Abstract
It is important to have well-defined, reproducible methods to evaluate and compare newly developed air filtration equipment. To facilitate accurate assessment of air purification devices at the bench scale, an experimental system was designed, built, and documented to evaluate particulate removal efficiency (PRE) of air filtration devices based on principles used in ASHRAE standards. The system was then carefully characterized and used to evaluate PRE and total energy consumption of a novel acoustically enhanced impaction (AEI) air purification device. The AEI device demonstrated 99.998% PRE of 0.5–1.5 μm diameter KCl particles while causing a 120 Pa pressure drop and requiring a total of 3.0 W/l of air treated at indoor ambient conditions. A single element of the AEI device operated in a biological safety level 2 facility was then used to evaluate PRE of bioaerosol consisting of Bacillus cereus (BC) spores. PRE of BC was 99.86 ± 0.05% at indoor ambient temperature and pressure. This research describes the use of the Bench-scale Air Purification Testing and Evaluation Chamber (BAP-TEC) to experimentally evaluate and compare PRE and total energy requirements of novel air purification devices at the bench scale (280–1400 alpm). Further, an AEI device containing a fibrous filter media and high intensity sound field in the same control volume is evaluated using the BAP-TEC. Temporally resolved PRE of a bioaerosol by the AEI is also presented.
Copyright 2013 American Association for Aerosol Research
INTRODUCTION
Many types of facilities require a high level of particulate removal in heating, ventilation, and air conditioning (HVAC) gas streams, which is achievable with high efficiency particulate air (HEPA) filters. A Class-5 clean room, often used in semiconductor manufacturing facilities, must contain less than 10,200 m−3 of particles with diameter ≥0.3 μm (ISO Citation1999). While this is to maintain integrity of a product, other guidance has been developed to protect occupants of indoor facilities from unintentional releases of bioaerosol in public locations (NIOSH Citation2002) and intentional release of biological warfare agents (BWAs) such as Bacillus anthracis (anthrax, BA) (US DoD Citation2008). The United States Centers for Disease Control has categorized BWAs as high-risk organisms (CDC Citation2012), and release of BWAs has been identified as a threat to the US civilian population (The White House Citation2004; Demirev et al. Citation2005). Control of BWAs and other bioaerosol is of particular interest due to secondary effects of biological contamination such as persistent biological growth (Lee 2011).
HEPA filtration is used to achieve high particle removal efficiency (PRE) values and remove sub-μm diameter particulate matter (PM) from HVAC systems (Bryden Citation2005). HEPA filters remove 99.97% of particles ≥0.3 μm diameter and induce a pressure drop of 250–1000 Pa depending on time in service and superficial gas velocity (Fisk et al. Citation2002). HEPA filters increase energy loads when compared with lower efficiency filtration (Rudnick Citation2004). The U.S. Army has recently defined goals for 25 installations with no net energy use by 2030 (US Army Citation2011). To achieve this goal, all reasonable methods for load reduction must be pursued (US DoD Citation2004, Citation2011).
Use of ASHRAE Method 52.2 is a consensus-based approach to evaluate and compare air purification equipment (ASHRAE Citation2007). It provides a method for testing PRE of polydisperse 0.30–10 μm diameter KCl particles at gas flow rates from 1.34 × 104 to 8.50 × 104 lpm. All gas flow rates reported below are at actual conditions (actual liters per minute, lpm) unless stated otherwise. While this is useful at the pilot scale, the gas flow rate is too large for evaluation of prototype bench-scale technologies (280–1400 lpm).
Multiple approaches to testing systems at the bench scale exist in the literature, but such methods are not fully characterized or explained (Griffiths et al. Citation2005; Liu at al. Citation2009a; Kim et al. Citation2012; Miaskiewicz-Peska and Lebkowska Citation2012) as described below. Kim et al. (Citation2012) describe the use of an environmental chamber utilizing a nebulizer to generate a Bacillus subtilis bioaerosol challenge to investigate PRE of medium efficiency filters. An SKC biosampler is used for sampling of the viable bioaerosol. Their approach is beneficial to measure total viable bioaerosol removal efficiency, but there is no information about graded (size-specific) PRE for the particle removal device. Griffiths et al. (Citation2005) demonstrated a similarly robust bioaerosol test method but their method is also not designed to determine graded PRE. Miaskiewicz-Peska and Lebkowska (Citation2012) utilize a two-step approach to first evaluate graded PRE of a test dust followed by bioaerosol removal using a second setup. While their system provides a method for testing overall filter performance as well as bioaerosol PRE, the maximum gas flow rate is 12 lpm, most suitable for small-scale lab tests. Similarly, the system described in Liu et al. (Citation2009a) is designed for a maximum flow of 32 lpm, making it unsuitable for testing prototype devices at the bench scale. Overall, the peer reviewed literature does not include a careful description of a method to design, build, and characterize a bench-scale (280–1400 lpm) system for evaluating novel air purification devices, as presented here with the Bench-scale Air Purification Testing and Evaluation Chamber (BAP-TEC). We describe the methods used for material and equipment selection, aerosol generation and mixing, aerosol sampling and analysis, and equipment validation to design and characterize a test chamber at the bench scale. Additionally, we correlate PRE of a polydisperse KCl aerosol at the bench scale with PRE of a viable bioaerosol measured at 5 lpm to verify that PRE observed using the KCl aerosol can be extended to a bioaerosol. This robust method can be utilized to evaluate PRE of polydisperse KCl aerosols by novel bench-scale air purification devices and verify that such results are also representative of bioaerosol removal efficiency. This manuscript provides a means to make meaningful comparisons of newly developed air purification technologies at the bench scale.
High intensity sound fields can induce aerosol movement (Goddard and Kaduchak Citation2005) and cause particle agglomeration in high-concentration gas streams (>105 particles/cm3) (Liu et al. Citation2009a). This agglomeration process has been studied to develop enhanced filtration systems (Reethof Citation1986; Shuster et al. Citation2002; Liu et al. Citation2009a,Citationb; Wang et al. Citation2011). In such systems, agglomeration is used to increase the mean particle size of an aerosol, thereby improving removal by impaction (Galica et al. Citation1994), gravitational settling (Liu et al. Citation2009a,Citationb), or filtration (Moldavsky et al. Citation2006). High intensity sound fields have also been exploited to concentrate particles prior to optical sizing (Holwill Citation2000), and to separate particles of a defined size range (Miles et al. Citation1995; Meegan and Gallia Citation2008). These approaches to enhance particle removal by means of acoustic fields utilize two-stage systems where aerosol agglomeration occurs separate from particle removal (whether by means of settling, cyclones, or filtration). Meegan and Gallia (Citation2008) demonstrated a single-stage device capable of removing sub-μm diameter particles from a continuous-flow gas stream. This was an effective approach for near-zero pressure drop and aerosol removal, but the complexity of the system was only amenable for demonstration up to a maximum gas flow of 5 lpm. This study also implements and evaluates a novel approach to concomitantly use high intensity sound fields within fibrous media in a single control volume to enhance PRE, a method we refer to as an acoustically enhanced impaction (AEI) device. The AEI device presented here is unique in that it does not rely on agglomerating an aerosol upstream of collection, but instead exploits the acoustically driven movement of particles in a high intensity sound field to increase the impaction of such particles onto a fibrous media contained in the same control volume as the sound field. Testing evaluates PRE of a polydisperse KCl test aerosol and a viable Bacillus cereus (BC) bioaerosol by an AEI device tested in the BAP-TEC. Also presented are scanning electron microscopy (SEM) images of KCl particles on the fibrous filter media as a result of the high intensity sound fields for visual verification of particle deposition on the fibers, temporally resolved PRE values of a viable bioaerosol at 0.2 Hz while cycling power to the AEI device to evaluate response time for particle removal, the potential for particle re-entrainment from the filter, and energy requirements for such device.
MATERIALS AND METHODS
Bench-Scale KCl Particle Removal Test
The BAP-TEC was comprised of sequential sections: airflow generation and pre-filtration, aerosol inlet, upstream mixing, upstream sampling port, air purification device, downstream mixing, downstream sampling port, and post-filtration. All tests were performed at indoor ambient room temperature, humidity, and pressure (21–23°C, 40–60% RH, 100.2–102.3 kPa, respectively). All sections were made of type 304 S.S. with 18 cm × 18 cm cross-section. Sections were electrically grounded, and the complete system was checked for electrical continuity. A rubber mat insulated the system from the laboratory bench. Each section was terminated with a 2.5 cm flange. Closed cell foam was applied to flanges prior to joining with clamps. Jack stands elevated and leveled the system ().
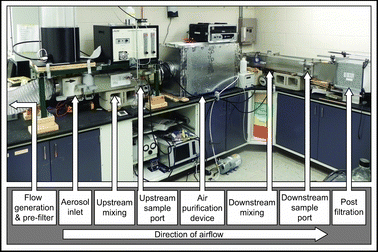
Airflow was generated with a forced-draft direct-drive blower (1TDP7, Grainger, Lake Forest, IL, USA). Blower speed was controlled with a variable voltage controller (3PN501B, Staco, Daton, OH, USA). A HEPA filter (6B617, Grainger) downstream of the blower was used for pre-filtration. Flow was directed to the centerline of the BAP-TEC using flexible ducting (Fabriflex Type 99, 3M, Maplewood, MN, USA). The BAP-TEC was designed to evaluate air filtration devices at 280–1400 lpm.
KCl test aerosol was generated (8108, TSI Inc., Shoreview, MN, USA) from an aqueous solution containing 30% by weight KCl (SigmaUltra ≥99.0%, Sigma-Aldrich, St. Louis, MO, USA) solute in reagent grade deionized water (Acros Organics, Geel, Belgium). Liquid feed to the atomizer was controlled with the integrated peristaltic pump held at setting 2 of 10. Dried, charge neutralized aerosol was introduced to the carrier gas via gravitational settling, transverse to the direction of gas flow. Mixing was achieved with turbulent flow in the duct. Terminal settling velocities were calculated for spherical particles of 0.5–1.5 μm diameters to ensure they would not settle from the carrier gas stream. Calculations were based on dry ambient indoor conditions (20°C, 101.2 kPa), and density of dry KCl particles was 1.98 g/cm3 at 25°C (Sigma-Aldrich Citation2012).
Upstream samples were extracted prior to the particle-laden carrier gas entering the air purification device. Pressure drop was monitored with a digital differential pressure gauge (D-1000, Dwyer, Michigan City, IN, USA). If the air purification device required power for operation, the load was monitored “at the wall” with an in-line load monitor (AmWatt Load Tester, Reliance Controls, Racine, WI, USA).
A fully turbulent downstream mixing section was placed after the air purification device followed by a downstream sample port. The particle-laden carrier gas was then passed through a HEPA filter before venting to the room.
Aerosol Sampling
All samples were isokinetically extracted with type 304 S.S. tubing. Conductive silicone tubing (Simolex Rubber Co., Plymouth Township, MI, USA) was used for sample lines. A wye junction was controlled manually by a straight ball valve (H6800, Ham-Let Group, Mississauga, ON, Canada) to switch between sample ports. Samples extracted upstream of the device were diluted by a factor of 100 using an aerosol diluter (3302A, TSI) because devices under testing are expected to achieve greater than 90% PRE. Downstream samples were not diluted.
Particle number concentration was measured with a laser aerosol spectrometer (LAS, 3340, TSI) operated with a LabVIEW interface. Samples were extracted at 3.1 lpm by a vacuum pump (0523, Gast, Benton Harbor, MI, USA) and data were collected continuously as 1 min integrated samples. Flow was controlled with a critical orifice immediately upstream of the pump. A 0.10 slpm sample was extracted from the 3.1 lpm flow immediately upstream of the LAS. Prior to testing, flow rates at all relevant junctions were verified with a piston meter (DC-1, Bios International, Butler, NJ, USA).
Airflow was determined from measurements of carrier gas velocity by a hot-wire anemometer (9555, TSI) at the upstream sample port. Uniformity of face velocity and aerosol concentration was characterized prior to PRE testing. An equally divided nine-section grid was defined for the cross-sectional area of the system and measurements were recorded from the center of each section.
Aerosol Sampling Equipment Validation
The LAS was calibrated for accuracy in measuring particle sizes and total particulate number concentration. Concentrated monodisperse polystyrene latex spheres (PSLs, Polysciences, Inc., Warrington, PA, USA) were diluted in nanopure water (Barnstead Nanopure, Lake Balboa, CA, USA) and aerosolized with an atomizer (3076, TSI), dried and charge neutralized. An electrostatic classifier (3071, TSI) was used upstream of the LAS to select the size range of interest. The LAS was operated in parallel with a scanning mobility particle sizer (SMPS, 3936, TSI) coupled to a condensation particle counter (CPC, 3010 with scanning chip, TSI) to inter-compare the results from both instruments and ensure that the calibration spheres were accurate in size.
A polydisperse aerosol was used to determine the accuracy in measuring aerosol number concentration. An aqueous solution containing 30% by weight KCl was atomized, dried, and neutralized. Simultaneous samples were taken with the LAS and CPC. Number concentrations reported by the LAS and CPC were compared to determine agreement in measurement of particle number concentration.
Measurement of Bacillus cereus Spore Removal Efficiency
BC has been used as a surrogate for BA to allow testing in a BSL 2 facility (Sagripanti et al. 2007). BC and BA are rod-shaped particles 1.0–1.7 μm long and 0.48–0.98 μm wide, corresponding to a mean equivalent spherical volume diameter of 1.0 ± 0.32 μm (Hinds Citation1999; Carrera et al. Citation2007). Particles of this size are easily deposited in the human respiratory system (Zhang Citation2004). For this study, BC spores (ATCC 4342) were selected for aerosolized testing due to the ease of rapidly producing high-concentration solutions. Spores were prepared and purified following a modification of the method by Nicholson and Setlow (Citation1990). Briefly, a pure culture of BC was streaked onto a nutrient agar plate and incubated overnight at 35°C. A single colony was inoculated into a 2 ml aliquot of NB in a 14 ml tube and grown for 8 h at 35°C, shaking at 220 rpm. After incubation, 10 ml of the cellular suspension was transferred to 250 ml sporulation medium in a 1 l flask and incubated for 5 days at 35°C, 220 rpm. Sporulation efficiency was measured by phase contrast microscopy, and spores were harvested once the fraction of phase bright spores was greater than 0.9. Harvested spores were washed three times in cold ultrapure water via (re)suspension and centrifugation at 3500 rpm in a benchtop centrifuge. Spores were subjected to heating at 70°C for 30 min to kill any remaining vegetative cells, followed by two additional rounds of washing. NaBr gradient separation was not used for purification of spore preparations because bioaerosol sampling was performed using a size-specific optical sizing method. Because particle size distribution of the bioaerosol was measured rather than total concentration of all bioaerosol, it was possible to discriminate spores from vegetative cells based on size. Measured particle diameters of aerosolized spores were consistent with literature values. The presence of monodisperse particles indicated that sufficient purity was achieved using the heat treatment method described above to remove vegetative cells, leaving only BC spores. Sporulation was further confirmed by heat resistance assays that demonstrated that the concentrations of the preparations were consistent with those samples not receiving heat treatment, indicating that spores remained in the viable population.
Purified stock solutions (1.2 × 109/ml) were stored at 4°C until use. These stocks were diluted 1000× in sterile deionized water to make lower-concentration solutions (∼106/ml), which were stored at 4°C and used for testing within 1 day. The viability of spore preparations was stable in solution for up to 6 months as determined by periodic viability assays using heat incubation (70°C for 30 min), serial dilution, and plate counting. Due to the stability of the spore preparations in solution, it was not necessary to culture the spore suspension prior to each experiment.
BC spore removal efficiency with the AEI was evaluated using a second, smaller-scale (5 lpm) system that was fully contained within a BSL-2 hood (SterilchemGARD III Advanced, The Baker Company, Sanford, ME, USA) to satisfy laboratory safety requirements. Therefore, it was necessary to utilize an aerosol generation system small enough to be enclosed within the hood. A nebulizer (3650D, Sunrise Medical, Fresno, CA, USA) was selected for aerosol generation as the TSI 8108 generator used in the BAP-TEC was too large to fit within an available BSL-2 hood. The nebulizer is suitable for generation of aerosol droplets containing microorganisms that have low sensitivity to shear forces, such as spores (Reponen et al. Citation1997). An integrated compressor and filter were used to supply air to the nebulizer. The compressor provided 8 lpm of filtered air to the nebulizer cup and the nebulization rate was no less than 0.15 ml/min. 5 ml of BC solution, enough for 20 min of testing, was added to the nebulizer cup prior to each trial. Laboratory compressed air, used for dilution, was conditioned with an oil trap (Sulzer-Metco, Winterthur, Switzerland), HEPA filter (HEPA-Vent, Whatman, Maidstone, UK), dispersion dryer (DD250, Air Techniques International, Baltimore, MD, USA), and a second HEPA filter ().
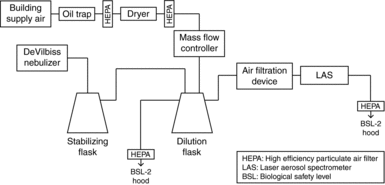
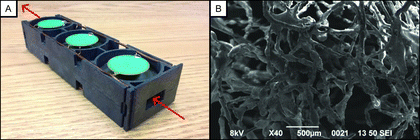
Two narrow-mouth Erlenmeyer flasks were used to stabilize and dilute the aerosol stream. The humidified carrier gas containing the bioaerosol was mixed with dry laboratory air to reduce humidity prior to passing through the air filtration device. Dilution air was controlled with a mass flow controller (Alicat Scientific, Tucson, AZ, USA) at 5 slpm. Dilution air and concentrated aerosol were mixed in the dilution flask at indoor ambient temperature and pressure to reduce fluctuations in aerosol concentration. All vented carrier gas containing BC was HEPA filtered before being exhausted into a BSL-2 hood.
Before performing PRE tests, the size of the dispersed BC was measured. The air filtration device was replaced with a straight section of S.S. tubing. Samples were extracted and analyzed by the LAS. After verifying the size of the inlet BC aerosol, the air purification device was installed in the test setup. The LAS recorded samples in 5 s time steps. The air purification device was cycled on and off while data were recorded for 8 min in triplicate.
Determination of Particle Removal Efficiency
PRE of a powered air purification device was determined by cycling the device on and off. Measurements were performed in triplicate, and mean PRE of the collection device is reported. Aerosol generation was started and gas flow established. With the device “off,” aerosol concentration was monitored at the downstream sample location. Once a uniform aerosol concentration was established, measurements were recorded. Next, power was supplied to the device and measurements were recorded. PRE was determined for 0.5–1.5 μm diameter particles using EquationEquation (1)[1] .
[1] where PRE is the particle diameter-dependent (graded) removal efficiency, Con is the downstream PM number concentration in specified diameter range with device on, and Coff is the downstream PM number concentration in specified diameter range with device off.
Imaging of the filter media and verification of KCl deposition to the fibers were performed before and after filtration tests using SEM (JSM-6380, JEOL, Peabody, MA, USA) with energy dispersive spectroscopy (EDS, Genesis 2000, EDAX, Mahwah, NJ, USA). Images of the media were collected before and after 8 h of exposure to KCl test aerosol at 280 lpm by removing it from the channel and cutting the filter into three sections.
Acoustically Enhanced Impaction Device
The AEI device is based on “building blocks” capable of treating 5 lpm each (). A block utilizes six piezo-electric sound sources—three on each side of a gas flow channel (1.9 cm × 0.95 cm × 18.5 cm). The channel is filled with a collection media ().
A bench-scale AEI device comprised of 60 building blocks operated in parallel (Applied Research Associates, Rocky Mountain Division, Littleton, CO, USA) was evaluated in the BAP-TEC. Each building block treated 4.7 lpm.
RESULTS AND DISCUSSION
Design and Fabrication of the BAP-TEC
The residence time of an air parcel in the BAP-TEC was based on the ASHRAE standard guide for the scaled design. Residence time was calculated based on the low flow rate for each section (). Low flow is 1.34 × 104 lpm for the ASHRAE system and 280 lpm for the BAP-TEC.
Table 1 Comparison of residence time and length of duct sections between ASHRAE Standard 52.2 and the new bench-scale system described in this article
Reynolds number (Re) for the sections described by the ASHRAE standard was determined for both high and low flow rates. Flow is fully turbulent, with Re > 4000 (2.36 × 104 to 1.51 × 105). Therefore, the BAP-TEC was designed with Re > 4000, consistent with the ASHRAE standard. The range of Re for all sections of the BAP-TEC was 4.33 × 103 to 1.73 × 104 at 280 and 1400 lpm, respectively.
Initial characterization of the uniformity of the upstream gas velocity and PM concentration determined that both were at maximum values at the centerline of the duct. Velocity uniformity measurements yielded a coefficient of variation (CV) of 23.8%. Gas velocity 3 cm from the walls of the BAP-TEC was less than 60% of centerline velocity. To establish a more uniform velocity profile, a plate was located 15 cm downstream of the aerosol injection nozzle. The plate was circular and centered in the duct covering 50% of the duct's cross-sectional area. It was coated with vacuum grease to prevent re-entrainment of impacted particles. After installation of the plate, at 280 lpm the CV for gas velocity and PM concentration were 6.9% and 9.4%, respectively. The KCl test aerosol was observed to have a count mean diameter of 0.72 ± 0.30 μm. At experimental conditions, removal of KCl particles by settling over the length of the chamber was less than 1.0% considering turbulent flow gravitational settling.
Aerosol Sampling Evaluation
Two sizes of PSL spheres were used to determine sizing accuracy of sub-μm diameter particles by the LAS. The vendor reported PSL diameters were 95.6 ± 1.2 nm and 199 ± 6 nm. The LAS reported the PSL diameters within 4% of the vendor's values. Average number concentration over 1 min as measured by the LAS was within 7.6 ± 4% of the CPC values. This result indicated that measurements collected with the LAS during PRE testing were accurate with respect to particle diameter.
Energy Consumption of the Bench-Scale AEI Device
When operating at full power and the design flow rate (280 lpm), the AEI device required 3.0 W/l to activate the piezo-electric crystals. The maximum sound pressure level at 1 m from the device never exceeded 68 dB due to the sound dampening system. No hearing protection was required at this level, and the sound was difficult to distinguish from other mechanical equipment in the lab. Static pressure drop across the AEI was 120 Pa during operation at 280 lpm. Assuming a fan efficiency of 75% (Bell 2008) a 120 Pa pressure drop accounts for 0.0043 W/l of fan power, a negligible amount compared to the power required for the sound sources.
Removal Efficiency of KCl by the Bench-Scale AEI Device
The AEI device removed 77.6 ± 2.4% of 0.5–1.5 μm diameter particles without the sound sources powered and 99.998 ± 0.001% when fully powered. Operation at 2.1 W/l resulted in 99.307 ± 0.182% removal of the same size range ().
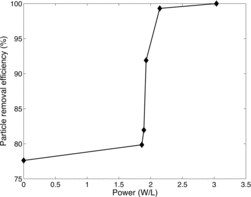
The filter media prior to PM collection was smooth with large inter-fiber distances (greater than 500 μm) (). Junctions between fibers were fused, creating a semirigid structure. Images show adhesion of particles to the smooth surface of the media (). Composition of the collected material was verified to be KCl due to detection of K and Cl peaks with the EDS.
Bacillus cereus Bioaerosol Size and Removal Efficiency
The measured diameter of dispersed BC was 0.98 ± 0.05 μm—consistent with the mean effective spherical volume diameter reported by Carrera et al. (Citation2007). Because there is potential variability in particle size due to the imperfect nature of spores, a 0.5–1.5 μm particle diameter range was used for particle measurements. A larger size range (0.90–10.0 μm diameter) was checked prior to data collection and the only observable peak was at 0.98 μm, verifying the existence of individual dispersed spores without agglomeration.
Under steady-state operating conditions at 3.0 W/l and 5 lpm, the BC removal efficiency was 99.86 ± 0.05%. Similar to above, BC removal efficiency without applying the sound field was 76 ± 2.0%. Particle number concentration during cyclic powering of the AEI on and off for one representative experiment is presented in . The response time to achieve a minimum number concentration after the device was powered on was 20 s. Similarly, when the device was powered off, the time to return to elevated concentration was 20 s.
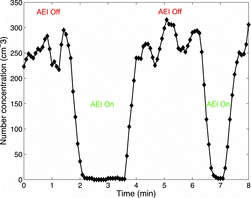
Effluent BC concentration returned to the initial “off” value when the device was switched off. Average background BC concentration downstream of the AEI with the device off was 260 ± 21.0 cm−3. In , the first period when the device was powered on lasted 110 s. Therefore, it is estimated that 2.4 × 105 ± 0.19 × 105 spores were collected in the device during that time. The lack of a surge in particle concentration after the device was turned off suggests that spores were firmly adhered to the filter media, or that the time scale of release was longer than investigated in this study.
Applications of AEI
The results herein are supportive of the use of AEI to purify indoor air streams containing inorganic or biological PM. AEI demonstrates a promising PM removal capability with a modest pressure drop. When operated at full power, it exceeded removal efficiency standards of HEPA: 99.97% PRE for 0.3 μm diameter particles and larger. Despite the AEI device's high PRE at low pressure drop, the overall energy efficiency was lower than what would be expected for a HEPA filter system. For comparison, a mid-life HEPA filter with a static pressure of 750 Pa requires a fan power of 0.017 W/l (Bell Citation2008). Accounting for the pressure drop and power to operate the sound sources, a mid-life AEI requires 3.0 W/l to achieve similar PRE for the same flow rate. However, the energy efficiency of the bench-scale AEI device can be improved by optimization of the piezo-electric elements and chamber geometry.
Additionally, the ability to vary purification efficiency by changing applied power is appealing. For instance, an AEI device could be installed in an HVAC system with a low-cost optical particle counter (OPC) located upstream to monitor total particle concentration in a particle size range of interest. Elevated particle concentration detected by the OPC would activate the device, thereby increasing PRE. Such approach would consume 0.0043 W/l for the AEI compared to 0.017 W/l for the HEPA unless an event occurs requiring activation of the AEI.
Using AEI in this manner would increase piezo-electric lifetime by reducing the duty cycle and have the advantage of lower energy requirements during normal operating conditions while allowing for a rapid response during a contamination event. The observed response time further supports the potential for application in a triggered setup with an OPC as it is capable of increasing bioaerosol PRE from 70% to greater than 99% within 20 s of powering on the device. Such a system could provide an alternative to full-time operation of HEPA filters for building protection applications.
REFERENCES
- ASHRAE. (2007). Method of Testing General Ventilation Air-Cleaning Devices for Removal Efficiency by Particle Size; ANSI/ASHRAE Standard 52.2-2007. American Society of Heating Refrigerating and Air-Conditioning Engineers Inc., Atlanta, GA.
- Bell, A.A. (2008). HVAC: Equations, Data, and Rules of Thumb,2nd ed. McGraw Hill, New York.
- Bryden, W. (2005). Immune Building Program, in Collective Protection Conference, National Defense Industrial Association, Monterrey, CA, USA. Available online at http://proceedings.ndia.org/5460/5460/1_bryden.pdf (accessed December 2012).
- Carrera, M., Zandomeni, R.O., Fitzgibbon, J., and Sagripanti, J.L. (2007). Difference Between the Spore Sizes of Bacillus anthracis and Other Bacillus Species. J. Appl. Microbiol., 102:303–312.
- CDC. (2012). Bioterrorism Agents/Diseases (by Category). Centers for Disease Control and Prevention, Atlanta, GA. . Available online at http://www.bt. cdc.gov/agent/agentlist-category.asp (accessed February 2013).
- Demirev, P.A., Feldman, A.B., and Lin, J.S. (2005). Chemical and Biological Weapons: Current Concepts and Future Defenses. J. Hopkins Apl. Tech. D., 26:321–333.
- Fisk, W.J., Faulkner, D., Palonen, J., and Seppanen, O. (2002). Performance and Costs of Particle Air Filtration Technologies. Indoor Air., 12:223–234.
- Galica, M.A., Campbell, A.H., and Rawlins, D.C. (1994). Subpilot-Scale Testing of Acoustically Enhanced Cyclone Collectors. Final Report to U.S. Department of Energy Office of Fossil Energy for Contract DE-AC21–88MC25010. . National Technical Information Service, US Department of Commerce, Springfield, VA, USA.
- Gallia, J., Meegan, D., and Zadler, B. (2012). Acoustic Fractionator Air Purification System. Final Report to ERDC-CERL for Contract W9132T-10-C-0012. . US Army ERDC-CERL, Champaign, IL, USA.
- Goddard, G., and Kaduchak, G. (2005). Ultrasonic Particle Concentration in a Line-Drive Cylindrical Tube. J. Acoust. Soc. Am., 117:3440–3447.
- Griffiths, W.D., Bennett, A., Speight, S., and Parks, S. (2005). Determining the Performance of a Commercial Air Purification System for Reducing Airborne Contamination Using Model Micro-Organisms: A New Test Methodology. J. Hosp. Infect., 61:242–247.
- Hinds, W.C. (1999). Aerosol Technology: Properties Behavior, and Measurement of Airborne Particles,2nd ed. Wiley-Interscience, New York.
- Holwill, I.L. J. (2000). The Use of Ultrasonic Standing Waves to Enhance Optical Particle Sizing Equipment. Ultrasonics, 38:650–653.
- ISO. (1999). Cleanrooms and Associated Controlled Environments – Part 1: Classification of Air Cleanliness. . ISO-14644-1. International Standardization Organization, Geneva, Switzerland.
- Kim, S.Y., Kim, M., Lee, S., Lee, J., and Ko, G. (2012). Survival of Microorganisms on Antimicrobial Filters and the Removal Efficiency of Bioaerosols in an Environmental Chamber. J. Microbiol. Biotechnol., 22(9):1288–1295.
- Lee, B.U. (2011). Life Comes from the Air: A Short Review on Bioaerosol Control. Aerosol Air Qual. Res., 11:921–927.
- Liu, J., Ruiying, Q., Li, Q., Han, G., and Qi, J. (2009a). Filtration of Bioaerosols Using Fibrous Air Filter Media. HVAC&R Res., 15(6):1165–1174.
- Liu, J., Zhang, G., Zhou, J., Wang, J., Zhao, W., and Cen, K. (2009b). Experimental Study of Acoustic Agglomeration of Coal-Fired Fly Ash Particles at Low Frequencies. Powder Technol., 193:20–25.
- Meegan, G.D., and Gallia, J.R. (2008). Acoustic Fractionator-Based Air Purification, in Chemical and Biological Defense Physical Science and Technology Conference, Defense Threat Reduction Agency, New Orleans, LA, USA.
- Miaskiewicz-Peska, E., and Lebkowska, M. (2012). Comparison of Aerosol and Bioaerosol Collection on Air Filters. Aerobiologia, 28:185–193.
- Miles, C.A., Morley, M.J., Hudson, W.R., and Mackey, B.M. (1995). Principles of Separating Micro-Organisms from Suspensions Using Ultrasound. J. Appl. Microbiol., 78:47–54.
- Moldavsky, L., Fichman, M., and Gutfinger, C. (2006). Enhancing the Performance of Fibrous Filters by Means of Acoustic Waves. Aerosol Sci., 37:528–539.
- Nicholson, W., and Setlow, P. (1990). Sporulation, Germination and Outgrowth, in Molecular Biological Methods for Bacillus, C. Harwood and S. Cutting, eds., John Wiley, New York, pp. 391–450.
- NIOSH. (2002). Guidance for Protecting Building Environments from Airborne Chemical, Biological, and Radiological Attacks. . Pub. No. 2002-139. National Institute for Occupational Safety and Health, Cincinnati, OH.
- Reethof, G. (1986). Acoustic Agglomeration of Power Plant Fly Ash for Environmental and Hot Gas Cleanup, in Fossil Fuels Utilization, R. Markuszewski and B.D. Blaustein, eds., American Chemical Society, Washington, DC, pp. 234–252.
- Reponen, T., Willeke, K., Ulevicius, V., Grinshpun, S.A., and Donnelly, J. (1997). Techniques for Dispersion of Microorganisms into Air. Aerosol Sci. Tech., 27:394–404.
- Rudnick, S.N. (2004). Optimizing the Design of Room Air Filters to Remove Submicrometer Particles. Aerosol Sci. Technol., 38:861–869.
- Sagripanti, J-L., Carrera, M., Insalaco, J., Ziemski, M., Rogers, J., and Zandomeni, R. (2007). Virulent Spores of Bacillus anthracis and other Bacillus Species Deposited on Solid Surfaces have Similar Sensitivity to Chemical Decontaminants. J. Appl. Microbiol., 102:11–21.
- Shuster, K., Fichman, M., Goldshtein, A., and Gutfinger, C. (2002). Agglomeration of Submicrometer Particles in Weak Periodic Shock Waves. Phys. Fluids., 4:1802–1805.
- Sigma-Aldrich. (2012). Potassium Chloride, . MSDS No. P3911.
- The White House. (2004). Homeland Security Presidential Directive 10: Biodefense for the 21st Century. . Available online at http://www.fas.org/irp/offdocs/nspd/hspd-10.html (accessed January 2013).
- US Army. (2011). Army Energy Program: Army Vision for Net-Zero. . Available online at http://army-energy.hqda.pentagon.mil/netzero/ (accessed December 2012).
- US DoD. (2004). Unified Facilities Criteria - Design: Clean Rooms. . UFC 4-310-02N. United States Department of Defense.
- US DoD. (2008). Unified Facilities Criteria – Procedures for Designing Airborne Chemical, Biological, and Radiological Protection for Buildings. . UFC 4-024-01. United States Department of Defense.
- US DoD. (2011). Unified Facilities Criteria – Design: Medical Military Facilities. . UFC 4-510-01. United States Department of Defense.
- Wang, J., Liu, J.Z., Zhang, G.X., Zhou, J.H., and Cen, K.F. (2011). Orthogonal Design Process Optimization and Single Factor Analysis for Bimodal Acoustic Agglomeration. Powder Technol., 210(3):315–322.
- Zhang, Y. (2004). Indoor Air Quality Engineering. CRC Press, Boca Raton, FL, pp. 13–20.