Abstract
The ability to produce nanoscale aerosols from dry powdered material is needed for studies of the toxicity and environmental transformation and fate of manufactured nanoparticles. Wet aerosol generation methods can alter particle chemistry, while dry methods often cannot produce truly nanoscale aerosols. We have developed a cost-effective dry dispersion technique for manufactured nanoparticles and have demonstrated its use with C60 fullerene, TiO2, and CeO2. The system disperses dry powders to create aerosols with mode diameters below 100 nm. Average mode and median diameters for each of the tested manufactured nanoparticles are 91 and 107 nm for C60, 65 and 77 nm for TiO2, and 40 and 43 nm for CeO2. All aerosols exhibit right-skewed unimodal distributions and irregular morphology. Aerosol mass concentrations produced by the dispersion system vary linearly with the mass of nanomaterial loaded into it and are of a magnitude appropriate for inhalation nanotoxicology studies. This work demonstrates the ability of a simple device to produce nanoscale aerosols from powdered engineered nanoparticles.
Copyright 2013 American Association for Aerosol Research
1. INTRODUCTION
As part of the rapid growth in nanotechnology, potential inhalation exposure to engineered nanoparticles (ENPs) has become a concern. ENPs have at least one, and often three dimensions that measure 100 nm or less. ENPs are produced in industrial or research facilities, while “incidental” nanoparticles may be similar to ENPs in composition but are the unintentional byproducts of human activities. In addition to engineered and incidental nanoparticles, “natural” nanoparticles are produced in the environment independent of human activities. There are likely to be substantial chemical and morphological differences between incidental and natural nanoparticles and ENPs (Win-Shwe and Fujimaki Citation2011).
Animal studies indicate that inhalation of ENPs leads to a variety of effects. Inhalation of gold nanoparticles by rats is associated with accumulation of gold nanoparticles in the lungs and kidneys (Sung et al. Citation2011), while inhalation of multiwalled carbon nanotubes (MWCNT) results in damage to DNA in rat lung cells (Kim et al. Citation2012). Iron oxide nanoparticle inhalation by rats is associated with enhanced levels of inflammation markers in blood (Srinivas et al. Citation2012) as well as elevated levels of reactive oxygen species (ROS) in the lungs (Sotiriou et al. Citation2012; Srinivas et al. Citation2012). The complex biological effects of inhaled manufactured nanoparticles are just beginning to be understood, however. Furthermore, nanotechnology as an economic sector is growing and is projected to exhibit an annual growth rate of 19% during 2011–2014 (RNCOS Citation2012). Together, these facts underscore the need for more toxicology research focused on inhaled ENPs. Such research is important from several perspectives, including consumer safety and worker safety.
ENPs are typically found as agglomerates in the environment. Agglomerates may exert different toxicological effects than their primary particle constituents. For example, rats exposed to sub-100 nm and super-100 nm agglomerates of 5 nm TiO2 ENPs via inhalation exhibit different markers of pulmonary toxicity, suggesting the agglomerate size, not the primary particle size, influenced the biological response (Noel et al. Citation2012). Because both the physical and chemical characteristics of inhaled particles can affect their toxicity, inhalation toxicology studies must take care to generate aerosols that represent fairly the substance of interest in a relevant exposure scenario. The choice of aerosol generation method, which can affect both of these characteristics, is an important one.
Aerosols may be generated through a variety of methods, both with and without the use of liquids. “Wet” aerosolization methods include pneumatic (nebulizing), electrospray, and ultrasonic techniques (Biskos et al. Citation2008), all of which create a spray from a liquid solution or suspension. Three types of aerosols can be produced: droplets from low-volatility liquids, or, upon drying, salts of a dissolved compound, or solids from a suspension. If the drying is only partial, then some solvent may remain on the aerosols. The concentration of the solution/ suspension and other process parameters can be adjusted to control characteristics of the resulting aerosol, such as size, morphology, and density (Vehring et al. Citation2007; Peltonen et al. Citation2010; Nandiyanto and Okuyama Citation2011). Because of this degree of control, high number concentrations and particle sizes well below 100 nm are achievable. These methods are well established, and numerous commercial devices employing them are available.
While wet methods can produce nanoscale particles with relative ease, there are drawbacks. Through interaction with impurities in the solvent or with the solvent itself, the particle surface may be chemically altered (Biskos et al. Citation2008; Masuda Citation2009). Sullivan et al. (Citation2010) found that the hygroscopicity of wet-generated CaCO3 aerosols exceeded that of dry-generated CaCO3 aerosols by a factor of 100 and that the hygroscopicity increased with both increasing time spent in water and with decreasing particle size. The authors noted that any changes to CaCO3 aerosols generated through wet methods had previously been assumed to be minimal, owing to the low solubility of CaCO3 in water. In addition to chemical effects, the choice of aerosolization method can also have physical and biological consequences. The viability of bacteria and fungi contained within grain dust aerosols (such as from wheat) was found to be influenced by the aerosol generation method (wet or dry) (Thorne Citation1994). Additionally, this difference in aerosol generation method also resulted in a 10-fold difference in particle size. Consequently, we should be hesitant to assume that aerosols produced via wet generation methods are chemically and physically equivalent substitutes for those produced via dry methods.
There are several techniques for generating aerosol that do not involve the use of liquids. A variety of techniques, including eductors, nozzles, impaction plates, and fluidized beds has been used to disperse a dry powder into a gas flow (Calvert et al. Citation2009). With any of these methods, though, creating aerosols smaller than 20 μm has been difficult (Calvert et al. Citation2009), and achieving an aerosol size below 1 μm has been especially challenging (Masuda Citation2009). As particle size decreases interparticle interactions, such as van der Waals and electrostatic forces, increase in relative importance (Calvert et al. Citation2013). Depending upon disperser design, forces supplied by the gas flow may not be able to overcome interparticle attraction, and the resulting agglomerates are mostly larger than 1 μm.
Although improved designs of commercial dry powder dispersers are enabling the production of significantly smaller particles, the generation of truly nanoscale aerosol remains elusive. A recent intercomparison of three commercial dispersers supplied with metal oxide NP powders demonstrated that while all produced submicron aerosols, most size distributions had mode diameters ranging from 200 nm to 400 nm, and none were below 100 nm (Tsai et al. Citation2012).
Several research groups have developed custom aerosolization devices, but in most cases the aerosols produced were not nanoscale. Tang et al. (Citation2008) used an inexpensive vacuum generator to disperse crystalline mannitol and amorphous bovine serum albumin, and produced aerosols with volume-weighted median diameters of 2.4 μm (mannitol) and 9.0 μm (BSA). These aerosols were only slightly larger than aerosols of the same materials generated by the Small Scale Powder Disperser (SSPD, TSI, Shoreview, MN, USA) and Scirocco dry powder disperser (Malvern, Worcs, UK), suggesting that at least in the cases of mannitol and BSA, such a system may prove an adequate substitute for costly commercial dispersers. To produce TiO2 agglomerates for a rat inhalation study, Noel et al. (Citation2012) coupled commercially available dispersion equipment with a custom-built venturi flow ejector; the median size of their aerosols was 185–194 nm. A custom-built system designed to aerosolize carbonaceous ENPs for inhalation toxicology studies produced single-walled carbon nanotube (SWCNT) aerosols over 1 μm in size (Madl et al. Citation2012), while a rodent inhalation study used a proprietary brush technique to generate multiwalled carbon nanotube (MWCNT) aerosols 0.7–2.0 μm in size as measured by cascade impaction (Ma-Hock et al. Citation2009).
To our knowledge, only two custom systems have produced nanoscale ENP aerosols. Schmoll et al. (Citation2009) used a speaker and a function generator to produce sub-100 nm aerosol from SiO2 and SWCNT. Size distributions for both particle types were bimodal, however, and the larger peak exceeded 100 nm. The system was not successful in aerosolizing TiO2. To et al. (Citation2009) described a rapid expansion of high pressure or supercritical suspensions (REHPS) system, which used high pressure and temperature to produce aerosols from titania and alumina ENPs with primary particle sizes of 21 and 13 nm, respectively. Aerosols generated by this system exhibited mode diameters of 40–80 nm (titania) and 70–90 nm (alumina). One possible disadvantage of the system is that the standard deviations of the size distributions were nearly as large as the mode diameter (70–80 for titania, 85–95 for alumina), indicating that the size distributions were quite wide (To et al. Citation2009). The REHPS system required high pressure (1.7–7.9 MPa) and temperature (45°C), which may be considered a drawback in some situations.
Producing sub-100 nm aerosol from ENPs via a dry dispersion process remains challenging. Most custom-designed systems are complex or labor-intensive, while often generating aerosols that are larger than can be considered nanoscale. Commercially available dispersers have not been demonstrated to generate nanoscale aerosols and can be expensive (∼$10,000). The objective of this work is to develop a cost-effective method to disperse bulk ENP powders into nanoscale aerosols. Based upon the work of Tang et al. (Citation2008), we use a venturi vacuum generator to disperse powdered manufactured nanoparticles by dispensing them into a high-velocity jet of air. We test the dispersion system using both carbonaceous (C60) and metal oxide (TiO2, CeO2) ENPs. The method shows promise for use in laboratory studies of both toxicity and environmental transformation and fate of ENPs.
2. EXPERIMENTAL
2.1. Disperser
The powder dispersion system is based on that of Tang et al. (Citation2008) and consists of a commercially available vacuum generating device (VCH10–018C, Pisco, Elmhurst, IL, USA), which costs less than $100, and standard tubing and fittings. Joining the T-junction of the vacuum generator to tubing with a standard threading system requires an adaptor to fit the vacuum generator's “R” threading. Other components are 0.635 cm o.d. tubing and compatible ball valves. A schematic and photograph of the setup are shown in .
The vacuum generator is positioned so that the vacuum arm points upward; this is used as the entry point for the ENP powder. The filter and metal cap provided with the vacuum generator are removed to reveal a nozzle, which acts as the aerosol outlet. Compressed air or other gas is provided to the opposite end of the disperser via 8-mm o.d. tubing.
The disperser system developed in this work differs from that of Tang et al. (Citation2008) in three ways: powder supply, aerosol destination, and pressure. First, the ENP powder is enclosed in a hopper for safety reasons, in contrast to the funnel used in Tang's work, which was open to ambient air. Second, the Tang system dispersed the aerosol directly into an aerosol sizing instrument (Mastersizer S laser diffractometer, Malvern, Worcs, UK), not into an environment at ambient pressure. Last, our system is operated at ∼150 kPa gauge pressure, in contrast to the higher (up to 620 kPa) pressures used in Tang's work.
In order to allow for safe handling practices while allowing for the dry dispersion of ENPs into an enclosed chamber, a nanomaterial hopper is attached to the vacuum arm of the disperser. A 10-cm length of stainless steel tubing (6.4 mm o.d.) serves as the hopper, which is bookended by brass ball valves. The hopper may be detached from the disperser below the lower valve to allow the user to load the ENPs into the hopper in a safe handling environment, such as an air curtain hood or other enclosure (Kuempel et al. Citation2012). Upon reassembly, the upper valve is opened to allow ambient air to flow into the disperser during operation. A filter may be attached to the upper valve as an additional safety measure. Compressed breathing air is then introduced to the disperser at a flow rate of ∼18 L min−1.
The powder release valve controls NP entry into the disperser. When the valve is opened, ENP powder falls into the air stream flowing through the disperser. Within the disperser, the powder undergoes rapid, forceful collisions (Tang et al. Citation2008), breaking it apart. Because aerosol generation happens rapidly (within seconds), the airflow may be stopped shortly after the powder is introduced.
2.2. Manufactured Nanoparticles
The disperser's ability to generate nanoscale aerosol was demonstrated with both carbonaceous and metal oxide ENPs: C60 (MER Corp., Tucson, AZ, USA), TiO2 (primary particle size 30–40 nm, NanoAmor, Houston, TX, USA), and CeO2 (primary particle size 15–30 nm, NanoAmor, Houston, TX, USA). Attempts to generate aerosols from MWCNT (NanoAmor) did not produce repeatable results. These ENPs were chosen because they facilitate comparison to other aerosolization techniques and they are currently used in toxicity studies.
As received from the manufacturer, C60 was in a crystalline form (with crystals >0.1 mm) and required milling in order to reduce the particle size prior to use. Milling was performed using a beadbeater with 2-mL polypropylene vials and 1-mm glass beads (mini-Beadbeater-1, vials and beads from BioSpec, Bartlesville, OK, USA). After milling, the beads, which had become coated with pulverized C60, were decanted into a larger glass vial and shaken vigorously. The beads were decanted a second time, and C60 powder clinging to the walls of the glass vial was scraped off using a stainless steel spatula. TiO2 and CeO2 were used as received. Aliquots of all ENPs were weighed in a capped 2-mL plastic vial before being loaded into the hopper.
2.3. Analytical Techniques
The ENP aerosols were dispersed directly into a ∼500-L flexible, polyethylene chamber (AtmosBag, Sigma Aldrich, St. Louis, MO, USA). Each ENP type was dispersed in 9–12 separate trials. Between trials, the bag was cleaned by evacuating it and refilling it with filtered breathing air; this cleaning process was repeated until the particle concentration in the bag was below 100 # cm−3. The compressed breathing air used to disperse the ENPs was confirmed not to introduce particles into the chamber.
The aerosol size distribution was measured using a Scanning Mobility Particle Sizer (SMPS, TSI, Shoreview, MN, USA) and an Aerodynamic Particle Sizer (APS, TSI). The size ranges measured by these instruments overlap slightly (SMPS: 14–750 nm; APS: 530 nm–20 μm) and can be merged into a single size distribution using the software DataMerge (TSI). The SMPS was equipped with the “long” differential mobility analyzer (DMA) and the 0.071 cm impactor, and it scanned the maximum size range (14–750 nm) over 5 min. Multiple charge correction was employed, and the CPC was set in low-flow mode. The size distribution measurement was initiated within 30 s of dispersion.
Density values entered into the SMPS and APS software were 0.6, 0.4, and 0.2 g cm−3 for C60, TiO2, CeO2, respectively. The density of 0.6 g cm−3 for C60 was measured for C60 powder resulting from the milling procedure described above, while the values for TiO2 and CeO2 are for the ENP powders, as reported by the manufacturer. While the density of the generated aerosols may differ from these values, the size distributions measured by the SMPS are independent of assumed particle density. The assumed densities influence the mass concentration estimates that are based on the size distributions. All particle size data shown in this work are from the merged distributions produced by DataMerge (TSI).
Particle morphology was examined using transmission electron microscopy (TEM, Philips EM420, 120 kV, LaB6 filament, spot size 2). Aerosol particles were deposited directly onto copper TEM grids using the third stage of an impactor with a cut size of 50 nm (MPS-3, California Measurements, Sierra Madre, CA, USA).
3. RESULTS
3.1. Size Distributions
shows typical size distributions of the three ENP aerosols. The smallest aerosols were obtained using CeO2, which had the smallest primary particle size, while the largest were of C60. All distributions were unimodal, right-skewed, and approximately lognormal. Particle size statistics are summarized in . All data are number-weighted and refer to the agglomerate, not primary, particle size. The geometric mean diameters differed from the median diameters by 3%, 14%, and 16% for C60, TiO2, and CeO2, respectively (in a perfectly lognormal distribution, these metrics would be identical). Mode diameters for aerosol particles of each ENP were approximately 90 nm, 65 nm, and 40 nm for C60, TiO2, and CeO2, respectively.
TABLE 1 Particle statistics for produced ENP aerosols
FIG. 2 Mean size distributions (error bars show standard deviation) of nanoparticle aerosols. Mean size distributions are normalized to the total particle count.
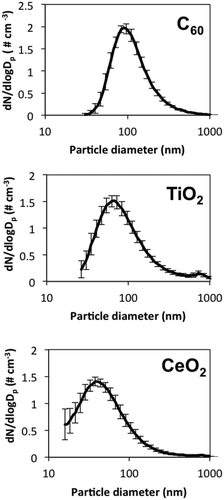
The mode diameter of the aerosols was not influenced by the mass of ENP powder loaded into the disperser. shows the mode diameter as a function of loaded ENP mass for each of the three substances. For all ENPs, the relationship between the two variables was not significant.
3.2. Aerosol Mass Concentration
shows the estimated aerosol mass concentration produced in the chamber as a function of ENP mass initially loaded into the disperser. For each type of ENP, increased loaded mass resulted in an increased aerosol mass concentration. The least-squares linear regression lines shown in are significant at the p < 0.005 level for all ENPs. For CeO2, the regression omits the five outlying points. The tendency of CeO2 to occasionally produce outlying aerosol mass concentrations in this system is discussed below.
3.3. Particle Morphology
The morphology of the resulting aerosol was examined using TEM. shows TEM images for each type of ENP aerosol. In both TiO2 and CeO2 aerosols, the primary particles could be seen easily, and their sizes agreed with the primary particle size range advertised by the manufacturer. Both TiO2 and CeO2 aerosols appeared tightly packed and of irregular rounded shape. The aerosols were larger than the mode diameter indicated by DataMerge; a potential reason for this discrepancy is discussed below. The C60 particles showed lattice fringes in a portion of the particle, which overall exhibited an irregular shape.
4. DISCUSSION
4.1. Particle Size and Size Distribution
This work has demonstrated a cost-effective method of producing nanoscale aerosols from powdered ENPs. Particle size distributions achieved by this system exhibit modes below 100 nm, smaller than produced by other dry dispersion systems described in the literature, both commercial and custom made, with the exception of the REHPS system described by To et al. (Citation2009). Particle statistics for the system described here and others from the literature are summarized in .
While the MWCNT aerosol produced by Ma-Hock et al. (Citation2009) appears to be sub-100 nm, as measured by the SMPS, those authors question the validity of both SMPS and optical methods to accurately size MWCNT aerosols due to their potential to form complex, tangled structures. This uncertainty is illustrated by the order-of-magnitude difference in aerosol size according to the two instruments used in the study. Titania and alumina ENP aerosols produced by the REHPS system exhibit median diameters from 40 to 90 nm, as shown in (To et al. Citation2009). However, that the REHPS system utilizes both elevated temperature (45°C CO2) and pressure (1.7–7.9 MPa), whereas the disperser described here operates at ambient temperature and significantly lower pressure (∼150 kPa). Additionally, the system described in this work has been demonstrated to produce nanoscale aerosols from ENPs of various compositions (carbonaceous, metal oxide), whereas the REHPS system has been demonstrated for metal oxide aerosols alone.
Aerosol size in this work is reported in terms of mobility diameter, dm . The accuracy of dm as a measure of an aerosol's true size has been questioned, especially for irregularly shaped aerosols, such as those composed of carbon nanotubes (Ku et al. Citation2007; Ma-Hock et al. Citation2009). While the aerosols in this work are not spherical, as is assumed when dm is calculated, the particle morphology is noticeably more densely packed than are carbon nanotube aerosols. Work by Shin et al. (Citation2009) with silver nanoparticle aerosols demonstrates that the “projected area diameter,” d pa, determined from TEM images is equal to or less than dm for aerosols in the size range of ∼80–250 nm. While d pa may exceed dm slightly for particles with dm <50 nm, overall dm should be a fairly accurate proxy for d pa for these aerosols, given their size and morphology. Aerosols smaller than 100 nm deposit with 20–70% efficiency in the alveolar region (Oberdorster et al. Citation2005; Asgharian and Price Citation2007); consequently, the aerosols in this work are relevant to inhalation toxicology studies in terms of size.
TABLE 2 Statistics of nanoparticle aerosols generated by various dry powder dispersion techniques. All values are number-weighted unless otherwise specified
In contrast to results from other dry dispersion techniques, the aerosol size distributions produced by this method are unimodal, approximately lognormal, and relatively narrow. Schmoll et al.'s ADAGE system (Citation2009) produces bimodal distributions for all ENPs tested. TSI's Small Scale Powder Disperser (SSPD) as tested by Tsai et al. (Citation2012) produces unimodal, but very broad, size distributions (with “peaks” that plateau over several hundred nanometers) for all three ENPs tested. The size distributions produced by the Palas RBG (rotating brush generator) and fluidized bed generator (FBG) utilized by Noel et al. (Citation2012) are also relatively broad, with interquartile (D25–D75) ranges of 108–284 nm and 81–470 nm, respectively. In comparison, interquartile ranges for the aerosols produced in this work, shown in , suggest narrower size distributions and are as follows: C60, 77–151 nm; TiO2, 51–124 nm; CeO2, 29–69 nm. The size distributions in this work also appear to be comparable in terms of relative width to those produced by the REHPS system, although a quantitative comparison is not possible. The size of the nozzle through which the ENPs are dispersed in each system (254 μm in To et al. [Citation2009] vs. 1 mm in this work) may affect the width of the size distribution. Tang et al. (Citation2008) found that narrower custom nozzles result in wider size distributions of BSA and mannitol aerosols, although the applicability of that relationship to ENPs has not been directly investigated.
4.2. Aerosol Number and Mass Concentration
This disperser can produce aerosol number concentrations that match or surpass those generated by other systems, as shown in . When dispersed into a ∼500 L chamber, the ENPs produced number concentrations of 6.82 × 104–1.40 × 106, 1.12 × 105–7.95 × 105, and 2.95 × 105–7.25 × 105 # cm−3 for C60, TiO2, and CeO2 respectively. Additionally, the number concentration can be controlled by changing the loaded ENP mass, chamber size, or both. In general, number concentrations higher than these are not desirable because coagulation would become important.
Using powder density values for all materials (0.6 g cm−3 for C60, 0.4 g cm−3 for TiO2, and 0.2 g cm−3 for CeO2), these number concentrations translate into mass concentrations () that are of similar order of magnitude to those used for inhalation nanotoxicology studies (Ma-Hock et al. Citation2009; Chen et al. Citation2012; Kim et al. Citation2012; Noel et al. Citation2012). The actual densities of aerosol particles, however, are likely to be higher than the bulk powder densities since the primary particles appear tightly packed within the aerosols, as discussed below. Since the density of the aerosol particles will likely lie between the density of the powder and the density of the nanoparticle material, the mass concentrations shown in are conservative.
Direct comparison of aerosol number concentrations obtained in this study and by Tsai et al. (Citation2012) with the SSPD is challenging, as the SSPD results are normalized to aerosol mass. This direct method of aerosol mass measurement removes uncertainty about the density of ENP aerosols but can prohibit simple comparison of number concentration. A rough comparison can be made, however, by using the SMPS mass concentration estimate of the TiO2 aerosols and bounds on the density of TiO2 powder. Using the powder and nanoparticle material densities of TiO2 ENP powder provided by the manufacturer (0.4 vs. 3.94 g cm−3), we estimate that the number concentrations, normalized to mass, of the TiO2 aerosols produced via our method range from 1.0 × 107 to 1.0 × 108 # cm−3 g−1. These concentrations exceed the normalized concentrations of TiO2 aerosol produced by the SSPD in Tsai's work (2.6 × 106 # cm−3 g−1). While a firm conclusion is precluded by the uncertainty in aerosol density and the indirect estimate of mass concentration provided by the SMPS, it is likely that the number concentrations of TiO2 aerosol produced in this system match, and possibly surpass, those produced by the SSPD as measured by Tsai et al.
The relationships between aerosol mass concentration and ENP mass loaded are statistically significant for all three materials, allowing the user to control the aerosol mass concentration produced. One exception is the group of outlying data points produced using CeO2. The texture of CeO2 is notably different from that of the other two tested ENPs; CeO2 appears fluffy and does not pour well during handling. This cohesive behavior may affect the way the powder flows into the gas stream in the disperser (Castellanos Citation2005). Cohesive powders pack into open structures (i.e., with more empty space between primary particles) that flow less well than their less cohesive, more densely packed counterparts (Castellanos Citation2005; Ghoroi et al. Citation2013). Comparing the bulk and true densities of the metal oxide ENPs provided by the manufacturer (0.4 vs. 3.94 g cm−3 for TiO2, <0.2 vs. 7.132 g cm−3 for CeO2), it appears that CeO2 packs much less densely than does TiO2, and thus would flow poorly by comparison. This cohesivity may allow for less reproducible behavior within the disperser and result in occasional outlying mass concentrations. Both the 20-fold difference between bulk and true densities of MWCNT (∼0.1 vs. 2.1 g cm−3, #1228NMG, NanoAmor, Houston, TX, USA), and the fibrous nature of MWCNT that allows them to become irregularly intertwined with one another, suggest that cohesiveness of MWCNT may also be a hurdle to their successful nanoscale dispersion.
4.3. Morphology, Crystallinity
TEM examination of the resulting ENP aerosols shows particles of irregular shape, as one might expect following a chaotic dry dispersion process. The TEM images, especially for TiO2 and CeO2, show aerosols larger than the mode diameter indicated by the SMPS. This is likely due to the use of impaction, which favors larger aerosols, as the mechanism for collecting samples onto TEM grids. An alternative sampling mechanism, thermophoretic precipitation, may result in a more representative sample of nanoscale aerosols since aerosols of diameter ∼100 nm and less deposit with equal efficiency under thermophoresis (Strom and Sasic Citation2012).
While the metal oxide ENPs have primary particle sizes from 10 to 40 nm, single C60 molecules are less than 1 nm across; the high-purity C60 molecules used in this study are packed into a crystal structure. The C60 crystals are milled as described above to reduce their size, and agglomerates of the crystalline C60 are broken up during dispersion. Consequently, the C60 aerosols result from a top-down size reduction process. The visible lattice fringes in show that the crystallinity of C60 remains intact through both milling and dispersion processes. This fundamental difference between the C60 and the metal oxides (crystalline molecules vs. primary particles) aligns well with the observation that the C60 aerosols were the largest of the three. Breaking down crystalline C60 even further—so the aerosols exhibited the same mode diameters as did the metal oxide aerosols—would require even stronger forces during milling and dispersion. By comparison, the formation of the metal oxide aerosols likely consists of both top-down and, possibly to a lesser extent, bottom-up processes, where as-received ENP agglomerates in powder form are broken up into both smaller agglomerates and possibly also into primary particles; these primary particles could reagglomerate.
4.4. Future Work
One common feature of commercial dry dispersion equipment is the ability to produce a constant flow of aerosols over several minutes or more. If a continuous aerosol source is required, as in many toxicity studies, this method could be used to disperse aerosols into an upstream mixing chamber, from which a continuous stream of aerosols could be drawn. The disperser system could be converted into a continuous output aerosol generator through the addition of a feed mechanism to control the introduction of ENP powder into the disperser. Further work is required in order to determine if this method can successfully be adapted into an aerosol generation system that is capable of generating aerosols (a) continuously, (b) at target concentrations, and (c) smaller than 100 nm in size, as verified by several analytical methods, including TEM.
5. CONCLUSIONS
This work describes the development of a dry dispersion technique capable of producing sub-100 nm aerosols from manufactured nanoparticles cost-effectively at ambient temperature and an operating pressure near 150 kPa. The mass concentration of aerosols produced varies linearly with the mass of manufactured nanoparticles loaded into the system, and for the three tested materials, the dispersed aerosols exhibit a unimodal distribution and irregular morphology. For these three materials (C60, TiO2, and CeO2), this system is capable of producing manufactured nanomaterial aerosols with smaller mode and geometric mean diameters than is commercially available dry powder dispersion equipment.
REFERENCES
- Asgharian , B. and Price , O. T. 2007 . Deposition of Ultrafine (NANO) Particles in the Human Lung . Inhal. Toxicol. , 19 ( 13 ) : 1045 – 1054 .
- Biskos , G. , Vons , V. , Yurteri , C. U. and Schmidt-Ott , A. 2008 . Generation and Sizing of Particles for Aerosol-Based Nanotechnology . Kona Powder Part. J. , 26 : 13 – 35 .
- Calvert , G. , Ghadiri , M. and Tweedie , R. 2009 . Aerodynamic Dispersion of Cohesive Powders: A Review of Understanding and Technology . Adv. Powder Technol. , 20 ( 1 ) : 4 – 16 .
- Calvert , G. , Hassanpour , A. and Ghadiri , M. 2013 . Analysis of Aerodynamic Dispersion of Cohesive Clusters . Chem. Eng. Sci. , 86 : 146 – 150 .
- Castellanos , A. 2005 . The Relationship Between Attractive Interparticle Forces and Bulk Behaviour in Dry and Uncharged Fine Powders . Adv. Phys. , 54 ( 4 ) : 263 – 376 .
- Chen , B. T. , Schwegler-Berry , D. , McKinney , W. , Stone , S. , Cumpston , J. L. Friend , S. 2012 . Multi-Walled Carbon Nanotubes: Sampling Criteria and Aerosol Characterization . Inhal. Toxicol. , 24 ( 12 ) : 798 – 820 .
- Ghoroi , C. , Han , X. , To , D. , Jallo , L. , Gurumurthy , L. and Dave , R. N. 2013 . Dispersion of Fine and Ultrafine Powders Through Surface Modification and Rapid Expansion . Chem. Eng. Sci. , 85 : 11 – 24 .
- Kim , J. S. , Sung , J. H. , Song , K. S. , Lee , J. H. , Kim , S. M. Lee , G. H. 2012 . Persistent DNA Damage Measured by Comet Assay of Sprague Dawley Rat Lung Cells After Five Days of Inhalation Exposure and 1 Month Post-Exposure to Dispersed Multi-Wall Carbon Nanotubes (MWCNTs) Generated by New MWCNT Aerosol Generation System . Toxicol. Sci. , 128 ( 2 ) : 439 – 448 .
- Ku , B. K. , Maynard , A. D. , Baron , P. A. and Deye , G. J. 2007 . Observation and Measurement of Anomalous Responses in a Differential Mobility Analyzer Caused by Ultrafine Fibrous Carbon Aerosols . J. Electrostat. , 65 ( 8 ) : 542 – 548 .
- Kuempel , E. D. , Geraci , C. L. and Schulte , P. A. 2012 . Risk Assessment and Risk Management of Nanomaterials in the Workplace: Translating Research to Practice . Ann. Occup. Hyg. , 56 ( 5 ) : 491 – 505 .
- Ma-Hock , L. , Treumann , S. , Strauss , V. , Brill , S. , Luizi , F. Mertler , M. 2009 . Inhalation Toxicity of Multiwall Carbon Nanotubes in Rats Exposed for 3 Months . Toxicol. Sci. , 112 ( 2 ) : 468 – 481 .
- Madl , A. K. , Teague , S. V. , Qu , Y. Q. , Masiel , D. , Evans , J. E. Guo , T. 2012 . Aerosolization System for Experimental Inhalation Studies of Carbon-Based Nanomaterials . Aerosol Sci. Technol. , 46 ( 1 ) : 94 – 107 .
- Masuda , H. 2009 . Dry Dispersion of Fine Particles in Gaseous Phase . Adv. Powder Technol. , 20 ( 2 ) : 113 – 122 .
- Nandiyanto , A. B. D. and Okuyama , K. 2011 . Progress in Developing Spray-Drying Methods for the Production of Controlled Morphology Particles: From the Nanometer to Submicrometer Size Ranges . Adv. Powder Technol. , 22 ( 1 ) : 1 – 19 .
- Noel , A. , Maghni , K. , Cloutier , Y. , Dion , C. , Wilkinson , K. J. Halle , S. 2012 . Effects of Inhaled Nano-TiO2 Aerosols Showing Two Distinct Agglomeration States on Rat Lungs . Toxicol. Lett. , 214 ( 2 ) : 109 – 119 .
- Oberdorster , G. , Oberdorster , E. and Oberdorster , J. 2005 . Nanotoxicology: An Emerging Discipline Evolving from Studies of Ultrafine Particles . Environ. Health Persp. , 113 ( 7 ) : 823 – 839 .
- Pauluhn , J. 2010 . Subchronic 13-Week Inhalation Exposure of Rats to Multiwalled Carbon Nanotubes: Toxic Effects are Determined by Density of Agglomerate Structures, Not Fibrillar Structures . Toxicol. Sci. , 113 ( 1 ) : 226 – 242 .
- Peltonen , L. , Valo , H. , Kolakovic , R. , Laaksonen , T. and Hirvonen , J. 2010 . Electrospraying, Spray Drying and Related Techniques for Production and Formulation of Drug Nanoparticles . Expert Opin. Drug Deliv. , 7 ( 6 ) : 705 – 719 .
- RNCOS, Industry Research Solutions . 2012 . Nanotechnology Market Forecast to 2014 . : 175
- Schmoll , L. H. , Elzey , S. , Grassian , V. H. and O’Shaughnessy , P. T. 2009 . Nanoparticle Aerosol Generation Methods from Bulk Powders for Inhalation Exposure Studies . Nanotoxicology , 3 ( 4 ) : 265 – 275 .
- Shin , W. G. , Wang , J. , Mertler , M. , Sachweh , B. , Fissan , H. and Pui , D. Y. H. 2009 . Structural Properties of Silver Nanoparticle Agglomerates Based on Transmission Electron Microscopy: Relationship to Particle Mobility Analysis . J. Nanopart. Res. , 11 ( 1 ) : 163 – 173 .
- Sotiriou , G. A. , Diaz , E. , Long , M. S. , Godleski , J. , Brain , J. Pratsinis , S. E. 2012 . A Novel Platform for Pulmonary and Cardiovascular Toxicological Characterization of Inhaled Engineered Nanomaterials . Nanotoxicology , 6 ( 6 ) : 680 – 690 .
- Srinivas , A. , Rao , P. J. , Selvam , G. , Goparaju , A. , Murthy , P. B. and Reddy , P. N. 2012 . Oxidative Stress and Inflammatory Responses of Rat Following Acute Inhalation Exposure to Iron Oxide Nanoparticles . Hum. Exp. Toxicol. , 31 ( 11 ) : 1113 – 1131 .
- Strom , H. and Sasic , S. 2012 . The Role of Thermophoresis in Trapping of Diesel and Gasoline Particulate Matter . Catal. Today , 188 ( 1 ) : 14 – 23 .
- Sullivan , R. C. , Moore , M. J. K. , Petters , M. D. , Kreidenweis , S. M. , Qafoku , O. Laskin , A. 2010 . Impact of Particle Generation Method on the Apparent Hygroscopicity of Insoluble Mineral Particles . Aerosol Sci. Technol. , 44 ( 10 ) : 830 – 846 .
- Sung , J. H. , Ji , J. H. , Park , J. D. , Song , M. Y. , Song , K. S. Ryu , H. R. 2011 . Subchronic Inhalation Toxicity of Gold Nanoparticles . Part. Fibre Toxicol. , 8 Article No. 16
- Tang , P. , Fletcher , D. F. , Chan , H. K. and Raper , J. A. 2008 . Simple and Cost-Effective Powder Disperser for Aerosol Particle Size Measurement . Powder Technol. , 187 ( 1 ) : 27 – 36 .
- Thorne , P. S. 1994 . Experimental Grain Dust Atmospheres Generated by Wet and Dry Aerosolization Techniques . Am. J. Ind. Med. , 25 ( 1 ) : 109 – 112 .
- To , D. , Dave , R. , Yin , X. L. and Sundaresan , S. 2009 . Deagglomeration of Nanoparticle Aggregates via Rapid Expansion of Supercritical or High-Pressure Suspensions . AIChE J. , 55 ( 11 ) : 2807 – 2826 .
- Tsai , C. J. , Lin , G. Y. , Liu , C. N. , He , C. E. and Chen , C. W. 2012 . Characteristic of Nanoparticles Generated from Different Nano-Powders by Using Different Dispersion Methods . J. Nanopart. Res. , 14 ( 4 ) Article No. 777
- Vehring , R. , Foss , W. R. and Lechuga-Ballesteros , D. 2007 . Particle Formation in Spray Drying . J. Aerosol Sci. , 38 ( 7 ) : 728 – 746 .
- Win-Shwe , T. T. and Fujimaki , H. 2011 . Nanoparticles and Neurotoxicity . Int. J. Mol. Sci. , 12 ( 9 ) : 6267 – 6280 .