Abstract
This study presents a novel high-volume aerosol-into-liquid collector, developed to provide concentrated slurries of fine and/or ultrafine particulate matter (PM) to be used for unattended, in situ measurements of PM chemistry and toxicity. This system operates at 200 liters per minute (L/min) flow and utilizes the saturation–condensation, particle-to-droplet growth component of the versatile aerosol concentration enrichment system (VACES), growing fine or ultrafine PM to 3–4-μm droplets, in conjunction with a newly designed impactor, in which grown particles are collected gradually forming highly concentrated slurries. Laboratory evaluation results indicated an excellent overall system collection efficiency (over 90%) for both monodisperse and polydisperse particles in the range of 0.01 to 2 μm. Field evaluations illustrated that overall a very good agreement was obtained for most PM2.5 species between the new aerosol collection system and the VACES/BioSampler tandem as well as filter samplers operating in parallel. Very good agreement between the new system and the VACES/BioSampler was also observed for reactive oxygen species (ROS) in ambient PM2.5 samples, whereas lower ROS values were obtained from the water extracts of the filter, likely due to incomplete extraction of water insoluble redox active species collected on the filter substrate. Moreover, the field tests indicated that the new aerosol collection system could achieve continuous and unattended collection of concentrated suspensions for at least 2 to 3 days without any obvious shortcomings in its operation. Both laboratory and field evaluations of the high-volume aerosol-into-liquid collector suggest that this system is an effective technology for collection and characterization of ambient aerosols.
Copyright 2013 American Association for Aerosol Research
1. INTRODUCTION
Numerous epidemiological and toxicological studies have documented robust associations between ambient particulate matter (PM), particularly fine and ultrafine PM, and adverse health outcomes (Ritz et al. Citation2002; Pope and Dockery Citation2006). Recent studies have shown that elevated concentration of PM may be linked to cardiovascular diseases (Pope et al. Citation2004; Delfino et al. Citation2005, Delfino et al. Citation2011), pulmonary injury (Li et al. Citation2009), and lung cancer (Castranova et al. Citation2001; Garshick et al. Citation2004). Understanding sources, atmospheric transformations, and aging of ambient PM allows for more effective regulatory control strategies, more targeted air quality standards, and ultimately, reductions in population exposure to harmful types of airborne PM. However, the composition of ambient PM is very complex and highly dependent on local pollution sources and meteorological conditions, both of which may vary in short periods of time (Sardar et al. Citation2005; Cheung et al. Citation2011; Daher et al. Citation2013). Thus, ambient PM measurement in high time resolution becomes critical to identify the toxicologically relevant PM species, as well as the sources of these species, and remains an active topic of aerosol research.
Traditional PM sampling methodologies usually involve collection of particles onto substrates by filtration or inertial impaction. Since the quantitative composition measurements are usually performed offline, the collected particles are extracted using different types of solvents (e.g., water, methanol, etc.) and analyzed by various analytical techniques, such as ion chromatography (IC), inductively coupled plasma mass spectrometry (ICPMS), and total organic carbon (TOC) analysis. Although these sampling methodologies have been widely adopted to date, they are associated with a variety of intrinsic drawbacks, such as long turn-around time for processed results of chemical analysis, long sampling intervals for collection of sufficient mass for subsequent chemical analysis (hours to days, depending on sampling flow rate and ambient concentrations), incomplete extraction of insoluble PM-bound species, potential sampling artifacts (e.g., losses of labile species such as ammonium nitrate and semivolatile organic carbon [SVOC]) (Eatough et al. Citation2003; Schauer et al. Citation2003) and/or changes in chemical speciation (e.g., oxidation state shifts in redox active metals) due to prolonged sampling time (Turpin et al. Citation1994; Eatough et al. Citation2003). These drawbacks may significantly limit the feasibility and accuracy of conventional sampling approaches in investigating chemical composition and toxicological properties of ambient PM.
A number of advanced aerosol sampling technologies have been developed in recent years to overcome these limitations in conventional methodologies. In these newer technologies, ambient PM is directly collected as an aqueous suspension; for instance, PM collection onto a filter that is periodically washed by water (Buhr et al. Citation1995; Phan and McFarland Citation2004), impaction into a flowing liquid (Karlsson et al. Citation1997), collection by a water cyclone (Orsini et al. Citation2008), and the combination of particle growth by water vapor condensation and impaction on surfaces covered by liquid flow (Weber et al. Citation2001). These technologies effectively eliminate the need for elaborate extraction procedures and have been well documented in achieving more reliable measurements of chemical species such as organic carbon (Zhang et al. Citation2012) and secondary ions, including nitrate, sulfate, and ammonium (Takegawa et al. Citation2005). However, most of these technologies are likely inadequate for the measurement of trace level species such as metals and trace elements due to their low signal-to-noise ratio. As an alternative methodology, a novel system was developed that combines the versatile aerosol concentration enrichment system (VACES) (Kim et al. Citation2001a,Citationb) in tandem with a liquid impinger PM collector (BioSampler, SKC West, Inc., Fullerton, CA, USA) to effectively concentrate and collect both fine and ultrafine particles in an aqueous suspension. In VACES/BioSampler tandem, ambient PM is first grown to supermicrometer size droplets via condensational growth, and subsequently concentrated by virtual impactor (VI), and finally collected as a highly concentrated liquid suspension in the BioSampler, in a technique described in details by Kim et al. (Citation2001b). A similar system was also developed by Kidwell and Ondov (Citation2001). Daher et al. (Citation2011) compared sampling techniques of filtration, impaction, and VACES/BioSampler tandem, and an overall very good agreement in PM collection efficiency and chemical composition was observed among the different samplers. In contrast, dissimilarities in PM oxidative properties, measured by an ROS assay (Landreman et al. Citation2008), were apparent between the BioSampler slurry and aqueous extracts of the filter and impactor samples, with the former technology measuring considerably higher ROS levels, whereas filtering the VACES/BioSampler slurries brought their ROS content to virtually identical levels to those of the impactor and filter. The higher reactive oxygen species (ROS) values of the VACES/BioSampler were thus attributed to potentially toxic insoluble PM species, which cannot be effectively extracted from filter or impactor substrates with water. The study by Daher et al. demonstrated that both water-soluble and water-insoluble portions of ambient PM were effectively captured in VACE/BioSampler tandem, which is essential for toxicological measurements of PM redox properties, including their ROS content. Despite the robust PM collection performance of the VACES/BioSampler technology, several drawbacks may still exist that hinder its use for high-volume PM collection; these including excess water accumulation and clogging of the VI acceleration, and especially collection nozzles, clogging of BioSampler nozzles, and the limited collector volume (20 mL) of the conventional BioSampler, which requires periodic removal of the collected suspension samples. As a result, the operation of that system requires some field supervision, which limits its unattended operation in long periods of time.
The current study presents a novel sampling technology for collecting aerosol directly into liquid suspension using the saturation-condensation part of the VACES system. The new methodology replaces the VI in VACES with a low-pressure drop, high-flow rate impactor that can be operated for a prolonged periods of time, with no clogging issues observed due to its relatively large nozzle size (0.3-cm wide and 1.35-cm long). This system can be used for the collection of fine or ultrafine PM directly into an aqueous suspension for subsequent chemical analysis, as well as for collection of PM for in vitro health studies, hence effectively eliminating elaborate procedures of extracting collected particles from a filter substrate. Moreover, as it will be discussed in subsequent sections, collecting particles directly into an aqueous solution allows more efficient capturing of both water-soluble and water-insoluble components of ambient PM and offers better collection efficiency for semivolatile species, such as semivolatile organic compounds (SVOC) and ammonium nitrate.
2. METHODOLOGY
2.1. Description of the System and Its Components
The aerosol-into-liquid collector utilizes the saturation– condensation, particle-to-droplet growth system developed for the VACES (Kim et al. Citation2001a,Citationb) that grows sampled airborne particles to 3–4 μm droplets, which are subsequently collected in a low-pressure drop, high-flow rate (200 L/min) impactor. The sampled air is first drawn through a PM2.5 or ultrafine PM impactor (already developed for the VACES) (Kim et al. Citation2001b; Misra et al. Citation2002) before entering a saturator tank filled with ultrapure water produced by lab water purification system (Millipore A-10, EMD Millipore, Billerica, MA, USA) and maintained at 30°C, to saturate the air. The particle–vapor mixture then enters two condensation tubes that are connected to a circulating chiller maintained at about −3°C to promote condensational particle growth. The grown aerosol leaves the condensational tubes at roughly 21°C–22°C. The flow rate through each condensational tube is set at 100 L/min (Kim et al. Citation2001a, Citation2001b). The two air streams are then combined before entering the impactor to provide the 200-L/min operational flow rate. The schematic of the impactor is presented in . The impactor is made of chemically inert Teflon and consists of three stages: impaction stage, middle stage, and suspension collection stage. In the impaction stage, the droplet aerosol is accelerated through two slit impaction nozzles (0.3-cm wide and 1.35-cm long) and is impacted on the lateral cylindrical surfaces of the impactor, as shown in . The impacting droplets gradually drain into the collection stage, shaped like an inverse conical cavity, and form slurry samples at an approximate accumulation rate of 4–6 mL/h. The designed 50% cutpoint and pressure drop of the impactor are 1.5 μm and 0.02 atm (7 inches of water), respectively. In the middle stage, a microliquid level detector (ML 101, Cosense Inc., Hauppauge, NY, USA) is deployed for automatic measurement of the liquid sample volume, a metric which is essential for converting aqueous PM concentrations to airborne PM concentrations. The liquid level detector is an ultrasonic measurement system that performs high-speed, noncontact ultrasonic measurements with an accuracy of ±0.1 mm, and introduces no disturbance of the sample because there is no physical contact with the liquid. The volume of slurry collected is determined by the dimension of reservoir and the liquid level distance to the sensor. A suspension outlet port is installed at the bottom of the collection stage, thus automatic sample transport and removal can be achieved by using peristaltic pumps for continuous and unattended operation.
2.2. Laboratory Evaluation Tests of the Impactor's Collection Efficiency
The first part of the laboratory tests was to characterize the new impactor. Different sizes of monodisperse fluorescent polystyrene latex (PSL) particles (Polyscience Inc., Sacramento, CA, USA) with sizes of 1, 1.5, 3, and 6 μm in diameter were generated by a HOPE nebulizer (B&B Medical Technologies, Carlsbad, CA, USA) to determine the experimental 50% cutpoint of the impactor. A 37-mm Teflon filter (Teflon, 1-μm pore; Pall Corp., Life Sciences, Ann Arbor, MI, USA) was connected before and after the impactor to collect the generated PSL particles. At the end of each run, the Teflon filters from both upstream and downstream of the impactor were extracted with 10 mL of ethyl acetate to dissolve the hydrophobic fluorescent dye from the collected particles. The quantities of the fluorescent dye in the extraction solutions were quantified by a Fluorescence Detector (FD-500, GTI, Concord, MA, USA) to determine particle concentration.
The second part of the laboratory tests was to evaluate the collection performance of the aerosol-into-liquid collector using aerosols initially much smaller than the impactor's cutpoint. Fluorescent PSL particles in submicrometer size range (i.e., 42.5, 100, 300, 750, and 1000 nm) were generated as test aerosols by the nebulizer and were collected directly as suspensions in the impactor. Since these particles were smaller than the cutpoint of the impactor, they were first grown in the saturation–condensation module. A silica gel diffusion dryer (Model 3062, TSI Inc., Shoreview, MN, USA) was placed before the upstream and downstream Teflon filters to remove the excess water. In a similar manner to that outlined previously, the Teflon filters from both upstream and downstream of the impactor were extracted with 10 mL of ethyl acetate for sequential fluorescence measurement. Moreover, the residuals on the impactor inlet and impaction surfaces were carefully washed with ethyl acetate and extracted to estimate the inlet and wall losses.
During the tests with 300- and 750-nm PSL particles, a third condensation tube coupled with a VI followed by a BioSampler (i.e., the traditional VACES /BioSampler tandem configuration) was added to the system and operated in parallel. The major and minor flow rates of the VI were 100 and 5 L/min, respectively. Since PSL particles are mostly hydrophobic, suspension samples collected by BioSampler and the aerosol-into-liquid collector were dried first by evaporating the collected water using laboratory high-efficiency particulate air-filtered, purified compressed air. The remaining residuals were reextracted with 10 mL of ethyl acetate, and the extraction solutions were defined as “recovery” samples, representing the amount of incoming PM that is actually collected by either the aerosol-into-liquid collector or the VACES/BioSampler tandem. Recoveries from both BioSampler collection and impactor collection were then measured by means of fluorescence analysis as described above. The system configuration is presented in .
Lastly, polydisperse glutaric acid and ammonium nitrate particles were generated to simulate the “real-world” particles. Ammonium nitrate was chosen because it represents the predominant inorganic species in PM2.5 in Los Angeles (Malm et al. Citation2004), which, importantly, is also semivolatile in nature and thus poses significant challenges to traditional aerosol samplers (Mozurkewich Citation1993; Chang et al. Citation2000). Glutaric acid was selected because it is a typical organic dicarboxylic acid formed by ozone photooxidation (Cruz and Pandis Citation1999; Saleh et al. Citation2012). A small fraction (1 L/min) of the aerosols before and after the impactor were diverted first into a diffusion dryer (Model 3062, TSI Inc., Shoreview, MN, USA) and then passed through a DustTrak (Model 8520, TSI Inc., Shoreview, MN, USA) to measure mass concentrations and thus characterize the impactor's collection efficiency.
2.3. Field Tests of the Aerosol-into-Liquid Collector and Ambient PM Sample Collection
After completion of the laboratory evaluation, the system described in was deployed in the particle instrumentation unit (PIU) of the University of Southern California for field evaluation tests during January and February 2013. The site is located in an urban area near downtown Los Angeles, CA, within 150 m of a major freeway (I-110), and thus represents a typical urban mix of particles emitted by mostly traffic sources (Ning et al. Citation2007). The first part of the field evaluation was the continuity (or unattended sampling) tests. Ambient PM was continuously collected by the system for periods ranging between 48–72 consecutive hours. PM number concentrations were measured concurrently upstream of the impactor with an Aerosol Particle Sizer (APS 3320, TSI Inc., Shoreview, MN, USA), and with an Optical Particle Size (OPS 3330, TSI Inc., Shoreview, MN, USA) after the impactor. Suspensions collected in the impactor were transferred to collection vials (LDPE bottles; Thermal Scientific, Rockwood, TN, USA) by a peristaltic pump (Mityflex mode 907, Anko product Inc., Bradenton, FL, USA, using Tygon R-3603 PVC laboratory tubing) automatically every 4 h.
To assess the collection efficiency of the system for soot particles, ambient PM2.5 were collected by aerosol-into-liquid collector and black carbon (BC) concentrations before and after the impactor as well as in the ambient air were determined by means of a two-channel Aethalometer (Model AE-22, Magee Scientific, Berkeley, CA, USA). A silica gel diffusion dryer was placed before the Aethalometer to remove excess water and return the aerosols to ambient relative humidity levels.
Finally, ambient PM2.5 samples were collected concurrently using either the conventional filter or the VACES/BioSampler tandem as a reference, in parallel with the aerosol-into-liquid collector system, to evaluate comparability in terms of PM2.5 chemical composition. The system configuration for these experiments was similar to the previous description in laboratory evaluations, shown in . A diffusion dryer followed by a Teflon filter were placed immediately after the impactor and in parallel with the BioSampler () to estimate the PM mass loading in the BioSampler sample. The flow rate passing through the parallel Teflon filter was 1 L/min. For filter collection, the 5-L/min minor flow of the VI was directly connected to a diffusion dryer, followed by a 37-mm Teflon filter. All samples were kept frozen at −20°C after the sampling and prior to chemical analysis.
The PM2.5 samples (both slurries and filters) were subsequently analyzed for inorganic ions, total major and trace elements as well as TOC, water-soluble organic carbon (WSOC), and macrophage ROS activity. TOC/WSOC analysis was restricted to the BioSampler and aerosol-into-liquid collector, because TOC analysis is not possible on Teflon filters, and WSOC analysis was not performed on filter samples due to insufficient collected PM mass loading. The slurry samples were divided into two portions: one portion was ultrafiltered at 10 kilo-Dalton (kD) using centrifugal ultrafilters (Amicon Ultra-15 Centrifugal Filter Device, regenerated cellulose membrane in an all polypropylene device) and was used for the analysis of water-soluble PM components and the other portion was left unfractionated and represented the total mass of chemical species in the PM samples collected by the BioSampler or the aerosol-into-liquid collector. Subsamples of the unfractionated (total elements, TOC, and ROS) and ultrafiltered (inorganic ions, WSOC, and ROS) slurry samples were taken and distributed for chemical analysis. The slurry subsample for total elements was acid digested prior to elemental chemical analysis. Filter samples were sectioned in quarters to provide subsamples for both total and water soluble analyses that paralleled the slurry processing. One section was digested (microwave-aided acid digestion in Teflon bombs) for total elemental analysis. The other filter sections were extracted with water to provide water-soluble samples (ROS, inorganic ions). The inorganic ion composition was determined by IC. Measured ions included ammonium, nitrate, and sulfate. TOC/WSOC analyses were carried out using Sievers 900 Total Organic Carbon Analyzer (Stone et al. Citation2009). Measurements for major and trace elements were conducted using high-resolution magnetic sector ICPMS (SF-ICPMS, Thermo-Finnigan Element 2, Thermo Fisher Scientific Inc., Waltham, MA, USA) (Zhang et al. Citation2008). The macrophage ROS assay employed a fluorogenic cell-based method to investigate the production of ROS in rat alveolar macrophages (NR8383; American Type Culture Collection, Manassas, VA, USA) using 2, 7-dichlorodihydrofluorescein diacetate (DCFH-DA) as the fluorescent probe. Details of the assay, extraction protocol, and detection methodology are explained in previous study (Landreman et al. Citation2008). All the analysis mentioned above was also performed on lab blanks, and all the chemical/ROS speciation data presented in this manuscript are blank corrected.
3. RESULTS AND DISCUSSION
3.1. Laboratory Characterization of the Impactor
The impactor is designed with an operating flow rate of 200 L/min with the measured total pressure drop through the impactor of 0.02 atm (i.e., 7 inches of water). The 50% cutpoint (d50) of impactor is determined by the Stokes number of a particle having a 50% probability of capturing by impaction, Stk50, defined as follows for slit geometry impactors (Hinds Citation2002):
3.2. Laboratory Evaluation of the Collection Efficiency of the Aerosol-into-Liquid Collector
To evaluate the collection performance of the aerosol-into-liquid collector, PSL particles of 42.5, 100, 300, 750 nm, and 1 μm, all smaller than the impactor's cutpoint, were used as the test aerosols. The total collection efficiency is defined as the ratio of the downstream mass concentration to upstream concentration of the impactor, which was determined by measuring the fluorescent signal from corresponding filter extractions, following the methodology described earlier in this manuscript. Moreover, the inlet and wall losses were estimated by extracting the PSL residuals on inlet and impaction wall. The fraction collected in the suspension was determined by subtracting estimated inlet and impaction wall losses from total collection efficiency. Results from these tests are presented in , showing very good overall collection for both fine and ultrafine size particle ranges, with average total collection efficiency over 90%. The average inlet loss and wall loss of the impactor were quite low across the particle size range tested (i.e., 2.5% for inlet loss and 8.1% for wall loss).
TABLE 1 Collection efficiency of PSL particles and BC
During the tests with 300 and 750 nm PSL particles, a comparison in the collection efficiencies between the aerosol-into-liquid collector and VACES/BioSampler tandem was also performed to demonstrate equivalence between these two samplers. Results of these tests are presented in . Overall, a very good agreement in the recoveries of the BioSampler and the aerosol-into-liquid collector was observed (the average recovery was 86.6% for BioSampler and 82.2% for the aerosol-into-liquid collector). Despite the uncertainties due to the evaporation–extraction procedure discussed earlier, the recovery collection efficiencies of BioSampler and the aerosol-into-liquid collector show an excellent agreement with the estimated fraction collected in suspension considering inlet and wall losses from total collection efficiency (agreeing within 8% with the values reported in ).
TABLE 2 Particle recovery of BioSampler and aerosol-into-liquid collector
In addition to monodisperse PSL particles, similar collection efficiency tests were conducted with polydisperse glutaric acid and ammonium nitrate particles using a DustTrak for mass concentration measurements upstream and downstream of the impactor. shows the results of mass concentration measurements. Generally, the mass collection efficiencies of the impactor were above 95%, corroborating the collection efficiency results based on monodisperse submicrometer fluorescent aerosols discussed earlier.
TABLE 3 Collection efficiency of polydisperse particles
The collection efficiency of BC is also included in . Overall, the collection efficiency of the BC collection in the aerosol-into-liquid collector is 94.3 ± 4.0%, which is in excellent agreement with the collection efficiency reported for the PSL particles. Both the collection efficiency results for PSL particles and BC validate the ability of the aerosol-into-liquid collector to collect water-insoluble particles with near-ideal collection efficiencies.
FIG. 4 Continuity test results and particle size distribution. (a) Continuity test results (a few gaps in downstream measurement are due to temporary malfunction of the OPS monitor). (b) Typical ambient particle size distribution at sampling site during sampling period. (c) Droplet size distribution upstream and downstream of the impactor.
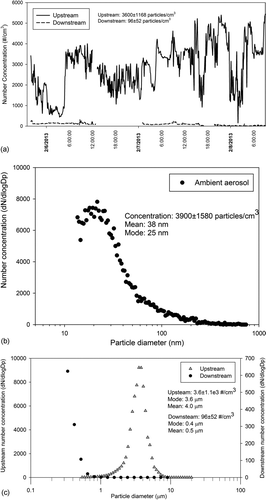
3.3. Field Evaluation of Continuous, Unattended Operation of the Aerosol-into-Liquid Collector
Field evaluation of the system was conducted after completion of lab evaluation tests. The system was deployed at PIU, and ambient PM was continuously sampled for about 72 h (shown in ) to validate the aerosol collector's ability to operate unattended for prolonged periods. The accumulation rate of the water from the aerosol droplets inside the impactor's collection cavity during the field tests varied between 4 to 8 mL per hour, depending on ambient temperature and relative humidity, as well as the ambient particle number concentrations. As shown in , the overall collection efficiency of the system was consistently above 90% (i.e., the average upstream number concentration was about on average 3600 ± 1168 particles/cm3, whereas the downstream number concentration was 96 ± 52 particles/cm3, indicating an overall collection efficiency of 96.8%), which confirms the ability of the system to operate unattended and for at least 2–3 days. Typical ambient particle size distribution () during the sampling period, as well as the droplet size distribution upstream and downstream of the impactor (), demonstrates that over 95% of the ambient particles (which are mostly smaller than the cutpoint of the impactor) are effectively grown through saturation–condensation (note the very close agreement in number concentration between ambient PM and grown droplets upstream of the impactor) and subsequently collected in the impactor.
3.4. Chemical Results for PM2.5 Samples
3.4.1. Inorganic Ions
presents the comparison between the filter, BioSampler and the aerosol-into-liquid collector samples for the three major inorganic ions in PM2.5: nitrate, ammonium, and sulfate. Error bars represent the standard deviation of multiple samples. Overall, agreement between filter, BioSampler, and the aerosol-into-liquid collector was obtained, considering the experimental uncertainties, with the exception of sulfate and nitrate levels measured on the filters, which were lower than those of the aerosol-into-liquid collector by about 35%–45%. It should be noted that the sulfate concentrations in the samples were generally very low and close to the IC detection limit, which increases the measurement uncertainty. The samples were also analyzed by SF-ICPMS, which has a much lower detection limit compared with IC, and considering that sulfate is the dominant species of soluble sulfur in ambient aerosols, the sulfate comparison after conversion of measured sulfur to sulfate yields excellent agreement ().
FIG. 5 Inorganic ions (nitrate, ammonium, and sulfate) comparison between filter, BioSampler, and impactor: (a) between BioSampler and aerosol-into-liquid collector. The p value from t test for nitrate, ammonium, and sulfate is 0.35, 0.85, and 0.8, respectively, and (b) between filter and aerosol-into-liquid collector. The p value from t test for nitrate, ammonium, and sulfate is 0.89, 0.97, and 0.31, respectively. Error bars represent the standard deviation of multiple samples. The sulfate (SF-ICPMS) results in a represent the estimated sulfate concentrations based on the sulfur (S) measurement results by SF-ICPMS analysis.
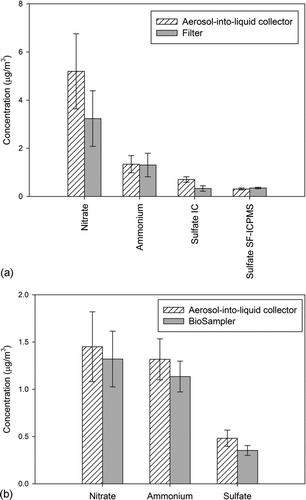
Previous studies have demonstrated sampling artifacts for labile semivolatile species, such as ammonium nitrate and SVOC, in traditional filter sampling methodologies (Turpin et al. Citation1994; Eatough et al. Citation2003). These artifacts are primarily introduced by volatilization losses of these labile species on filters during prolonged sampling periods. It has also been documented that preconcentration of PM and/or collection of PM directly into liquid (such as our case) may significantly reduce such artifacts for particulate nitrate (Chang et al. Citation2000; Khlystov et al. Citation2005). Our observations are generally consistent with these findings, given that volatilization loss of nitrate is probably reduced, if not eliminated, by collecting PM directly into a suspension, manifested by the near-excellent agreement between the nitrate levels measured in the aerosol-into-liquid collector and the BioSampler suspensions. We also acknowledge that condensation of gas-phase nitric acid and/or ammonia onto the sampled particles might occur during the saturation–condensation process. During the evaluation of the VACES, which uses the same components to the sampler described in this article to achieve supersaturation, Khlystov et al. (Citation2005) reported a positive artifact of 2.5%–7.5% from gas-phase condensation, which was more pronounced in ammonia-limited conditions, whereas in ammonia-rich environment these artifacts were negligible. Given that the samples in this study were collected in Los Angeles under ammonia-rich conditions (Kim et al. 2001b; Geller et al. Citation2002; Misra et al. Citation2004), the formation of extra material in the system is expected to be insignificant.
3.4.2. Total (TOC) and Water-Soluble Organic Carbon (WSOC)
To investigate the equivalence in their organic carbon concentrations, suspensions collected by BioSampler and aerosol-into-liquid collector were analyzed for both TOC and WSOC. As mentioned in previous section, TOC analysis cannot be done on Teflon filter samples and WSOC analysis was not performed on filter samples due to insufficient mass loadings. Results for this analysis are presented in . Error bars represent the standard deviation of multiple samples. Both TOC and WSOC in samples collected by VACES/BioSampler tandem were very close to those collected by the aerosol-into-liquid collector, considering the experimental uncertainties. The average TOC concentration is 5.6 ± 0.8 μg/m3 for BioSampler and 7.1 ± 1.7 μg/m3 for aerosol-into-liquid collector, whereas 5.1 ± 0.9 μg/m3 for BioSampler and 6.2 ± 1.8 μg/m3 for aerosol-into-liquid collector for WSOC measurements. The slightly higher (roughly 15%) TOC and WSOC levels measured by the aerosol-into-liquid collector could be due to its slightly smaller 50% cutpoint (1.4 μm in aerodynamic diameter) of the impactor compared with that of the BioSampler (2-μm aerodynamic diameter at 5 L/min (Kim et al. Citation2001b). The droplet size distribution of the grown ambient aerosols, shown in , reveals that there is approximately 10%–15% of the total droplet mass between 1.4 and 2 μm, which is consistent with the slightly higher concentrations of the aerosol-into-liquid collector. This may also explain the slightly higher sulfate and nitrate levels of the aerosol-into-liquid collector compared with the BioSampler, discussed in the previous section. Nonetheless these differences are not statistically significant as indicated by the p values from the t tests; with a p value of 0.66 and 0.73 for TOC and WSOC, respectively.
FIG. 6 TOC and WSOC comparison between BioSampler and aerosol-into-liquid collector. The p value from t test for TOC and WSOC is 0.66 and 0.73, respectively. Error bars represent the standard deviation of multiple samples.
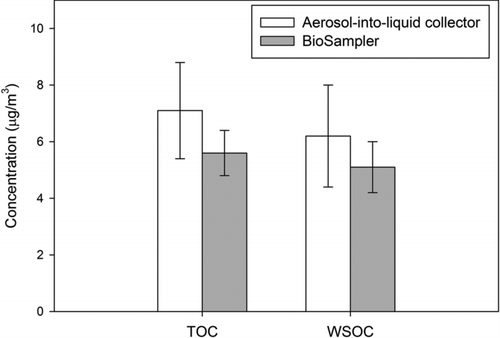
As mentioned in a previous section, we also acknowledge the formation of certain secondary organic products by reactions after water condenses to form cloud droplets, as well as the positive artifacts due to condensation of gas-phase semivolatile organic species may occur during sample collection. Based on our own field experiments, reported in the online supplemental information, condensation of gas-phase organics may be at most in the 1.5–2 μg/m3 range, so the reported particle–phase levels may be overestimated by about 20%.
3.4.3. Metals and Trace Elements
illustrates the comparison for total metals and trace elements between filter, BioSampler, and the aerosol-into-liquid collector samples. Error bars represent the standard deviation of multiple samples. Overall agreement for most metal species is observed between samples collected by the BioSampler and the aerosol-into-liquid collector, with correlation slope close to 1 and with high R 2 value (R 2 > 0.9). The average concentration ratio of the aerosol-into-liquid collector versus BioSampler across all measured trace elements and metals is 1.03 ± 0.10. This agreement is obtained over a large number of elements (a total of 24) covering a concentration range that span 5 orders of magnitude. Regarding the filters versus aerosol-into-liquid collector comparison, very good overall agreement is obtained for most total metals, with the average aerosol-into-liquid collector versus filter concentration ratio of 1.04 ± 0.14. Species are also well correlated with R 2 = 0.95. A few species were excluded from these correlations due to the fact that the measured concentrations were very close to the method detection limit (i.e., analytical, sampling, and handling) and the associated relative uncertainties were very high.
FIG. 7 Metals and trace elements comparison between filter, BioSampler, and impactor samples: (a) between filter and aerosol-into-liquid collector. The p value from t test is 0.71, and (b) between BioSampler and aerosol-into-liquid collector. The p value from t test is 0.94. Error bars represent the standard deviation of multiple samples. Inserted plot shows the correlations on log-scale. The line Y = X is also included for visibility purposes.
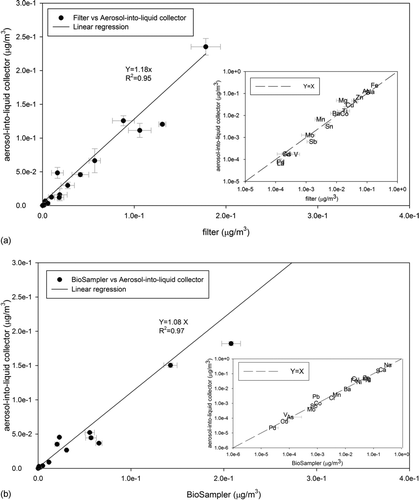
3.5. Macrophage ROS Activity
In addition to the chemical analysis discussed previously, a portion of PM2.5 samples collected by the filter, BioSampler, and the aerosol-into-liquid collector were analyzed for macrophage ROS activity. This cellular macrophage ROS assay provides an estimate of the overall oxidative potential of PM by measuring their ROS content (Verma et al. Citation2009; Shafer et al. Citation2010; Daher et al. Citation2012). represents the results from these comparisons. Error bars represent the standard deviation of multiple samples. The ROS levels are expressed on a per volume (of air) based activity metric in μg Zymosan units/m3 of air. As shown in , the ROS activity of suspension samples collected by aerosol-into-liquid collector is generally very close to that from BioSampler samples, for both unfiltered and filtered suspension samples (the latter representing the ROS activity presumably associated with water-soluble species), and within the experimental uncertainty.
FIG. 8 ROS activity comparison between filter, BioSampler, and aerosol-into-liquid collector: (a) between BioSampler and aerosol-into-liquid collector. The p value from t test for unfiltered and filtered slurry is 0.75 and 0.68, respectively, and (b) between filter extractions, filtered, and unfiltered aerosol-into-liquid collector slurries. The p value from t test between filter extraction and filtered slurry is 0.71. Error bars represent the standard deviation of multiple samples.
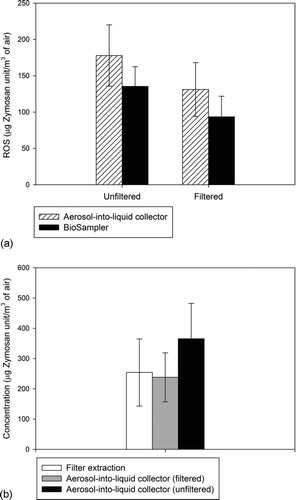
When comparing the ROS levels between filter and aerosol-into-liquid collector suspensions, however, we see that the ROS activity of the latter is higher on average by about 40% (i.e., average ROS activity on filter samples is 254 ± 110 μg Zymosan units/m3 of air while 365 ± 116 μg Zymosan units/m3 of air for unfiltered aerosol-into-liquid collector slurries). In a previous study, Daher et al. (Citation2011) demonstrated that the ROS activity of PM2.5 samples collected by the VACES/BioSampler was higher than that of parallel-sampling filters, but filtering the BioSampler slurry brought the ROS levels to almost identical values to the filter-based ROS. Similarly to the results presented in that study, filtering the aerosol-into-liquid collector slurries to remove insoluble PM species decreases the ROS activity to about the same levels as those of the filter extracts. These findings underscore the contribution of insoluble species to the overall PM2.5 redox activity. Given the rather small number of our samples, the observed difference between the ROS levels on slurries versus filters does not reach statistical significance, as we noted earlier. Nonetheless, the reported trends are consistent with the more rigorous data of Daher et al. (Citation2011) and illustrate the advantages of collecting PM directly into liquid suspension compared with conventional filter sampling, particularly for evaluating toxicological properties of PM such as redox activity. By eliminating extraction of PM from a filter substrate, sampling methodologies such as the aerosol-into-liquid collector, capture effectively both water-soluble and water-insoluble redox active PM components in suspension samples. Thus, this approach is likely to be more appropriate for collecting PM for in vitro and in vivo assays in the future.
4. SUMMARY AND CONCLUSION
This study describes the development, laboratory, and field evaluation of a new aerosol sampler operated in conjunction with a saturation–condensation system for growing the particles to supermicrometer sizes, capable of high volume collection of fine and ultrafine particles directly into aqueous suspensions. This sampling methodology was developed as an alternative to the VACES/BioSampler tandem for the collection of PM directly into liquid suspensions. The performance of the VACES/BioSampler tandem as a tool for collecting highly concentrated fine and ultrafine PM into liquid suspensions was previously demonstrated (Kim et al. Citation2001a; Khlystov et al. Citation2005). However, this system is not suitable for long-term sampling periods as it requires attendance by trained personnel. The aerosol-into-liquid collector improves this system by replacing the VI with a newly designed low-pressure drop impactor. This modification eliminates clogging of the BioSampler and VI nozzles as a result of excessive particle accumulation that comes with prolonged sampling periods, and therefore it makes it possible to achieve continuous and unattended collection of concentrated suspensions for at least 2 to 3 days. The collection performance of the system was evaluated in laboratory experiments using different types of particles, demonstrating excellent collection efficiency for hygroscopic and hydrophobic test aerosols.
The chemical composition of PM collected in the suspension with this sampler was quite similar to those obtained using the traditional filtration as well as the VACES/BioSampler system, for PM species including inorganic ions (except of nitrate), selected elements and metal species, and total and WSOC. Moreover, the field experiments indicated that volatile species such as nitrate are better preserved when the particles are collected directly into suspension compared with conventional filtration. Similar results were observed based on the ROS assays, demonstrating that this system can provide concentrated suspensions for use in in vitro studies that contain important water-soluble and water-insoluble redox active species, a unique advantage comparing with using filters to determine the oxidant properties of PM. Although there are some limitations, such as potential positive artifacts resulting from condensation of gas-phase semivolatile species onto the sampled particles, the new aerosol-into-liquid collector is highly adaptable and versatile for potential applications in long-term, in situ measurement of PM chemistry by overcoming the drawbacks of traditional sampling methods, both in terms of detection limit and time resolution.
Supplemental Information.zip
Download Zip (531.5 KB)Acknowledgments
The authors would like to thank Dr. Massoud Pirbazari and Woonhoe Kim (USC) for their assistance in the chemical analysis. This study has been supported by the South Coast Air Quality Management District (AQMD) through award number #11527 and the National Institutes of Health (NIH) through Grants R01AI065617-13 and R21AG040753-02 to the University of Southern California (USC). The research described herein has not been subjected to the agency's required peer and policy review and, therefore, does not necessarily reflect the views of the agency, and no official endorsement should be inferred. Mention of trade names or commercial products does not constitute an endorsement or recommendation for use.
[Supplementary materials are available for this article. Go to the publisher's online edition of Aerosol Science and Technology to view the free supplementary files.]
REFERENCES
- Buhr , S. M. , Buhr , M. P. , Fehsenfeld , F. C. , Holloway , J. S. , Karst , U. Norton , R. B. 1995 . Development of a Semicontinuous Method for the Measurement of Nitric-Acid Vapor and Particulate Nitrate and Sulfate . Atmos. Environ. , 29 : 2609 – 2624 .
- Castranova , V. , Ma , J. Y. C. , Yang , H. M. , Antonini , J. M. , Butterworth , L. Barger , M. W. 2001 . Effect of Exposure to Diesel Exhaust Particles on the Susceptibility of the Lung to Infection . Environ. Health Perspect. , 109 : 609 – 612 .
- Chang , M. C. , Sioutas , C. , Kim , S. , Gong , H. and Linn , W. S. 2000 . Reduction of Nitrate Losses from Filter and Impactor Samplers by Means of Concentration Enrichment . Atmos. Environ. , 34 : 85 – 98 .
- Cheung , K. , Daher , N. , Kam , W. , Shafer , M. M. , Ning , Z. Schauer , J. J. 2011 . Spatial and Temporal Variation of Chemical Composition and Mass Closure of Ambient Coarse Particulate Matter (PM10-2.5) in the Los Angeles Area . Atmos. Environ. , 45 : 2651 – 2662 .
- Cruz , C. N. and Pandis , S. N. 1999 . Condensation of Organic Vapors on an Externally Mixed Aerosol Population . Aerosol Sci. Technol. , 31 : 392 – 407 .
- Daher , N. , Hasheminassab , S. , Shafer , M. M. , Schauer , J. J. and Sioutas , C. 2013 . Seasonal and Spatial Variability in Chemical Composition and Mass Closure of Ambient Ultrafine Particles in the Megacity of Los Angeles . Environ. Sci. Proc. Impacts , 15 : 283 – 295 .
- Daher , N. , Ning , Z. , Cho , A. K. , Shafer , M. , Schauer , J. J. and Sioutas , C. 2011 . Comparison of the Chemical and Oxidative Characteristics of Particulate Matter (PM) Collected by Different Methods: Filters, Impactors, and BioSamplers . Aerosol Sci. Technol. , 45 : 1294 – 1304 .
- Daher , N. , Ruprecht , A. , Invernizzi , G. , De Marco , C. , Miller-Schulze , J. Heo , J. B. 2012 . Characterization, Sources and Redox Activity of Fine and Coarse Particulate Matter in Milan, Italy . Atmos. Environ. , 49 : 130 – 141 .
- Delfino , R. J. , Gillen , D. L. , Tjoa , T. , Staimer , N. , Polidori , A. Arhami , M. 2011 . Electrocardiographic ST-Segment Depression and Exposure to Traffic-Related Aerosols in Elderly Subjects with Coronary Artery Disease . Environ. Health Perspect. , 119 : 196 – 202 .
- Delfino , R. J. , Sioutas , C. and Malik , S. 2005 . Potential Role of Ultrafine Particles in Associations Between Airborne Particle Mass and Cardiovascular Health . Environ. Health Perspect. , 113 : 934 – 946 .
- Eatough , D. J. , Long , R. W. , Modey , W. K. and Eatough , N. L. 2003 . Semi-Volatile Secondary Organic Aerosol in Urban Atmospheres: Meeting a Measurement Challenge . Atmos. Environ. , 37 : 1277 – 1292 .
- Garshick , E. , Laden , F. , Hart , J. E. , Rosner , B. , Smith , T. J. Dockery , D. W. 2004 . Lung Cancer in Railroad Workers Exposed to Diesel Exhaust . Environ. Health Perspect. , 112 : 1539 – 1543 .
- Geller , M. D. , Kim , S. , Misra , C. , Sioutas , C. , Olson , B. A. and Marple , V. A. 2002 . A Methodology for Measuring Size-Dependent Chemical Composition of Ultrafine Particles . Aerosol Sci. Technol. , 36 : 748 – 762 .
- Hering , S. , Gundel , L. and Daisey , J. M. 1997 . A Microslot Impactor for Organic Aerosol Sampling . J. Aerosol Sci., , 28 : 1283 – 1290 .
- Hinds , W. C. 2002 . Aerosol Technology: Properties, Behavior, and Measurement of Airborne Particles , New York : Wiley-Interscience .
- Karlsson , A. , Irgum , K. and Hansson , H. C. 1997 . Single-Stage Flowing Liquid Film Impactor for Continuous On-Line Particle Analysis . J. Aerosol Sci. , 28 : 1539 – 1551 .
- Kidwell , C. B. and Ondov , J. M. 2001 . Development and Evaluation of a Prototype System for Collecting Sub-Hourly Ambient Aerosol for Chemical Analysis . Aerosol Sci. Technol. , 35 : 596 – 601 .
- Kim , S. , Jaques , P. A. , Chang , M. C. , Barone , T. , Xiong , C. Friedlander , S. K. 2001a . Versatile Aerosol Concentration Enrichment System (VACES) for Simultaneous in vivo and in vitro Evaluation of Toxic Effects of Ultrafine, Fine and Coarse Ambient Particles—Part II: Field Evaluation . J. Aerosol Sci. , 32 : 1299 – 1314 .
- Kim , S. , Jaques , P. A. , Chang , M. C. , Froines , J. R. and Sioutas , C. 2001b . Versatile Aerosol Concentration Enrichment System (VACES) for Simultaneous in vivo and in vitro Evaluation of Toxic Effects of Ultrafine, Fine and Coarse Ambient Particles—Part I: Development and Laboratory Characterization . J. Aerosol Sci. , 32 : 1281 – 1297 .
- Khlystov , A. , Zhang , Q. , Jimenez , J. L. , Stanier , C. , Pandis , S. N. Canagaratna , M. R. 2005 . In situ Concentration of Semi-Volatile Aerosol Using Water-Condensation Technology . J. Aerosol Sci. , 36 : 866 – 880 .
- Landreman , A. P. , Shafer , M. M. , Hemming , J. C. , Hannigan , M. P. and Schauer , J. J. 2008 . A Macrophage-Based Method for the Assessment of the Reactive Oxygen Species (ROS) Activity of Atmospheric Particulate Matter (PM) and Application to Routine (Daily-24 h) Aerosol Monitoring Studies . Aerosol Sci. Technol. , 42 : 946 – 957 .
- Li , N. , Wang , M. Y. , Bramble , L. A. , Schmitz , D. A. , Schauer , J. J. Sioutas , C. 2009 . The Adjuvant Effect of Ambient Particulate Matter Is Closely Reflected by the Particulate Oxidant Potential . Environ. Health Perspect. , 117 : 1116 – 1123 .
- Malm , W. C. , Schichtel , B. A. , Pitchford , M. L. , Ashbaugh , L. L. and Eldred , R. A. 2004 . Spatial and Monthly Trends in Speciated Fine Particle Concentration in the United States . J. Geophys. Res. Atmos. , : 109:D03306
- Marple , V. A. and Willeke , K. 1976 . Impactor Design. . Atmos. Environ. , 10 : 891 – 896 .
- Misra , C. , Fine , P. M. , Singh , M. and Sioutas , C. 2004 . Development and Evaluation of a Compact Facility for Exposing Humans to Concentrated Ambient Ultrafine Particles . Aerosol Sci. Technol. , 38 : 27 – 35 .
- Misra , C. , Kim , S. , Shen , S. and Sioutas , C. 2002 . A High Flow Rate, Very Low Pressure Drop Impactor for Inertial Separation of Ultrafine from Accumulation Mode Particles . J. Aerosol Sci. , 33 : 735 – 752 .
- Mozurkewich , M. 1993 . The Dissociation-Constant of Ammonium-Nitrate and Its Dependence on Temperature, Relative-Humidity and Particle-Size . Atmos. Environ. Part A-Gen. Topics , 27 : 261 – 270 .
- Ning , Z. , Geller , M. D. , Moore , K. F. , Sheesley , R. , Schauer , J. J. and Sioutas , C. 2007 . Daily Variation in Chemical Characteristics of Urban Ultrafine Aerosols and Inference of Their Sources . Environ. Sci. Technol. , 41 : 6000 – 6006 .
- Orsini , D. A. , Rhoads , K. , McElhoney , K. , Schick , E. , Koehler , D. and Hogrefe , O. 2008 . A Water Cyclone to Preserve Insoluble Aerosols in Liquid Flow—An Interface to Flow Cytometry to Detect Airborne Nucleic Acid . Aerosol Sci. Technol. , 42 : 343 – 356 .
- Phan , H. N. and McFarland , A. R. 2004 . Aerosol-to-Hydrosol Transfer Stages for Use in Bioaerosol Sampling . Aerosol Sci. Technol. , 38 : 300 – 310 .
- Pope , C. A. , Burnett , R. T. , Thurston , G. D. , Thun , M. J. , Calle , E. E. Krewski , D. 2004 . Cardiovascular Mortality and Long-Term Exposure to Particulate Air Pollution—Epidemiological Evidence of General Pathophysiological Pathways of Disease . Circulation , 109 : 71 – 77 .
- Pope , C. A. and Dockery , D. W. 2006 . Health Effects of Fine Particulate Air Pollution: Lines that Connect . J. Air Waste Manag. Assoc. , 56 : 709 – 742 .
- Ritz , B. , Yu , F. , Fruin , S. , Chapa , G. , Shaw , G. M. and Harris , J. A. 2002 . Ambient Air Pollution and Risk of Birth Defects in Southern California . Am. J. Epidemiol. , 155 : 17 – 25 .
- Saleh , R. , Khlystov , A. and Shihadeh , A. 2012 . Determination of Evaporation Coefficients of Ambient and Laboratory-Generated Semivolatile Organic Aerosols from Phase Equilibration Kinetics in a Thermodenuder . Aerosol Sci. Technol. , 46 : 22 – 30 .
- Sardar , S. B. , Fine , P. M. and Sioutas , C. 2005 . Seasonal and Spatial Variability of the Size-Resolved Chemical Composition of Particulate Matter (PM10) in the Los Angeles Basin . J. Geophys. Res. Atmos. , : 110:D07S08
- Schauer , C. , Niessner , R. and Poschl , U. 2003 . Polycyclic Aromatic Hydrocarbons in Urban Air Particulate Matter: Decadal and Seasonal Trends, Chemical Degradation, and Sampling Artifacts . Environ. Sci. Technol. , 37 : 2861 – 2868 .
- Shafer , M. M. , Perkins , D. A. , Antkiewicz , D. S. , Stone , E. A. , Quraishi , T. A. and Schauer , J. J. 2010 . Reactive Oxygen Species Activity and Chemical Speciation of Size-Fractionated Atmospheric Particulate Matter from Lahore, Pakistan: an Important Role for Transition Metals . J. Environ. Monit. , 12 : 704 – 715 .
- Stone , E. A. , Hedman , C. J. , Sheesley , R. J. , Shafer , M. M. and Schauer , J. J. 2009 . Investigating the Chemical Nature of Humic-Like Substances (HULIS) in North American Atmospheric Aerosols by Liquid Chromatography Tandem Mass Spectrometry . Atmos. Environ. , 43 : 4205 – 4213 .
- Takegawa , N. , Miyazaki , Y. , Kondo , Y. , Komazaki , Y. , Miyakawa , T. Jimenez , J. L. 2005 . Characterization of an Aerodyne Aerosol Mass Spectrometer (AMS): Intercomparison with other Aerosol Instruments . Aerosol Sci. Technol. , 39 : 760 – 770 .
- Turpin , B. J. , Huntzicker , J. J. and Hering , S. V. 1994 . Investigation of Organic Aerosol Sampling Artifacts in the Los-Angeles Basin . Atmos. Environ. , 28 : 3061 – 3071 .
- Verma , V. , Ning , Z. , Cho , A. K. , Schauer , J. J. , Shafer , M. M. and Sioutas , C. 2009 . Redox Activity of Urban Quasi-Ultrafine Particles from Primary and Secondary Sources . Atmos. Environ. , 43 : 6360 – 6368 .
- Weber , R. J. , Orsini , D. , Daun , Y. , Lee , Y. N. , Klotz , P. J. and Brechtel , F. 2001 . A Particle-into-Liquid Collector for Rapid Measurement of Aerosol Bulk Chemical Composition . Aerosol Sci. Technol. , 35 : 718 – 727 .
- Zhang , X. L. , Liu , J. M. , Parker , E. T. , Hayes , P. L. , Jimenez , J. L. de Gouw , J. A. 2012 . On the Gas-Particle Partitioning of Soluble Organic Aerosol in Two Urban Atmospheres with Contrasting Emissions: 1. Bulk Water-Soluble Organic Carbon . J. Geophys. Res.Atmos. , : 117:D00V16
- Zhang , Y. X. , Schauer , J. J. , Shafer , M. M. , Hannigan , M. P. and Dutton , S. J. 2008 . Source Apportionment of in vitro Reactive Oxygen Species Bioassay Activity from Atmospheric Particulate Matter . Environ. Sci. Technol. , 42 : 7502 – 7509 .