Abstract
This work concentrates on the simultaneous mobility and mass measurement of negative ions generated by the ionizing radiation in a 241Am aerosol charger in N2 (5.0), a 1:1-mixture of N2 and synthetic air, pure synthetic air (5.0), and filtered laboratory air at ∼30% relative humidity. Therefore, a high-resolution mobility analyzer (UDMA) and an atmospheric pressure interface time-of-flight mass spectrometer (APi-TOF) were operated in series. Experiments with N2 as carrier gas showed a dominating signal at an electrical mobility of 2.09 cm2/Vs with 90% of the ions being nitrate based. The ion composition was altered after a baking-out to a spectrum with three strong mobility-peaks at Z1 = 2.34 cm2/Vs, Z2 = 1.42 cm2/Vs, Z3 = 1.08 cm2/Vs and a higher diversity of ions in the corresponding mass spectra. The carrier gas was gradually changed from N2 (5.0) to a 1:1-mixture of N2 with synthetic air and pure synthetic air (5.0), having only a minor effect on the overall pattern of the ion spectrum. Using room air leads to a domination of the nitrate based ions. The mobility-dependent transmission efficiency of the UDMA was modeled using an empirical, laminar diffusion deposition model. The data were further compared to an empirical mass-mobility relationship to evaluate the fragmentation of the ion clusters in the inlet of the mass spectrometer. This study suggests that the nitrate ion, NO3 −, is found to be the dominant ion species produced in an aerosol charger, and that it may be mostly responsible for the charging of aerosol particles in negative polarity.
Copyright 2014 American Association for Aerosol Research
1. INTRODUCTION
Many aerosol particle detection or size classification methods rely on electrical detection or size classification of the particles (Flagan Citation1998). Accordingly, an efficient and controlled charging or neutralization of the particles is one of the crucial prerequisites for the correct determination of particle number size distributions (Wiedensohler et al. Citation2012). However, the nature and the properties of the ions that are responsible for aerosol charging in commonly used radioactive aerosol neutralizers are unknown or not well defined. This is particularly important at very small sizes, especially sub-3 nm, where atmospheric clustering occurs (Kulmala et al. Citation2013) and a detailed knowledge of the properties of the ions that contribute to the particle charging is essential (Kulmala et al. Citation2012). At this size, making the distinction between the measured particles and charging ions is challenging (Manninen et al. Citation2011; McMurry et al. Citation2011). Furthermore, recent experiments on ion properties and charging probabilities show that the ion species produced in a standard aerosol charger strongly depend on the composition of the carrier gas (Steiner and Reischl Citation2012).
Given sufficiently high ion concentrations, electrical mobility spectrometry (EMS) is one of the methods suitable for sub-3 nm measurements. The mobility resolving power of EMS instruments has advanced to the point that high-resolution differential mobility analyzers (HR-DMAs) now enable high-resolution mobility analysis of single-molecular clusters (Rossell-Llompart et al. Citation1996; Rosser and Fernández de la Mora Citation2005; Brunelli et al. Citation2009; Steiner et al. Citation2010; Fernández de la Mora and Kozlowski Citation2013).
Another essential advance in recent years is the development of mass spectrometers that are able to efficiently transfer molecular clusters from atmospheric pressure into the high vacuum of a mass spectrometer (Junninen et al. Citation2010; Ehn et al. Citation2011; Hogan and Fernández de la Mora Citation2011).
The aim of this study is to explore the role of carrier gas on the ion concentration and ion composition in a 241Am aerosol charger. The experiments were conducted with the atmospheric pressure interface time-of-flight mass spectrometer (APi-TOF, Junninen et al. Citation2010) and a Vienna-type HR-DMA, also referred to as the UDMA (Steiner et al. Citation2010), operated in series. The setup enables simultaneous measurement of electrical mobility (and corresponding mobility equivalent diameter) and m/z ratio of the ion clusters generated in the aerosol charger. Accordingly, this study tries to elucidate the ion species involved in the aerosol charging in a radioactive bipolar aerosol charger. The results help to improve current understanding of aerosol charging.
2. INSTRUMENTAL SETUP
The experimental setup is shown in . The high-resolution UDMA and the APi-TOF were operated in series. The 241Am aerosol charger was located directly at the ion inlet of the UDMA, which was operated in a closed loop sheath air arrangement at approximately 700 L min−1. Here we focused only on negative polarity. The UDMA had a resolution power R of approximately R = 30 at a particle size of 1.47 nm, following the resolution definition of Flagan (Citation1999). More details on the UDMA are described in Steiner et al. (Citation2010). All tubing material was chosen to be either stainless steel or Teflon to minimize contamination from the tubing.
FIG. 1 Experimental setup of UDMA and APi-TOF in series. The radioactivity based 241Am aerosol charger is located directly at the ion-inlet of the UDMA. After the UDMA, the classified ions are detected by means of a custom built Faraday cup electrometer (FCE) and the APi-TOF. To study the dependency of the generated ions on the carrier gas, different experiments involving N2 (5.0), synthetic air (5.0) and filtered room air were performed.
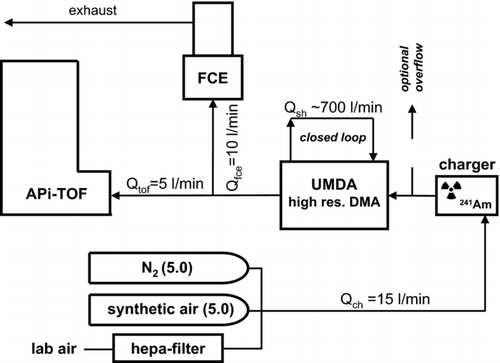
After the UDMA, the sample flow was split to two. One part entered a custom built Faraday cup electrometer (FCE), a modernized version of FCE used in Winklmayr et al. (Citation1991). The remaining sample flow was supplied to the APi-TOF for mass-to-charge analysis. A detailed review on the design and principle of operation of the APi-TOF was presented by Junninen et al. (Citation2010). All APi-TOF data processing was done using a Matlab-based program tofTools (Junninen et al. Citation2010).
For simultaneous determination of ion mobility and ion mass, the UDMA was first set to scanning mode to analyze the complete accessible mobility/size spectrum of the ions. Then, after identifying the mobility peaks of interest, the UDMA was set to classifying mode, selecting one narrow mobility fraction at a time for the mass to charge analysis with the APi-TOF.
To study the dependence of the ion mobility and composition on the carrier gas, four types of experiments with different carrier gases were performed. In the first experiment, the charger was exclusively supplied with N2 (5.0) with no additional cleaning. The next experiment was done after the charger was baked-out at 80°C in an oven and simultaneously flushed with nitrogen for 12 h. In the following experiments the carrier gas was changed to a mixture of 50% N2 (5.0) and 50% synthetic air (5.0) and further to 100% synthetic air. The final experiment was carried out using filtered, but humid (relative humidity ∼30%) laboratory air.
3. RESULTS AND DISCUSSION
Here, we present the electrical mobility at a temperature of 293 Kelvin, mobility equivalent diameter, total ion number concentration, and the corresponding mass spectrum for each selected mobility fraction and carrier gas composition. All mobility equivalent diameters presented in this work are calculated according to the Stokes-Millikan formalism, using the coefficients for the slip correction factor from Fuchs (Citation1964).
3.1. Nitrogen
The first mobility measurements of negative ions in nitrogen 5.0 () show a pattern that was already observed in earlier studies (De Juan and Fernández de la Mora Citation1998; Alonso et al. Citation2009; Steiner and Reischl Citation2012), where one peak exclusively dominated the spectrum. Here, a peak-mobility of Z = 2.09 cm2/Vs, corresponding to a mobility equivalent diameter of 0.98 nm was determined. The total number concentration of ions in this experiment was 1.45×105 cm−3.
FIG. 2 (a) First number-size-distribution of ions generated in N2 with the dominant peak at 0.98 nm/2.09 cm2/Vs. (b) Corresponding mass spectrum of the classified ions with Z 1 = 2.09 cm2/Vs with dominating peaks: NO3 − (m/z integer 62 Th) and HNO3·NO3 − (m/z integer125 Th) peaks. Other strong signals originate from NO2 − (integer m/z 46) and H2O·NO3 − cluster (integer m/z 80).
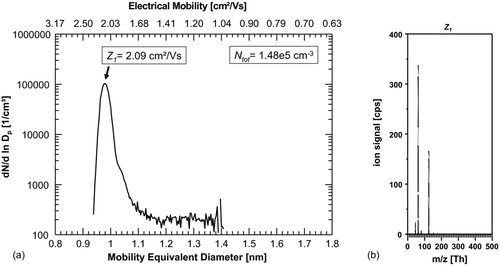
shows the mass spectrum corresponding to the classified ion fraction with mobility Z = 2.09 cm2/Vs. As can be seen, NO3 − (nitrate ion, integer m/z 62) and HNO3·NO3 − (nitrate ion dimer, integer m/z 125) clearly dominate the spectrum. Other strong signals originate from NO2 − (integer m/z 46) and the H2O·NO3 − cluster (integer m/z 80). In the mass range from 1–1000 Th, 90% of the total detected ions origins from these ions.
The charger used in this study had been used as an aerosol charger for ambient particle measurements on a regular basis. Accordingly, it is very likely that a contamination of nitric acid on the inner walls of the charger would provide the dominant ion composition of nitric acid clusters. To address this issue, the charger was baked out over night at 80°C in an oven while being continuously flushed with 0.3 L min−1 N2 (5.0) to minimize the effects from adsorbed contaminants from ambient air. After the baking-out, the number size distribution and mass distribution of the generated ions changed dramatically, as shown in and b.
FIG. 3 (a) Number-size-distribution of ions generated in the charger after the baking-out. Three significant peaks can be found at 2.34, 1.42, and 1.08 cm2/Vs. The total number concentration of ions increases to 9.38 × 105 ions/cm3. (b) Corresponding mass spectra of the three significant size/mobility-peaks. Presently, we can only speculate about the actual cluster composition and give examples for possible configurations: the lowest selected mobility fraction (Z3 ) is dominated by integer m/z 213 (C9H10O2 .NO3 −), 425 (C9H10O2 .HNO3NO3 −), and 461 ((C9H10O2)2 .HCl.HNO3NO3 −). Z2 already shows a transition to smaller masses as I− (integer m/z 127) and NO3 − (integer m/z 62) arise Z1 is again dominated by HNO3·NO3 − (nitrate ion dimer, integer m/z 125) and NO3 − (integer m/z 62); other strong signals originate from lactic acid (m/z 89) and nitric acid trimer ((HNO3)2NO3 −, m/z 188).
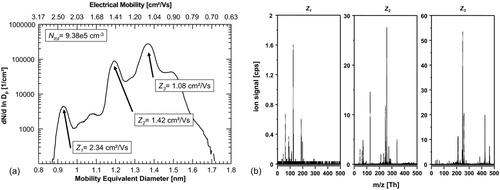
The ion number-size distribution changed from a single dominant peak () to a spectrum with at least three strong peaks () at ion mobilities Z1 = 2.34 cm2/Vs, Z2 = 1.42 cm2/Vs, Z3 = 1.08 cm2/Vs. In total at least five peaks can be found in the mobility spectrum. After the baking-out, the total number concentration of detected ions increased approximately by a factor of six to 9.4×105 ions cm−3. The most reasonable explanation for the appearance of the multiple additional peaks in the mobility and mass spectra is that the nitric acid impurity in the charger was significantly reduced by the evaporation. HNO3 is one of the most abundant substances with high gas phase acidity in the atmosphere (Danalatos and Glavas Citation1999 and references in there). Accordingly, the formation of NO3 − and its clusters is very likely after bipolar ionization. It should be noted that, in environments where a substance with even higher gas phase acidity than HNO3, for example sulfuric acid (H2SO4), is significant, a large fraction of the negative ions would be HSO4 − and associated clusters. If strong acids like HNO3 or H2SO4 are missing, other compounds with higher proton affinity (lower gas phase acidity) can be charged, leading to a more complex mobility and mass spectrum as observed in the atmospheric ion population (Ehn et al. Citation2011). After baking-out, a higher diversity of clusters is also seen from the measured corresponding mass spectra presented in .
The lowest selected mobility fraction (Z3 ) gives out a rather complex mass spectrum that is dominated by integer m/z 213 (C9H10O2 .NO3 −), 425 (C9H10O2 .HNO3NO3 −), and 461 ((C9H10O2)2 .HCl.HNO3NO3). It has to be pointed out that, at present, we can only speculate about the actual composition and configuration of these clusters and can only give examples of the possible cluster configurations. The middle peak electrical mobility fraction (Z2 ) already showed a transition toward smaller masses as I− (integer m/z 127) was detected. The selected peak with the highest electrical mobility (Z1 ) was dominated by HNO3·NO3 − (nitrate ion dimer, integer m/z 125); other strong signals originate from lactic acid (m/z 89) and nitric acid trimer ((HNO3)2NO3-, m/z 188).
FIG. 4 (a) Number-size-distribution of negative ions produced in a 1:1-mixture of N2 (5.0) and synthetic air (5.0). The addition of synthetic air has only a minor effect on the overall pattern of the ion spectrum. Only the peak at 0.92 nm/2.34 cm2/Vs changed into a double-peak structure. (b) Corresponding mass spectra of the four significant size/mobility-peaks, showing a very similar pattern with the earlier N2 experiment.
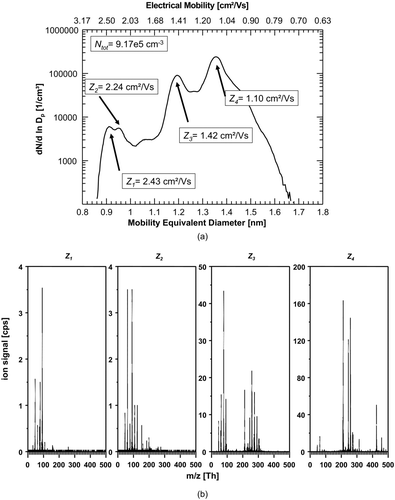
FIG. 5 (a) Number-size-distribution and of negative ions produced in synthetic air (5.0). A difference to the experiment using the 1:1-mixture of N2 (5.0) and synthetic air (5.0) can only be found in a slightly higher total ion number concentration of 1.08 × 106 ions/cm3. (b) The corresponding mass spectra of the four significant size/mobility-peaks using exclusively synthetic air also strongly resembles the situation of the 1:1-mixture of N2 (5.0) and synthetic air (5.0).
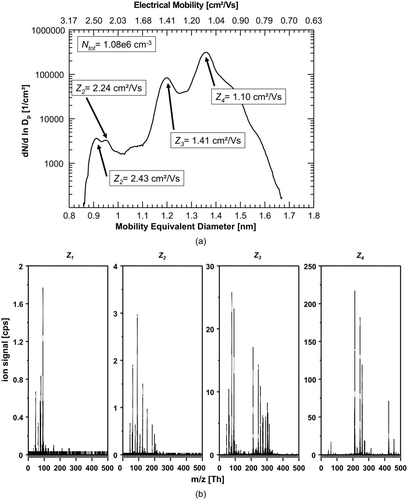
3.2. Synthetic Air
For the second set of experiments, the gas flow through the charger was changed from pure nitrogen to a 1:1-mixture of nitrogen (5.0) and synthetic air (5.0) (), and further in the third experiment to 100% synthetic air ().
Adding synthetic air to the system had only a minor effect on the overall pattern of the ion spectrum. Only the peak at 0.92 nm/2.34 cm2/Vs changed into a double-peak structure. The mass spectrum also showed a very similar pattern to the earlier experiment. It is notable that formic acid, HCOO−, at integer m/z 45 appears in the spectrum and chlorine, Cl−, which was present in the N2 spectrum at integer m/z 35/37 reduced significantly.
3.3. Filtered Room Air
The last experiment was carried out by supplying the aerosol charger with filtered but humid (∼30% RH) laboratory air as carrier gas (), leading again to a dramatic change in the ion spectrum with one single mobility/size peak close to 1 nm with an electrical mobility of Z1 = 2.09 cm2/Vs. In the corresponding mass spectrum, the nitrate ion (integer m/z 62) and its dimer (integer m/z 125) dominated the signal.
FIG. 6 (a) Number-size-distribution of negative ions produced in filtered (HEPA-filter), humid (rH∼30%) laboratory air. Again, a single mobility/size peak close to 1 nm with an electrical mobility of Z1 = 2.09 cm2/Vs is dominating the spectrum. (b) The corresponding mass spectra of the dominant size/mobility-peak again shows dominant signals of the nitrate ion (integer m/z 62) and its dimer (integer m/z 125). Apparently, by using a “contaminated” carrier gas, such as room air, the situation of the first experiments as presented in Figures 2a and b can be reproduced.
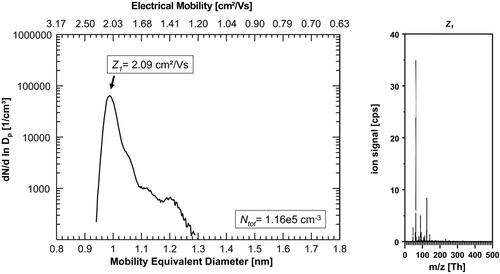
Approximately 78% of the total ions originated from NO3 −, HNO3 NO3 −, and (H2O) NO3 −. Apparently, by using a “contaminated” carrier gas, such as room air, the situation of the first experiments as presented in and b can be reproduced quite accurately. Even the total number concentration of 1.48 × 105 ions cm−3 closely resembled the results from the first experiment. Evidently, nitric acid was introduced to the charger by using room air as a carrier gas and is again dominating the ion generation.
3.4. Total Number Concentration
For each experiment, the total number concentration of ions was determined by integrating the measured number distributions. On first view, the total ion concentrations seemed to vary significantly from 1.48 × 105 ions cm−3 to almost 1×106 ions cm−3 before and after the baking-out, respectively. Generally, this appears to be peculiar as one would expect the ionizing radiation in the 241Am charger to generate a steady-state concentration of ions. However, in different experiments the mobility and composition of ions changed, and the total ion concentrations for each experiment are biased due to mobility dependent transport losses in the HR-DMA. The measured total number concentration of ions should be conserved, if increased diffusion losses of small ions and the mobility/size dependent transmission efficiency of the HR-DMA, with a decreased performance for small ions, are taken into account.
Unfortunately, no comprehensive measurements of the penetration efficiency of the UDMA are available as the development of the UDMA has focused on the improvement of its mobility resolving power rather than the ion transmission efficiency. However, previously Steiner et al. (Citation2010) determined a penetration efficiency of approximately 6.5% for 1.47 nm clusters under similar operating conditions. Taking this as the starting point, and following the approach of Jiang et al. (Citation2011), we estimated that an effective length L eff of 22 m characterizes the losses in the UDMA. This calculation is based on the laminar flow diffusion model of Gormley and Kennedy (Citation1949). The estimated losses in the UDMA are depicted in , along with the estimated variation of 30% of the effective length L eff.
According to the model, in the typical size range of charger generated ions of about 1–1.5 nm, the penetration efficiency of the UDMA ranges from 0.5% for 1 nm ions to 8.3% for 1.5 nm ions. Accounting for the increased diffusion losses, the total number concentrations of ions in the different experiments compare much better (). The black bars indicate the uncorrected data, as also displayed in Figures –. The data presented by the grey bars accounts for diffusion losses, calculated by the size-dependent laminar diffusion deposition model. The error bars indicate the change in the corrected total number concentration by an assumed variation of the effective length L eff of ±30%. This way the maximum difference of the concentrations reduced from almost an order of magnitude to a factor of two, which is within the estimated error.
FIG. 7 Estimated size-dependent penetration efficiency of the high-resolution UDMA using a laminar diffusion deposition model with an effective length L eff of 22 m and a sample flow rate of 15 l/min. The shaded area corresponds to an assumed variation of 30% of the effective length L eff. In the typical size range of charger generated ions of about 1–1.5 nm, the penetration efficiency of the UDMA ranges from 0.5% for a 1 nm particle to 8.3% for a 1.5 nm particle; corresponding to a difference of a factor of 17.
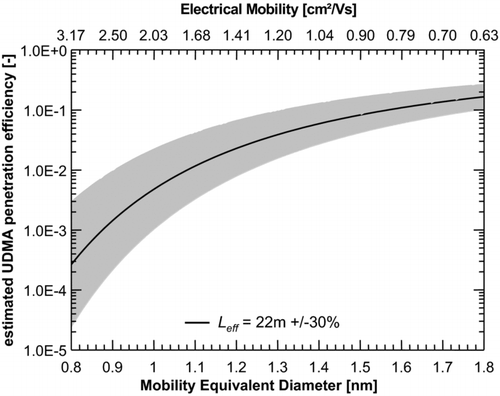
FIG. 8 Comparison of the total number concentration of ions during the course of the experiments. The black bars indicate the uncorrected data, as also presented in Figures 2–6. The grey bars account for diffusion losses, calculated by the size-dependent laminar diffusion deposition model. The error bars indicate the change in the corrected total number concentration by an assumed variation of the effective length L eff of ±30%. This way, the maximum difference of the concentrations reduces from a factor of 8 to a factor of 2.
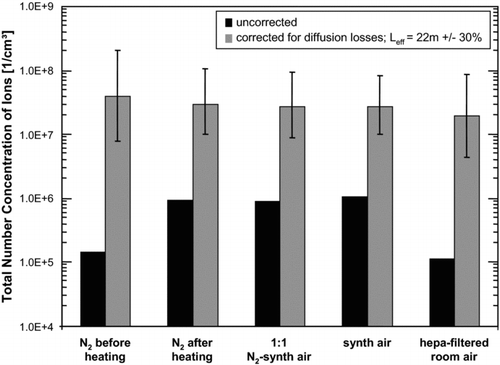
Another additional processes that probably will affect the total concentration of ions leaving the bipolar 241Am charger are ion-ion recombination effects. As this study focuses exclusively on the measurement of negative ions, no measurements of the corresponding positive ions are available. Moreover, any estimate of their properties would be too uncertain to allow a quantitative analysis. Overall, the present results suggest that the total number concentration of ions is conserved in the charger and that all available ions distribute over the mobility/mass spectrum according to the proton affinities of the compounds in the carrier gas.
3.5. Mass–Mobility Relationship
The simultaneous measurement of mobility and mass enables the comparison of the data with empirically derived mass-mobility relationships. The most frequently used mass mobility relationship is that of Kilpatrick (Citation1971) who determined the electrical mobilities of twenty five different positive and negative ions using a plasma chromatograph in clean and dry air and/or nitrogen. The masses of the very different species ranged from about 35–2211 m/z. Kilpatrick determined the mobilities of the molecular clusters at a temperature of about 200°C. Kilpatrick converted his data into a so-called “reduced” mobility Z0 , by correcting his values to a temperature of 273 K and a pressure of 760 Torr using the Langevin rule given in equation (1):
summarizes measured and reduced electrical mobilities of the selected mobility fractions, and the corresponding mean masses calculated as weighted average of the respective mass spectrum.
TABLE 1 Experimentally determined electrical mobility, reduced electrical mobility (Langevin rule), and mean m/z of selected mobility fractions of charger generated negative ions
Other authors have examined the relationship between mass and mobility-equivalent diameters of molecular clusters, and found good agreement between the mobility-equivalent diameter and the volume diameter when the latter was augmented by 0.23 nm (Tammet Citation1995) or 0.3 nm (Ku and Fernández de la Mora Citation2009; Ehn et al. Citation2010; Larriba et al. Citation2011). However, the conversion from electrical mobility into mass remains uncertain due to its dependence on parameters such as the size of the carrier gas molecules, the cluster density, and its physical structure. The conversion into a reduced mobility using the Langevin rule is also uncertain (Tammet Citation1995; Tammet Citation1998), but is nonetheless used to facilitate comparison of the different datasets.
compares the present data (black datapoints) with Kilpatrick's original data and the corresponding fit of Mäkelä et al. (Citation1996), the data of Ku and Fernández de la Mora (Citation2009), and those of Larriba et al. (Citation2011). The data of Kilpatrick (Citation1971), Ku and Fernández de la Mora (Citation2009), and Larriba et al. (Citation2011) have also been fit to a power law of the form,
FIG. 9 Reduced electrical mobility versus mass of ionic molecular clusters. Data derived during this study (black dots) is compared to data from Kilpatrick (Citation1971), Ku and Fernández de la Mora (Citation2009), Larriba et al. (Citation2011), a fit-function by Mäkelä et al. (Citation1996) to Kilpatrick's data and a power fit to the data by Kilpatrick (Citation1971), Ku and Fernández de la Mora (Citation2009), and Larriba et al. (Citation2011).
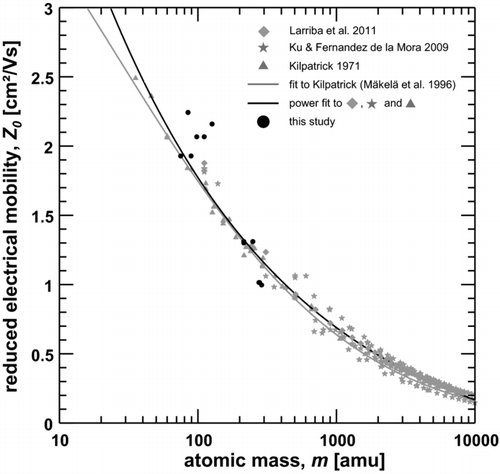
As can be seen from , the data points in the range of the reduced mobilities from 1.02 to 1.31 cm2/Vs (corresponding to Z 2 of the experiment with N2 after the baking-out and Z 3 and Z 4 of the experiments with the 1:1-mixture of N2 with synthetic air and pure synthetic air) agree quite well with the literature data and their corresponding fit functions. The data for a reduced mobility of Z0 = 1.93 cm2/Vs (Z = 2.09 cm2/Vs), corresponding to Z 1 of the experiments before the baking-out and the HEPA-filtered room air, agree very well with the other data.
However, the mean masses for the selected fractions Z 1 of the experiment with N2 after the baking-out, and Z 1 and Z 2 of the experiments with the 1:1-mixture of N2 with synthetic air and pure synthetic air (reduced mobility 2.07–2.24 cm2/Vs) show large deviations form the trend of the other data, and yield too high masses compared to the mass mobility relationship.
This deviation (similar to the situation of the HR-DMA) could be attributed to a mass dependent transmission efficiency of the APi-TOF with a decreased performance for smaller clusters which may cause the signal for small clusters to be underestimated, leading to an overestimate of the mean mass for a selected mobility fraction. In this study, the APi-TOF was operated with optimized transmission for the larger ions.
The simultaneous mass-mobility measurement gives information on fragmentation processes of the mobility selected molecular ion clusters inside the mass spectrometer if the measured mass of a mobility-selected ion cluster yields a smaller mass than predicted by the mass-mobility relationship (again keeping the limitation in mind). Accordingly, the almost perfect match of the data corresponding to the experiments with N2 before the baking-out of the charger and with HEPA-filtered room air as carrier gas at reduced mobility Z0 = 1.93 cm2/Vs (Z = 2.09 cm2/Vs), suggests that the signal of the nitrate ion (integer m/z 62) is not caused by a fragmentation of its dimer cluster (integer m/z 125). Instead, it suggests that the nitrate ion, rather then its dimer, is the predominant ion species in these experiments.
4. CONCLUSION
In this work, the mobility and mass spectra of negative ions generated in a commonly used radioactivity based 241Am charger were studied with different carrier gas composition. Our results corroborate previous studies that concluded that contaminants in the carrier gas showed a remarkable influence on the mobility spectra of the generated ions. Beginning with nitrogen as carrier gas, the nitrate ion (integer m/z 62) and its dimer (integer m/z 125) produced the dominant signal. After baking-out and cleaning of the charger, the results changed. With the absence of the nitric acid contamination on the inner walls of the charger, many other compounds with higher proton affinities acquired charge and appeared in the mobility and mass spectra. We tentatively identified some of the compounds, such as the nitrate ion, the nitrate ion dimer and trimer (integer m/z 62, 125, and 188), and lactic acid (m/z 89).
The influence of the carrier gas on the generated ion species was investigated by a stepwise change of the carrier gas from pure nitrogen (5.0), to a mixture of nitrogen (5.0) and synthetic air (5.0), and to pure synthetic air (5.0). Only minor differences in the generated ion species were detected in these experiments. Significant changes in the mobility and mass spectra were again found during the change to filtered, but humid room air as the carrier gas. This mixture apparently again introduced nitric acid into the charger, resulting in a clearly dominating signal of the nitrate ion and the nitrate ion dimer.
At first, the measured total number concentrations of ions seemed to vary significantly. However, we were able to attribute this to the size-dependent penetration efficiency of the high-resolution UDMA, and to the increased diffusion losses of the smaller ions. After applying a size dependent laminar diffusion deposition model, the maximum difference of the concentrations reduced to a factor of two.
Comparing our data with literature data and with an empirical mass-mobility relationship suggests that the dominant nitrate ion signal is not caused by a fragmentation of its dimer cluster. Our results suggest that the abundance of nitric acid in room (laboratory) air is sufficient to clearly dominate the production of negative ions. At room air conditions, typically encountered in many EMS aerosol measurements, the nitrate ion NO3 − at m/z integer 62, with a corresponding electrical mobility of Z = 2.09 cm2/Vs, was found to be the dominant ion species, and may be mostly responsible for the charging of aerosol particles in the negative polarity. This finding advances the understanding of the charging mechanisms in the 1 nm size range. If the charger-generated ions are predominantly nitrate ions, the charge transfer may follow the rules of chemical ionization. This means that the generated ions only react with compounds that are more acidic than HNO3 – making the charging of aerosol particles dependent on their chemical composition, at least in the sub-3 nm size range.
It should be noted that this study exclusively focuses on a 241Am charger. The mobility distributions of ions produced using different aerosol chargers (e.g., corona discharge or soft x-ray sources) show a very similar pattern (Kallinger et al. Citation2012), so the findings of this study are likely valid for other charging techniques.
FUNDING
This study was supported by the Austrian Science Fund FWF under grant P20837-N20, the PEGASOS project funded by the European Commission under the Framework Programme 7 (FP7-ENV-2010-265148), and European Research Council (ERC Advanced Grant ATM-NUCLE), and the Academy of Finland (251427, 139656, 141135).
REFERENCES
- Alonso , M. , Santos , J. P. , Hontanón , E. and Ramiro , E. 2009 . First Differential Mobility Analysis (DMA) Measurements of Air Ions Produced by Radioactive Source and Corona . Aerosol Air Qual. Res. , 9 : 453 – 457 .
- Brunelli , N. A. , Flagan , R. C. and Giapis , K. P. 2009 . Radial Differential Mobility Analyzer for One Nanometer Particle Classification . Aerosol Sci. Technol. , 43 ( 1 ) : 53 – 59 .
- Danalatos , D. and Glavas , S. 1999 . Gas Phase Nitric Acid, Ammonia and Related Particulate Matter at a Mediterranean Coastal Site, Patras, Greece . Atmos. Environ. , 33 : 3417 – 3425 .
- De Juan , L. and Fernández de la Mora , J. 1998 . High Resolution Size Analysis of Nanoparticles and Ions: Running a Vienna DMA of Near Optimal Length at Reynolds Numbers up to 5000 . J. Aerosol Sci. , 29 : 617 – 626 .
- Ehn , M. , Junninen , H. , Petäjä , T. , Kurten , T. , Kerminen , V. M. Schobesberger , S. 2010 . Composition and Temporal Behavior of Ambient Ions in the Boreal Forest . Atmos. Chem. Phys. , 10 : 8513 – 8530 .
- Ehn , M. , Junninen , H. , Schobesberger , S. , Manninen , H. E. , Franchin , A. Sipila , M. 2011 . An Instrumental Comparison of Mobility and Mass Measurements of Atmospheric Small Ions . Aerosol Sci. Technol. , 43 ( 4 ) : 522 – 532 .
- Fernández de la Mora , J. and Kozlowski , J. 2013 . Hand-Held Differential Mobility Analyzers of High Resolution for 1–30 nm Particles: Design and Fabrication Considerations . J. Aerosol Sci. , 57 : 45 – 53 .
- Flagan , R. C. 1998 . History of Electrical Aerosol Measurements . Aerosol Sci. Technol. , 28 ( 4 ) : 301 – 380 .
- Flagan , R. C. 1999 . On Differential Mobility Analyzer Resolution . Aerosol Sci. Technol. , 30 ( 6 ) : 556 – 570 .
- Fuchs , N. A. 1964 . The Mechanics of Aerosols , Oxford : Pergamon Press .
- Gormley , P. G. and Kennedy , M. K. 1949 . Diffusion from a Stream Flowing Through a Cylindrical Tube . Proc. R. Ir. Acad. , A52 : 163 – 169 .
- Hogan , C. J. and Fernandez de la Mora , J. 2011 . Ion Mobility Measurement of Non-Denatured 12–150 kDa Proteins and Protein Multimers by Tandem Differential Mobility Analysis – Mass Spectrometry (DMA-MS) . J. Am. Soc. Mass Spectrom. , 22 : 158 – 172 .
- Jiang , J. , Attoui , M. , Heim , M. , Brunelli , N. A. , McMurry , P. H. Kasper , G. 2011 . Transfer Functions and Penetrations of Five Differential Mobility Analyzers for Sub-2nm Particle Classification . Aerosol Sci. Technol. , 45 ( 4 ) : 480 – 492 .
- Junninen , H. , Ehn , M. , Petäjä , T. , Luosujärvi , L. , Kotiaho , T. Kostiainen , R. 2010 . A High-Resolution Mass Spectrometer to Measure Atmospheric Ion Composition . Atmos. Meas. Tech. , 3 : 1039 – 1053 .
- Kallinger , P. , Steiner , G. and Szymanksi , W. W. 2012 . Characterization of Four Different Bipolar Charging Devices for Nanoparticle Charge Conditions . J. of Nanopart. Res. , 14 : 944
- Kilpatrick , W. D. 1971 . An Experimental Mass-Mobility Relation for Ions in Air at Atmospheric Pressure . : 320 – 326 . Proc. Ann. Conf. Mass Spectrosc. 19th
- Ku , B. K. and Fernández de la Mora , J. 2009 . Relation Between Electrical Mobility, Mass, and Size for Nanodrops 1–6.5 nm in Diameter in Air . Aerosol Sci. Technol. , 43 ( 3 ) : 241 – 249 .
- Kulmala , M. , Kontkanen , J. , Junninen , H. , Lehtipalo , K. , Manninen , H. E. Nieminen , T. 2013 . Direct Observations of Atmospheric Aerosol Nucleation . Science , 339 : 943
- Kulmala , M. , Petäjä , T. , Nieminen , T. , Sipilä , M. , Manninen , H. E. Lehtipalo , K. 2012 . Measurement of the Nucleation of Atmospheric Aerosol Particles . Nature Protocols , 7 : 1651 – 1667 . doi: 10.1038/nprot.2012.091
- Larriba , C. , Hogan , C. J. , Attoui , M. , Barrajo , R. , Fernández-Garcia , J. and Fernández de la Mora , J. 2011 . The Mobility-Volume Relationship Below 3 nm Examined by Tandem Mobility-Mass Measurement . Aerosol Sci. Technol. , 45 : 453 – 467 .
- Manninen , H. E. , Franchin , A. , Schobesberger , S. , Hirsikko , A. , Hakala , J. Skromulis , A. 2011 . Characterisation of Corona-Generated Ions used in a Neutral Cluster and Air Ion Spectrometer (NAIS) . Atmos. Meas. Tech. , 4 : 2767 – 2776 . doi: 10.5194/amt-4–2767–2011
- McMurry , P. H. , Kulmala , M. and Worsnop , D. R. 2011 . Special Issue on Aerosol Measurements in the 1 nm Range . Aerosol Sci. Technol. , 45 ( 4 ) : 1 – 1 .
- Mäkelä , J. M. , Jokinen , V. , Mattila , T. , Ukkonen , A. and Keskinen , J. 1996 . Mobility Distribution of Acetone Cluster Ions . J. Aerosol Sci. , 27 ( 2 ) : 175 – 190 .
- Rosell-Llompart , J. , Loscertales , I. G. , Bingham , D. and Fernández de la Mora , J. 1996 . Sizing Nanoparticles and Ions with a Short Differential Mobility Analyzer . J. Aerosol Sci. , 27 : 695 – 719 .
- Rosser , S. and Fernández de la Mora , J. 2005 . Vienna Type DMA of High Resolution and High Flow Rate . Aerosol Sci. Technol. , 39 ( 12 ) : 1191 – 1200 .
- Steiner , G. , Attoui , M. , Wimmer , D. and Reischl , G. P. 2010 . A Medium Flow, High Resolution Vienna DMA Running in Recirculating Mode . Aerosol Sci. Technol. , 44 ( 4 ) : 308 – 315 .
- Steiner , G. and Reischl , G. P. 2012 . The Effect of Carrier Gas Contaminants on the Charging Probability of Aerosols Under Bipolar Charging Conditions . J. Aerosol Sci. , 54 : 21 – 31 .
- Tammet , H. 1995 . Size and Mobility of Nanometer Particles, Clusters and Ions . J. Aerosol Sci. , 26 ( 3 ) : 459 – 475 .
- Tammet , H. 1998 . Reduction of Air Ion Mobility to Standard Conditions . J. Geophys. Res. , 103(D12):13,933–13,937.
- Wiedensohler , A. , Birmili , W. , Nowak , A. , Sonntag , A. , Weinhold , K. Merkel , M. 2012 . Mobility Particle Size Spectrometers: Harmonization of Technical Standards and Data Structure to Facilitate High Quality Long-Term Observations of Atmospheric Particle Number Size Distributions . Atmos. Meas. Tech. , 5 ( 3 ) : 657 – 685 .
- Winklmayr , W. , Reischl , G. P. , Lindner , A. O. and Berner , A. 1991 . A New Electromobility Spectrometer for the Measurement of Aeorosol Size Distribution in the Size Range from 1 to 1000 nm . J. Aerosol Sci. , 22 : 289