foci include a thorough evaluation of factors impacting PAS measurements at high RH, measurement of absorption-RH profiles for a range of homogenously mixed and core-coat absorbing systems, and comparison to theoretical predictions made using the model framework of Murphy (Citation2009).
2. EXPERIMENTAL
The basic principles of the PAS technique applied to aerosol measurements have been discussed at length previously (e.g., Arnott et al. Citation2006) and are only briefly covered here. The photoacoustic technique involves exposing an absorbing aerosol to an intensity-modulated laser beam. Particles undergo period cycles of heating (driven by absorption of laser radiation) and cooling (driven by conduction into the gas phase), which generates a pressure (sound) wave in the gas phase at the laser modulation frequency. The sound wave is amplified by acoustic resonance in the sample cell and detected using a sensitive microphone. The microphone response generally varies linearly with absorption and can be readily calibrated. The situation is somewhat complicated by the presence of semi-volatile aerosol components such as water. Here, evaporation from the heated aerosol consumes energy as latent heat that would otherwise have contributed to the generation of sound. This additional energy loss mechanism leads to the low bias of PAS signals.
illustrates the experimental apparatus developed for this study, which comprised three main sections: aerosol generation, conditioning, and detection.
FIG. 1 Schematic of the apparatus used to probe the RH dependence of aerosol light absorption. DMA: Differential mobility analyzer; PAS: photoacoustic spectrometer; CRDS: cavity ringdown spectrometer; CPC: condensation particle counter; P: pressure; T: temperature.
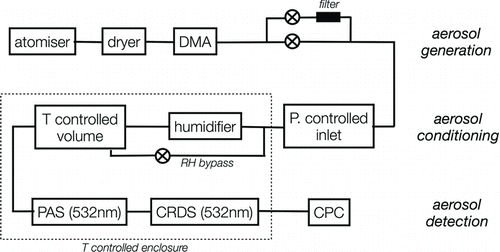
2.1. Aerosol Generation
We studied three hygroscopic particle systems: nigrosin dye, nigrosin dye–ammonium sulfate internal mixtures, and dyed polystyrene latex spheres (dyed PSL, Thermo Scientific, Boston, MA, USA) coated in ammonium sulfate. These aerosols were generated using an atomizer (Liu and Lee Citation1975) and dried using silica gel to an RH < 15%. A differential mobility analyzer (DMA; Knutson and Whitby Citation1975) then size-selected the aerosol to produce a pseudo-monodisperse population.
Nigrosin (C48N9H51) is an organic dye often used in laboratory systems as a proxy for absorbing atmospheric aerosol (Lack et al. Citation2006; Brem et al. Citation2012; Flores et al. Citation2012). It is moderately hygroscopic and has a known refractive index at 532 nm (). Nigrosin aerosol was generated using a solution concentration of 6.7 g L−1 water.
TABLE 1 Optical and physical properties of pure compounds. The κ parameter (Petters and Kreidenweis Citation2007) is used to describe hygroscopic growth behavior
Nigrosin–ammonium sulfate mixtures were studied to provide an absorbing aerosol with stronger water uptake than pure nigrosin. To investigate the degree to which these components were internally mixed within individual particles, size-selected particles were analyzed using an Ultra-High Sensitivity Aerosol Spectrometer (UHSAS; Droplet Measurement Technologies, Boulder, CO, USA) that was modified to optically size particles at elevated RH. The UHSAS was modified by introducing a temperature-controlled Nafion-humidifier (MH-110, Perma Pure, Toms River, NJ, USA) into the sample flow line, which enabled the sample RH within the optical sensing volume to be varied in the range 60–90% with an uncertainty in determination of the actual RH of +5%, −7%. Using this technique, we observed a single size mode under dry conditions (RH ∼ 15%) that shifted uniformly to larger sizes at high RH (RH ∼ 85%). This uniform growth indicated that the two chemical components were mixed within individual particles. Two nigrosin–ammonium sulfate solution concentrations were used: 3.3:8.9 g L−1 (solution 1) and 3.3:3.3 g L−1 (solution 2) water.
Absorbing dyed PSL were coated in ammonium sulfate to yield an aerosol with a core-coat morphology and an absorber other than nigrosin. The coating of a hydrophobic core material with an optically transparent, hydrophilic substance provided a proxy system for absorption by aged atmospheric BC and mineral dust. Particles were coated by atomizing monodisperse dyed PSL from a concentrated solution of ammonium sulfate.
The successful application of coatings was verified in the following way: first, working with a DMA set-point diameter of 690 nm, we confirmed that as expected no transmission of 600 nm diameter dyed PSL (atomized from pure distilled water) was observed through the system. We then conducted a similar experiment using a pure ammonium sulfate solution containing no dyed PSL. For a solution concentration of 5 g L−1, we observed no particle transmission with the DMA size selecting at 690 nm. Upon mixing the ammonium sulfate and dyed PSL, a strong signal at 690 nm appeared, indicating the successful application of ammonium sulfate coatings. We note that it is highly unlikely that the coatings were uniformly applied at the 45 nm thickness selected by the DMA. However, possible coating nonuniformity was not a critical limitation for this work.
As is common when sizing aerosol through differential mobility analysis, in addition to singly charged particles at the desired diameter, larger, multiply charged particles were also transmitted in these experiments. This phenomenon was accounted for explicitly as follows: first, the full aerosol size distribution exiting the drier was measured by operating the DMA in a scanning mode (Wang and Flagan Citation1989). This information was then combined with the normalized DMA transfer function calculated at the diameter set-point voltage (Knutson and Whitby Citation1975; Wiedensohler Citation1988 with subsequent erratum) to yield the full distribution of particles exiting the DMA. Across all experiments conducted, multiply charged particles contributed at most 25% by number to the total particle population. Full size distributions including multiply charged particles were used for all calculations presented.
The physical and optical properties of the aforementioned pure compounds are drawn upon extensively for model calculations in the following sections. They are summarized in .
2.2. Aerosol Conditioning
The sample flow was periodically diverted through an automated filter assembly to acquire aerosol-free background measurements. A simple pressure-controlled inlet comprising an 800 μm diameter orifice (O’Keefe Controls, Trumbull, CT, USA) and variable flow rate bypass provided control of the downstream system pressure. All measurements presented here were performed close to ambient pressure in the range 803– 824 mb.
Acoustic filters in the sample line were used to reduce background flow noise. After the acoustic filters, the sample entered a thermally and acoustically insulated temperature-controlled enclosure. The enclosure comprised an aluminum optical baseplate that was cooled to 18°C by a temperature-regulated water flow (LC-200, TE technology, Traverse City, MI, USA). Upon entering the box, the sample passed through a Nafion-humidifier (MH-110, Perma Pure, Toms River, NJ, USA) that was housed within a temperature-regulated aluminum block. The temperature of the humidifier assembly was controlled to within 0.1°C using a set of three thermoelectric Peltier elements. The sample exited the humidifier close to saturation at the humidifier temperature and then entered a temperature-controlled aluminum volume (∼0.06 L), which provided a residence time of ∼6 s. The temperature of this volume was set to match that of the PAS detection cell and was intended to provide time for the aerosol to reach equilibrium at the measurement RH before detection (Sjogren et al. Citation2007). For the acquisition of dry aerosol measurements, a bypass line directed the flow around the humidifier and directly into the equilibrium volume.
2.3. Photoacoustic Absorption Measurement
The photoacoustic instrument comprised a laser source, optical chopper, multipass optical system, and acoustic resonator and was based closely on the design of Lack et al. (Citation2012). The laser source was a continuous wave (cw) diode pumped Nd:YAG that was frequency-doubled to emit radiation at 532 nm (GSF32–200P, MeshTel Intelite, Genoa, NV, USA). The maximum cw power output was 150 mW. Radiation was modulated using a computer-controlled optical chopper (MC2000, Thorlabs, Newton, NJ, USA) and directed into an astigmatic multipass cell designed to provide 182 passes through the acoustic resonator (Hao et al. Citation2002; Silver Citation2005). In practice, achieving this number of passes required careful alignment, which was not pursued in this work. Rather, optimization was achieved though visual inspection of the pattern density, likely resulting in a lower number of passes. The acoustic resonator incorporated optical windows for laser transmission (#48–924, Edmund Optics, Barrington, NJ, USA) and was placed within the multipass system. Each window was purged with a small flow (7 ccm) of dry particle-free air to prevent condensation. The resonator comprised dual ½-wave resonators capped at each end by ¼-wave volumes. Both resonators received sample flow, but only one was excited by the laser radiation. The other provided characterization of background noise for subtraction. Each resonator contained an electret microphone (EK-3132, Knowles Acoustics, Itasca, IL, USA) placed at an acoustic antinode at its center. A Knowles Acoustics EP-7369 speaker was placed in the background resonator and was used to measure the cell resonance frequency (Fr ) and quality factor (Q). The temperature of the photoacoustic cell was stabilized to within 0.1°C using a Peltier element. This temperature stabilization was needed to prevent drift in Fr during experiment runs. It also enabled measurements to be performed at low temperature. A range of housekeeping data associated with the photoacoustic measurement was collected including RH and temperature (HMP50, Vaisala, Helsinki, Finland), laser power (S120b, Thorlabs, Newton, NJ, USA), and pressure (PPT0015, Honeywell, Morristown, NJ, USA).
2.4. Cavity Ringdown Extinction Measurement
A cavity ringdown spectrometer (CRDS) measured aerosol extinction at elevated humidity (O’Keefe and Deacon Citation1988). Data from this instrument were primarily used to derive particle growth factors needed for absorption modeling (Section 3.2). The CRDS system was based on that described in Langridge et al. (Citation2011). Briefly, 532 nm radiation from a pulsed, frequency-doubled Nd:YAG (QL532–200, CrystaLaser, Reno, NV, USA) was coupled into a linear high finesse optical cavity. The cavity was assembled from two high reflectivity mirrors (ATFilms, Boulder, CO, USA) spaced 0.4 m apart. Each mirror was purged with a small flow (7 ccm) of aerosol-free synthetic air to protect against contamination. Light exiting the cavity was detected using a photomultiplier tube (HC120, Hamamatsu, Hamamatsu City, Shizuoka Pref., Japan) and the signal's exponential decay rate analyzed to determine extinction (Langridge et al. Citation2011). The average ringdown time (τ) for the particle-free cavity at 800 mb pressure was 80 μs. The CRDS was thermally isolated from the optical baseplate and was not temperature controlled. Thus, the CRDS RH was often slightly different from that measured in the PAS cell. It was monitored using a second HMP50 RH probe placed within the airflow at the center of the CRDS cavity.
2.5. Particle Counting, Losses, and Airflow
A Nafion dryer (PD-200, Perma Pure, Toms River, NJ, USA) was positioned at the exit of the temperature-controlled assembly to prevent condensation in external sample lines. A commercial condensation particle counter (CPC, 3022A, TSI, Shoreview, MN, USA) measured particle number concentrations. These measurements were primarily used to monitor aerosol generation stability during experiment runs.
Simple loss tests were carried out to determine differences in particle transmission through the instrument for operation in humidified and RH-bypass modes. They demonstrated that losses for submicron diameter sizes were less than 3%. Volumetric flow through the entire system was controlled at 0.6 lpm using a mass flow controller (MC-5LPM, Alicat Scientific, Tucson, AZ, USA). This relatively modest flow rate was chosen to limit the generation of acoustic noise in the sample flow lines.
2.6. Measurement Calibration
The PAS was calibrated using ozone absorption at levels determined online by the CRDS. Previous work has demonstrated the suitability of this calibration method for PAS instruments intended for aerosol measurements (Lack et al. Citation2006). Ozone was generated by passing pure oxygen through a set of two high power mercury lamps (90–0004–01, UVP, Upland, CA, USA). The oxygen flow rate was controlled in the range 2–10 ccm to provide multiple stable ozone concentrations.
3. DATA ANALYSIS
3.1. PAS Correction Procedures
This section outlines the basic steps needed to generate absorption-RH profiles (humidigrams) from raw PAS microphone measurements. EquationEquation (1) reproduced from Arnott et al. (Citation2006) shows the key factors linking the aerosol absorption coefficient, β abs, to the PAS microphone response, P mic:
Data were collected as a function of RH in stepwise fashion. At each RH, we first characterized the frequency response of the cell by filtering aerosol from the sample stream and acoustically exciting the resonator with a broadband chirp generated by the speaker. This measurement provided the cell Q and Fr . We then observed the background by turning the speaker off and modulating the laser at the observed Fr . Finally, particles were introduced into the cell and the response under laser-excitation measured. Data were typically collected for 75 s at each step and the system required 5–10 min to achieve RH stability between steps. Thus, the total acquisition time for a multipoint humidigram was on the order of several hours.
Data analysis proceeded as follows:
i. | The background P mic signal was subtracted from that measured for aerosol sampling. By far the largest contribution to background signal in the current system arose from absorption of laser radiation by the optical windows, which generated acoustic signal at Fr . The background signal was RH dependent, increasing linearly on average by 1.55 between dry conditions and 90% RH. This dependence was in part due to changes in microphone sensitivity with RH (Section 4.1). Adsorption of water onto the window surfaces (at levels insufficient to reduce the laser power transmitted through the resonator) likely also contributed. Background noise often accounted for a significant fraction of the total aerosol sample response. This fraction depended on the sample absorption strength and was up to 16% for experiments with pure nigrosin, 25% for nigrosin–ammonium sulfate mixtures, and 70% for ammonium sulfate-coated dyed PSL. Collecting background signal at each RH step was important as variability between experiments prevented effective use of a parameterized correction. | ||||
ii. | The background-corrected microphone response was multiplied by Fr /Q to correct for RH dependencies. Typically Fr /Q increased by ∼5% moving from dry conditions to 90% RH. | ||||
iii. | The product was then divided by the specific heats term γ−1, calculated following Arnott et al. (Citation2006). Typically this was a very small correction, decreasing by less than 1% between dry conditions to 90% RH. | ||||
iv. | A correction was then applied to account for changes in P laser during the measurement period. Owing to the high stability of the laser source used, this correction was typically <5%. | ||||
v. | The signal was next corrected for significant changes in microphone sensitivity observed with RH. The characterization of microphone sensitivity with RH comprised a key element of this work and is presented in detail in Section 4.1. | ||||
vi. | Finally, the above product was normalized by the aerosol number concentration to account for (typically minor) variations during the measurement period. |
The resulting β abs was not dimensioned on an absolute absorption scale. However, this was of no consequence given our interest in the enhancement of absorption at high RH, commonly termed f(RH)abs, which was calculated from the ratio of β abs measured at high RH to dry (low RH) conditions.
3.2. Model Calculations of Extinction at High RH
The enhancement in aerosol extinction at high RH, f(RH)ext, was derived from the raw CRDS measurements using well-established analysis procedures described in Langridge et al. (Citation2011). These data were used in this work to evaluate aerosol hygroscopic growth behavior through comparison to model calculations employing Mie theory (Bohren and Huffman Citation1983) and the κ-Köhler parameterization of particle growth (Petters and Kreidenweis Citation2007). In κ-Köhler theory, κ is a hygroscopicity parameter that can be directly related to the diameter growth factor, which is the necessary input for extinction calculations at elevated RH. Kreidenweis et al. (Citation2008) showed that κ-Köhler theory represents water uptake in multicomponent systems well at subsaturated conditions.
Model calculations of f(RH)ext proceeded as follows: (i) equilibrium particle diameters at a series of elevated RH levels were determined by applying κ-Köhler theory to the measured dry particle size distribution; (ii) size-resolved extinction at each elevated RH was calculated using Mie theory with the refractive indices of wet particles determined through volume weighting the refractive indices of water and the dry components (Chylek et al. Citation1988); (iii) total extinction was calculated by summing across the full size distribution; and (iv) f(RH)ext was determined by dividing the wet particle extinction by that calculated for dry conditions. These calculations included a number of simplifying assumptions, such as particle sphericity and full internal mixing.
Model calculations of f(RH)ext were used in two ways. First, κ was derived from experimental data using an optimization routine that minimized differences between experimental measurements and model predictions of f(RH)ext; this approach was applied to pure nigrosin. Second, f(RH)ext was calculated using a prescribed κ determined by volume weighting (Petters and Kreidenweis Citation2007) with subsequent comparison to measured data; this technique was applied to nigrosin–ammonium sulfate mixtures. Validation experiments using 200 nm diameter size-selected ammonium sulfate particles showed excellent agreement (within 2%) between measured f(RH)ext and predictions calculated using a literature value of κ = 0.53 (Petters and Kreidenweis Citation2007).
3.3. Model Calculations of Absorption at High RH
Two separate calculations were performed to model the expected change in absorption at high RH, both with and without the treatment of evaporation effects on the photoacoustic response. The first calculation assumed no evaporation bias and determined f(RH)abs_full in an identical manner to that outlined for f(RH)ext in Section 3.2. These calculations used aerosol hygroscopic growth information determined from f(RH)ext and assumed spherical particles and complete internal mixing of water and the dry aerosol components. Several studies have shown that the latter assumption introduces modest uncertainty for absorbing aerosol systems (Brem et al. Citation2012; Flores et al. Citation2012).
The second calculation considered evaporation effects on the PAS signal using the model framework of Murphy (Citation2009), which treated coupled heat and mass transfer in the transition regime for pure water droplets. The original publication should be referenced for full background information, including a detailed description of model assumptions. The key result pertinent to this work is that the ratio of the photoacoustic signal recorded under wet and dry conditions (S ratio) is given by
The theoretical absorption response including the effects of evaporation, f(RH)abs_bias, was determined as follows: (i) S ratio was calculated as a function of size at a series of elevated RH levels using humidified size distributions determined as described in Section 3.2; (ii) S ratio was weighted by particle number and integrated across the size distribution to yield an average value at each RH level; (iii) all S ratio values were divided by that calculated for the “dry” PAS measurement RH; and (iv) S ratio was multiplied by f(RH)abs_full to yield f(RH)abs_bias.
4. INSTRUMENT CHARACTERIZATION
4.1. PAS Microphone Sensitivity
The RH dependence of the microphone sensitivity was determined by performing multipoint ozone calibrations at different RH levels in synthetic air. The sensitivity was calculated by linearly fitting the background-corrected PAS signal (corrections up to and including step (iv) in Section 3.1) to the ozone absorption strength measured by CRDS. shows example calibration data collected at four different RH levels. The gradients derived from these data, together with those collected during six additional experiments, are shown in . To facilitate comparison, data were normalized to yield a sensitivity of 1 at high RH (85–89%). shows that there was a strong positive linear correlation between the microphone sensitivity and RH (factor of ∼1.25 between dry conditions and 90% RH). Failing to account for this strong dependence would clearly introduce significant errors to PAS measurements at high RH.
4.2. Validation of PAS Corrections
Experiments were performed to validate the performance of the correction procedures for high RH PAS operation outlined in Section 3.1. These tests used 300 nm diameter dyed PSL spheres atomized from pure distilled water. Dyed PSL spheres are not hygroscopic and therefore the optical behavior of this aerosol was not expected to be a function of RH. shows extinction measured during these experiments. Within experimental error, f(RH)ext = 1 from 10–87% RH, confirming that there was no significant water uptake by the dyed PSL. shows similar behavior for f(RH)abs, which provides a high degree of confidence that the large PAS corrections (10's% over this RH range) were applied accurately. This result gives a benchmark to which measurements on hygroscopic absorbing aerosol systems can be compared.
5. ABSORPTION MEASUREMENTS AT ELEVATED RH
5.1. Pure Nigrosin
Initial experiments focused on examining the water uptake properties of nigrosin, which have not been studied extensively (Flores et al. Citation2012). shows f(RH)ext measured for size-selected 200 nm diameter nigrosin particles. The data show continuous water uptake over the full 13–87% RH range studied. Model calculations (described in Section 3.2) retrieved κ nigrosin = 0.16, corresponding to diameter growth factors of 1.18 and 1.33 at 80% and 90% RH, respectively. These values are within 7% of those reported by Flores et al. (Citation2012).
FIG. 4 Extinction (a) and absorption (b–e) measurements for size-selected nigrosin as a function of RH. Unless otherwise stated, experiments were performed using 200 nm dry diameter particles at a temperature of 25°C. Model predictions shown for each experiment f(RH)abs_full (solid line) and f(RH)abs_bias (dotted lines) are described fully in the main text. Measurements of f(f(RH)abs shown in (b) are repeated in (c–e) to facilitate comparison (gray squares).
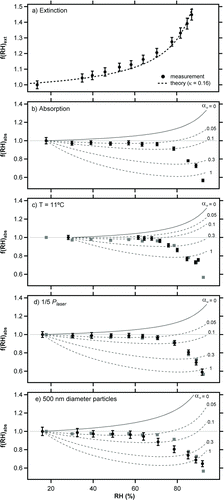
We performed measurements of f(RH)abs under a series of conditions pertinent to the evaluation of evaporation effects on the photoacoustic signal (). Unless noted otherwise, experiments were performed for 200 nm diameter nigrosin particles, a temperature of 25°C and a cw laser power of 150 mW. To facilitate comparison between datasets, observations for the base case (b) are also shown in the latter panels (gray squares). Each absorption dataset is accompanied by a series of theoretical predictions of f(RH)abs calculated as described in Section 3.3: f(RH)abs_full (solid line and equivalent to αM = 0) and f(RH)abs_bias (dotted lines evaluated for αM = 0.05, 0.1, 0.3, and 1).
All experimental data show a strong negative bias of absorption measured by the PAS at high RH. For measurements recorded at 25°C (b) the PAS signal was low biased by ∼0.57 at 90% RH, despite f(RH)abs_full predicting that absorption should have increased by ∼1.25. The RH dependence of the measured bias deviated strongly from that modeled by theory under the assumption of pure water droplets (f(RH)abs_bias) and a constant value of αM . At low RH (20–70%), where little water was present in the aerosol phase, observations were consistent with calculations performed using a relatively low value of αM = 0.05. At high RH (70–90%), where particles took up significant amounts of water, f(RH)abs was more strongly biased and could not be modeled using a single αM . Instead, model calculations suggest that αM increased in this RH range from ∼0.05 to 1. At the highest RH levels, the measured f(RH)abs was biased more strongly than the theoretical maximum predicted by model calculations using αM = 1. This discrepancy may be due to uncertainty in both the S ratio and f(RH)abs_full calculations. These results clearly show that the effective αM for water uptake to nigrosin varied as a function of RH. This dependence likely arose from the change in particle composition from nigrosin-rich (low αM ) to water-rich (high αM ) with increasing RH, which is discussed further in Section 6.
shows similar results for experiments conducted at a temperature of 11°C. This finding has important implications for the predictions of Murphy (Citation2009) that PAS biases could be reduced to workable levels by cooling samples to lower the absolute humidity. While this is indeed true at a fundamental level, the lack of sensitivity observed over the temperature range covered here indicates that operation well below 0°C would be necessary to lower the bias sufficiently. Achieving this experimentally would be difficult.
shows results for two additional experiments run with 1/5 incident laser power and larger 500 nm diameter particles, respectively. These experiments serve as further reference points for the universal observation of large, negative PAS biases at high RH. Of note, measurements recorded at 1/5 laser power were identical (within the uncertainty of the measurements) to those at full power, which was expected from theoretical considerations (Murphy Citation2009).
5.2. Nigrosin–Ammonium Sulfate Mixtures
A further series of experiments was conducted using internally mixed nigrosin–ammonium sulfate particles, which provided an absorbing aerosol with greater water uptake than pure nigrosin. The rationale for these experiments was to further explore the role of aerosol liquid water content on the observed PAS bias, which appeared to limit evaporation effects for pure nigrosin at low RH. Unless otherwise noted, experiments were performed using 200 nm dry diameter particles, a temperature of 25°C, and a solution concentration of 3.3:8.9 g L−1 nigrosin–ammonium sulfate (solution 1). A second solution concentration of 3.3:3.3 g L−1 nigrosin–ammonium sulfate was also tested (solution 2).
shows f(RH)ext measured for both solutions 1 and 2. Solution 1 showed stronger water uptake, in accordance with its higher ammonium sulfate mass fraction. Theoretical calculations were performed for each solution using prescribed κ values determined by volume weighting κ of the pure components (0.16 for nigrosin and 0.53 for ammonium sulfate). This approach yielded κ = 0.4 and 0.32 for solutions 1 and 2, respectively. Excellent agreement between modeled and measured f(RH)ext was observed.
FIG. 5 Extinction (a) and absorption (b–d) measurements for 200 nm dry diameter nigrosin–ammonium sulfate mixtures as a function of RH. Unless otherwise stated, experiments were performed at a temperature of 25°C using a solution concentration of 3.3:8.9 g L−1 nigrosin–ammonium sulfate (solution 1). A second solution concentration containing 3.3:3.3 g L−1 was also tested (solution 2). Model predictions shown for each experiment f(RH)abs_full (solid line) and f(RH)abs_bias (dotted lines) are described fully in the main text. Measurements of f(RH)abs in (b) are repeated in (c) and (d) to facilitate comparison (gray squares).
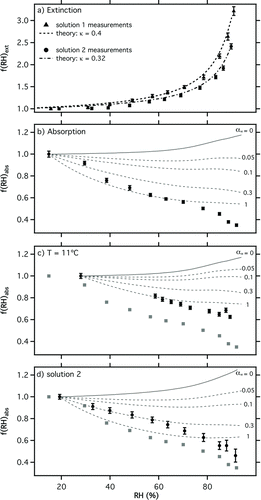
shows experimental measurements of f(RH)abs. As for pure nigrosin, all data were strongly low biased at high RH. However, a number of features clearly distinguished these results from those of pure nigrosin. First, the bias was stronger: at 25°C (b) it reached ∼0.35 at 90% RH compared to ∼0.57 for pure nigrosin. Second, the bias RH dependence was markedly different, with significantly stronger biases at low RH. The experimental f(RH)abs was not captured accurately by model calculations using a single value of αM , although model results indicated less variation in αM with RH than for pure nigrosin. In particular, αM was larger at low RH, which may have been due to the increased availability of water in the mixed solution particles and/or fundamentally faster water uptake kinetics for ammonium sulfate than nigrosin.
As expected, measurements for the less hygroscopic solution 2 (d) showed behavior in between that observed for pure nigrosin and solution 1. Measurements at low temperature (11°C, (c)) were less strongly biased than those at 25°C and were broadly consistent with the maximum theoretical bias modeled using αM = 1.
5.3. Ammonium Sulfate-Coated Dyed PSL
A final experiment was conducted using ammonium sulfate-coated dyed PSL spheres. This experiment had two purposes: to confirm that PAS signal biases were observed for particles with core-coat (as opposed to homogenous) morphologies, and to establish that previous results were not exclusive to nigrosin-based absorbing systems. As described in Section 2.1, experiments were performed by coating 600 nm diameter dyed PSL in ammonium sulfate to yield particles with a central mobility diameter of 690 nm. Given that the coating characteristics (e.g., thickness and uniformity) were poorly constrained, model calculations were not performed. This limitation did not however detract from the main purpose of these tests.
shows f(RH)ext and f(RH)abs measured for the coated dyed PSL aerosol. Extinction was enhanced significantly at high RH (by 1.7 at 88% RH) indicating strong water uptake by the coated particles. This result is markedly different from that observed for uncoated dyed PSL () which showed no enhanced extinction over a similar RH range. Aerosol absorption was strongly biased at high RH (0.45 at 89% RH), which also contrasted behavior observed for uncoated particles (). The bias increased approximately linearly with RH, sharing similarities with behavior observed for the mixed nigrosin–ammonium sulfate particles shown in Section 5.2. These results demonstrate that strong PAS biases at high RH are a universally observed phenomenon and are not specific to the aerosol systems studied in Sections 5.1 and 5.2.
6. DISCUSSION AND CONCLUSIONS
This work evaluated the performance of PAS for aerosol absorption measurements at high RH. PAS measurements on a range of absorbing aerosol, including different morphologies, hygroscopicities, and absorbing entities, all showed strong low biases at high RH due to the effects of water evaporation on the PAS signal. Importantly, the magnitude and RH dependence of the bias was not captured well using a model of PAS response incorporating coupled heat and mass transfer in the transition regime. The poor agreement with theory was attributed to the mass accommodation coefficient for water uptake changing as a function of RH. This dependence likely arose from the evolution of the particle surface composition from solute-rich to water-rich with increasing RH. Molecular dynamics simulations examining water uptake to liquid water surfaces coated by fatty acid monolayers have observed similar changes in αM as a function of surface state. For example, Takahama and Russell (Citation2011) showed an inverse linear relationship between αM and the water surface coverage by acid groups.
In principle, a correction scheme for the PAS bias could be developed if the functional RH dependence of αM was known. However, in practice this would require detailed knowledge of the particle surface state as a function of RH, which is not accessible. We performed additional calculations where αM was scaled by the aerosol water volume fraction, which was used as a proxy for the surface water coverage. This simplified approach assumed instantaneous, homogenous mixing of water following uptake and similar surface packing densities for water and the solute components. It failed to represent observations well. Our inability to model the PAS bias accurately on well-characterized aerosol systems demonstrates that it is currently not possible to use theory to apply adequate corrections to PAS measurements made at high RH.
The magnitude of the PAS bias measured at low temperature (11°C) was very similar to that measured at ambient temperature (25°C) for pure nigrosin particles, and was only slightly smaller for mixed nigrosin–ammonium sulfate particles. This indicated a low sensitivity to the predicted decrease in PAS bias magnitude with decreasing absolute humidity. While cooling may provide a route to reducing PAS biases, these results suggest that temperatures well below 0°C would be required, which would be difficult to achieve experimentally. Cooling particles to this extent could also introduce artifacts associated with condensation of volatiles and ultimately freezing.
Our results are consistent with the low-biased PAS absorption measurements reported by Arnott et al. (Citation2003) and Lewis et al. (Citation2009). This work extended those studies by examining the validity of the model framework used to represent the effects of evaporation. Our results are hard to reconcile with a reported field observation of strongly enhanced absorption by aged-mineral dust at high RH (factor 1.5 at 75% RH) (Lack et al. Citation2009). Possible reasons for the lack of bias observed by Lack et al. (Citation2009) could have been reduced sensitivity to biases due to the larger dust particle sizes (Murphy Citation2009), low αM for uptake of water onto dust, and/or incomplete application of the necessary PAS corrections for high RH operation (Section 3.1). Further measurements with laboratory-generated dust samples could provide useful further insight.
This work also has implications for the more common use of PAS to measure dry aerosol absorption. For normal operation, samples dried to below ∼35% RH would usually be considered “dry.” Under these conditions two key factors should be considered. First, the microphone sensitivity can vary significantly between 0 and 35% RH (by >1.1 in our system) and thus calibrations must be performed at the same RH used for aerosol measurements. Second, the magnitude of the PAS low bias can be significant at 35% RH for a hygroscopic absorbing aerosol (–). The extent of drying needed to render the bias insignificant is difficult to constrain on account of its dependence on particle properties. It may also not be advisable to dry samples too far, which could induce changes to particle phase and morphology that would impact absorption. Given these constraints, we recommend operation in the RH range 10–30% with the understanding that PAS biases may introduce a degree of irreducible uncertainty.
In summary, the body of work presented here suggests that the PAS technique is not well suited to the measurement of aerosol absorption at high RH. The technique may be useful for determining mass accommodation coefficients, which will be explored further in future work. Alternative techniques for the direct measurement of aerosol absorption using filter-based approaches (Schmid et al. Citation2006) and photothermal interferometry (Sedlacek and Lee Citation2007) are also known to perform poorly at high RH. Indirect methods that derive absorption from the difference in extinction and scattering at high RH have been applied successfully in laboratory settings using high loadings of absorbing aerosol (Brem et al. Citation2012), but these methods have not achieved the level of performance needed for field-based applications.
Acknowledgments
This work was supported by NOAA climate and air quality funding.
REFERENCES
- Arnott , W. P. , Moosmuller , H. , Sheridan , P. J. , Ogren , J. A. , Raspet , R. Slaton , W. V. 2003 . Photoacoustic and Filter-Based Ambient Aerosol Light Absorption Measurements: Instrument Comparisons and the Role of Relative Humidity . J. Geophys. Res. , 108 : 4034 – 4045 .
- Arnott , W. P. , Walker , J. W. , Moosmuller , H. , Elleman , R. A. , Jonsson , H. H. Buzorius , G. 2006 . Photoacoustic Insight for Aerosol Light Absorption Aloft from Meteorological Aircraft and Comparison with Particle Soot Absorption Photometer Measurements: DOE Southern Great Plains Climate Research Facility and the Coastal Stratocumulus Imposed Perturbation Experiments . J. Geophys. Res. , 111 D05S02
- Baynard , T. , Garland , R. M. , Ravishankara , A. R. , Tolbert , M. A. and Lovejoy , E. R. 2006 . Key Factors Influencing the Relative Humidity Dependence of Aerosol Light Scattering . Geophys. Res. Lett. , 33 : L06813
- Bohren , C. F. and Huffman , D. R. 1983 . Absorption and Scattering of Light by Small Particles , NJ : John Wiley, Hoboken .
- Bond , T. C. , Habib , G. and Bergstrom , R. W. 2006 . Limitations in the Enhancement of Visible Light Absorption due to Mixing State . J. Geophys. Res. , 111 D20211
- Brem , B. T. , Gonzalez , F. C. M. , Meyers , S. R. , Bond , T. C. and Rood , M. J. 2012 . Laboratory-Measured Optical Properties of Inorganic and Organic Aerosols at Relative Humidities up to 95% . Aerosol Sci. Technol. , 46 : 178 – 190 .
- Carrico , C. M. , Kus , P. , Rood , M. J. , Quinn , P. K. and Bates , T. S. 2003 . Mixtures of Pollution, Dust, Sea Salt, and Volcanic Aerosol During ACE-Asia: Radiative Properties as a Function of Relative Humidity . J. Geophys. Res. , 108 : 8650 – 8668 .
- Chylek , P. , Srivastava , V. , Pinnick , R. G. and Wang , R. T. 1988 . Scattering of Electromagnetic-Waves by Composite Spherical-Particles - Experiment and Effective Medium Approximations . Appl. Opt. , 27 : 2396 – 2404 .
- Covert , D. S. , Charlson , R. J. and Ahlquist , N. C. 1975 . A Study of the Relationship of Chemical Composition and Humidity to Light Scattering by Aerosols . J. Appl. Meteorol. , 11 : 968 – 976 .
- Flores , J. M. , Bar-Or , R. Z. , Bluvshtein , N. , Abo-Riziq , A. , Kostinski , A. Borrmann , S. 2012 . Absorbing Aerosols at High Relative Humidity: Linking Hygroscopic Growth to Optical Properties . Atmos. Chem. Phys. , 12 : 5511 – 5521 .
- Ghan , S. J. and Zaveri , R. A. 2007 . Parameterization of Optical Properties for Hydrated Internally Mixed Aerosol . J. Geophys. Res. , 112 : D10201
- Hao , L. Y. , Qiang , S. , Wu , G. R. , Qi , L. , Feng , D. Zhu , Q. S. 2002 . Cylindrical Mirror Multipass Lissajous System for Laser Photoacoustic Spectroscopy . Rev. Sci. Instrum. , 73 : 2079 – 2085 .
- IPCC . 2007 . Climate Change 2007: The Physical Science Basis: Contribution to the Fourth Assessment Report of the IPCC , Vol. 996 , Cambridge : Cambridge University Press .
- Jacobson , M. Z. 2001 . Strong Radiative Heating due to the Mixing State of Black Carbon in Atmospheric Aerosols . Nature , 409 : 695 – 697 .
- Jeong , M. J. , Li , Z. Q. , Andrews , E. and Tsay , S. C. 2007 . Effect of Aerosol Humidification on the Column Aerosol Optical Thickness over the Atmospheric Radiation Measurement Southern Great Plains Site . J. Geophys. Res. , 112 : D10202
- Khalizov , A. F. , Xue , H. X. , Wang , L. , Zheng , J. and Zhang , R. Y. 2009 . Enhanced Light Absorption and Scattering by Carbon Soot Aerosol Internally Mixed with Sulfuric Acid . J. Phys. Chem. A , 113 : 1066 – 1074 .
- Knutson , E. O. and Whitby , K. T. 1975 . Aerosol Classification by Electric Mobility: Apparatus, Theory and Applications . J. Aerosol Sci. , 6 : 443 – 451 .
- Kreidenweis , S. M. , Petters , M. D. and DeMott , P. J. 2008 . Single-Parameter Estimates of Aerosol Water Content . Environ. Res. Lett. , 3 doi: 10.1088/1748–9326/3/3/035002
- Lack , D. A. , Lovejoy , E. R. , Baynard , T. , Pettersson , A. and Ravishankara , A. R. 2006 . Aerosol Absorption Measurement Using Photoacoustic Spectroscopy: Sensitivity, Calibration, and Uncertainty Developments . Aerosol Sci. Technol. , 40 : 697 – 708 .
- Lack , D. A. , Quinn , P. K. , Massoli , P. , Bates , T. S. , Coffman , D. Covert , D. S. 2009 . Relative Humidity Dependence of Light Absorption by Mineral Dust after Long-Range Atmospheric Transport from the Sahara . Geophys. Res. Lett. , 36 : L24805
- Lack , D. A. , Richardson , M. S. , Law , D. , Langridge , J. M. , Cappa , C. D. McLaughlin , R. J. 2012 . Aircraft Instrument for Comprehensive Characterization of Aerosol Optical Properties, Part 2: Black and Brown Carbon Absorption and Absorption Enhancement Measured with Photo Acoustic Spectroscopy . Aerosol Sci. Technol. , 46 : 555 – 568 .
- Langridge , J. M. , Richardson , M. S. , Lack , D. , Law , D. and Murphy , D. M. 2011 . Aircraft Instrument for Comprehensive Characterization of Aerosol Optical Properties, Part I: Wavelength-Dependent Optical Extinction and its Relative Humidity Dependence Measured Using Cavity Ringdown Spectroscopy . Aerosol Sci. Technol. , 45 : 1305 – 1318 .
- Lewis , K. A. , Arnott , W. P. , Moosmuller , H. , Chakrabarty , R. K. , Carrico , C. M. Kreidenweis , S. M. 2009 . Reduction in Biomass Burning Aerosol Light Absorption Upon Humidification: Roles of Inorganically-Induced Hygroscopicity, Particle Collapse, and Photoacoustic Heat and Mass Transfer . Atmos. Chem. Phys. , 9 : 8949 – 8966 .
- Lide , D. R. 2001 . Handbook of Chemistry and Physics, , 82nd ed. , Boca Raton, FL, USA : CRC Press .
- Liu , B. Y. H. and Lee , K. W. 1975 . An Aerosol Generator of High Stability . Amer. Indust. Hygiene Assoc. , 36 : 861 – 865 .
- Malm , W. C. , Day , D. E. , Kreidenweis , S. M. , Collett , J. L. , Carrico , C. McMeeking , G. 2005 . Hygroscopic Properties of an Organic-Laden Aerosol . Atmos. Environ. , 39 : 4969 – 4982 .
- Massoli , P. , Murphy , D. M. , Lack , D. A. , Baynard , T. , Brock , C. A. and Lovejoy , E. R. 2009 . Uncertainty in Light Scattering Measurements by TSI Nephelometer: Results from Laboratory Studies and Implications for Ambient Measurements . Aerosol Sci. Technol. , 42 : 1064 – 1074 .
- Murphy , D. M. 2009 . The Effect of Water Evaporation on Photoacoustic Signals in Transition and Molecular Flow . Aerosol Sci. Technol. , 43 : 356 – 363 .
- O’Keefe , A. and Deacon , D. A. G. 1988 . Cavity Ring-Down Optical Spectrometer for Absorption-Measurements Using Pulsed Laser Sources . Rev. Sci. Instrum. , 59 : 2544 – 2551 .
- Petters , M. D. and Kreidenweis , S. M. 2007 . A Single Parameter Representation of Hygroscopic Growth and Cloud Condensation Nucleus Activity . Atmos. Chem. Phys. , 7 : 1961 – 1971 .
- Quinn , P. K. , Bates , T. S. , Baynard , T. , Clarke , A. D. , Onasch , T. B. Wang , W. 2005 . Impact of Particulate Organic Matter on the Relative Humidity Dependence of Light Scattering: A Simplified Parameterization . Geophys. Res. Lett. , 32 : L22809
- Ramanathan , V. and Carmichael , G. 2008 . Global and Regional Climate Changes due to Black Carbon . Nat. Geosci. , 1 : 221 – 227 .
- Raspet , R. , Slaton , W. V. , Arnott , W. P. and Moosmuller , H. 2003 . Evaporation-Condensation Effects on Resonant Photoacoustics of Volatile Aerosols . J. Atmos. Ocean Tech. , 20 : 685 – 695 .
- Redemann , J. , Russell , P. B. and Hamill , P. 2001 . Dependence of Aerosol Light Absorption and Single-Scattering Albedo on Ambient Relative Humidity for Sulfate Aerosols with Black Carbon Cores . J. Geophys. Res. , 106 : 27485 – 27495 .
- Rood , M. J. , Covert , D. S. and Larson , T. V. 1987 . Hygroscopic Properties of Atmospheric Aerosol in Riverside, California . Tellus , 39B : 383 – 397 .
- Schmid , O. , Artaxo , P. , Arnott , W. P. , Chand , D. , Gatti , L. V. Frank , G. P. 2006 . Spectral Light Absorption by Ambient Aerosols Influenced by Biomass Burning in the Amazon Basin. I: Comparison and Field calibration of absorption measurement techniques . Atmos. Chem. Phys. , 6 : 3443 – 3462 .
- Schwartz , S. E. 2004 . Uncertainty Requirements in Radiative Forcing of Climate Change . J. Air Waste Manage. Assoc. , 54 : 1351 – 1359 .
- Sedlacek , A. and Lee , J. 2007 . Photothermal Interferometric Aerosol Absorption Spectrometry . Aerosol Sci. Technol. , 41 : 1089 – 1101 .
- Silver , J. A. 2005 . Simple Dense-Pattern Optical Multipass Cells . Appl. Opt. , 44 : 6545 – 6556 .
- Sjogren , S. , Gysel , M. , Weingartner , E. , Baltensperger , U. , Cubison , M. J. Coe , H. 2007 . Hygroscopic Growth and Water Uptake Kinetics of Two-Phase Aerosol Particles Consisting of Ammonium Sulfate, Adipic and Humic Acid Mixtures . J. Aerosol Sci. , 38 : 157 – 171 .
- Stier , P. , Seinfeld , J. H. , Kinne , S. and Boucher , O. 2007 . Aerosol Absorption and Radiative Forcing . Atmos. Chem. Phys. , 7 : 5237 – 5261 .
- Takahama , S. and Russell , L. M. 2011 . A Molecular Dynamics Study of Water Mass Accommodation on Condensed Phase Water Coated by Fatty Acid Monolayers . J. Geophys. Res. , 116 : D02203
- Toon , O. B. , Pollack , J. B. and Khare , B. N. 1976 . Optical-Constants of Several Atmospheric Aerosol Species - Ammonium-Sulfate, Aluminum-Oxide, and Sodium-Chloride . J. Geophys. Res. , 81 : 5733 – 5748 .
- Wang , S. C. and Flagan , R. C. 1989 . Scanning Electrical Mobility Spectrometer. . J. Aerosol Sci. , 20 : 1485 – 1488 .
- Wiedensohler , A. 1988 . An Approximation of the Bipolar Charge-Distribution for Particles in the Sub-Micron Size Range . J. Aerosol Sci. , 19 : 387 – 389 .
- Winkler , P. M. , Vrtala , A. , Rudolf , R. , Wagner , P. E. , Riipinen , I. Vesala , T. 2006 . Condensation of Water Vapor: Experimental Determination of Mass and Thermal Accommodation Coefficients . J. Geophys. Res. , 111 : D19202