Abstract
Bioaerosols, such as bacterial and fungal cells and their spores, are components of indoor airborne particulate matter and have been associated with human health problems as well as various environmental issues. Natural antimicrobial products have been used in air filters for bioaerosol control. However, natural products may lose some function due to their sensitivity to environmental factors such as temperature and humidity. In this study, we investigated the effects of temperature on antimicrobial fiber filters coated with nanoparticles of a natural product, namely, Sophora flavescens extract. Inactivation efficiency decreased with increasing temperature and treatment time. A quantitative chemical analysis of the filters revealed that the quantities of antimicrobial compounds decreased noticeably, with a consequent decrease in antimicrobial activity. In addition, the S. flavescens nanoparticles on the filter fiber surface melted gradually as treatment time increased at temperatures >100°C. This change in nanoparticle morphology in turn affected the pressure and filtration efficiency of filters, both of which decreased with increasing temperature and treatment time. These results could provide a scientific basis for the improvement of indoor air-quality control using antimicrobial air filters coated with S. flavescens nanoparticles.
Copyright 2014 American Association for Aerosol Research
INTRODUCTION
Bioaerosols are usually defined as aerosols or particulate matter of microbial, plant, or animal origin, and the term is often used synonymously with organic dust (Douwes et al. Citation2003; Mandal and Brandl Citation2011; Després et al. Citation2012). They may also consist of live or dead pathogenic or nonpathogenic microorganisms such as bacteria, fungi, and viruses (Lee Citation2011; Moon et al. Citation2012). Bioaerosols can impact humans and cause diseases such as bronchitis, necrosis, and skin diseases. Research on bioaerosols has progressed continually, in particular due to concerns about nosocomial and contact infections with avian influenza and swine flu viruses (Douwes et al. Citation2003).
Various techniques for controlling bioaerosols, including ultraviolet germicidal irradiation (Lin and Li Citation2002; Walker and Ko Citation2007), air electric ion emission (Grinshpun et al. Citation2005), thermal treatment (Jung et al. Citation2009a; Jung et al. Citation2009b; Hwang et al. Citation2010), and air filtration, have been developed. Air filtration is the most widely used method for removing bioaerosols and maintaining indoor air quality (IAQ) (Price et al. Citation1994). However, after bioaerosols accumulate on the surfaces of filters, microorganisms can multiply under appropriate conditions, in particular high moisture levels. Moreover, organic material deposited on the filter medium may provide nutrients and contribute to microbial growth, which not only contaminates the filter but also reduces its efficacy.
Antimicrobial air filters have notable advantages, as they rapidly inactivate captured microorganisms and minimize the number of live/viable particles deposited on the filter by passing air (Pyankov et al. Citation2008). Air filtration using antimicrobial materials such as silver (Ag) and copper (Cu) nanoparticles, carbon nanotubes (CNT), and biocidal chemicals is considered practical and effective and can disrupt bacterial membranes and remove bacterial bioaerosols (Jung et al. Citation2011a). Ag and Cu nanoparticles are effective inactivators of bacteria (Yoon et al. Citation2007), and single-walled CNT can be used as absorbent media to eliminate pathogenic bacteria from contaminated water (Upadhyayula et al. Citation2009). However, nanoparticles that are introduced into the body through breathing or via the skin, can freely penetrate the cell membrane. Due to this phenomenon, nanoparticles can affect other organs such as the lungs and heart, penetrate to the brain, be delivered to the fetus, and pose a risk of destroying DNA. Also, nanoparticles can persist for a long time in the environment and may bioaccumulate as precisely purified particles (Lin et al. Citation2006; Sharma et al. Citation2009; Hackenberg et al. Citation2011).
Research related to IAQ has examined alternative antimicrobial materials with lower toxicity to humans. In an effort to identify efficient antimicrobial materials, many researchers have investigated natural products for potential use in air filters and have demonstrated that some natural products suppress the growth and reproduction of, or are lethal to, microorganisms (Kuroyanagi et al. Citation1999; Donaldson et al. Citation2005; Pibiri et al. Citation2006). Natural antimicrobial products such as Melaleuca alternifolia (tea tree oil) and Eucalyptus oil can be used as a coating for filters to inactivate fungal spores, bacteria, and influenza viruses (Pyankov et al. Citation2008; Huang et al. Citation2010; Pyankov et al. Citation2012). Lee et al. (Citation2013) used Mukdenia rossil (olive) Koidz to create an antimicrobial air filter (Lee et al. Citation2013). Jung et al. (2011b) used filters coated with Sophora flavescens nanoparticles and a nebulization-thermal drying process to evaluate their inactivation efficiency toward Staphylococcus epidermidis, Bacillus subtilis, and Escherichia coli (E. coli) (Jung et al. Citation2011b).
However, although natural products are safe antimicrobial substances, they are sensitive to environmental conditions such as temperature and relative humidity. An antimicrobial air filter can be used in various indoor facilities, such as schools, offices, and homes, for air purification. Also, from the manufacturing stage to product storage or usage, antimicrobial air filters can be exposed to various environmental conditions. In this regard, several studies have been conducted to assess the efficacy of natural nanoparticles under various environmental conditions. Chong et al. (Citation2013) evaluated the antimicrobial durability of air filters coated with S. flavescens nanoparticles over a 5-month period at ∼20°C and 20% relative humidity, and found that the levels of antimicrobial compounds in the nanoparticles decreased with time (Chong et al. Citation2013). Hwang et al. (Citation2012) studied the short-term effects of circulation of humid air on antimicrobial filters coated with S. flavescens nanoparticles. High relative humidity induced morphological and coalescence changes in the nanoparticles with a concomitant reduction in the rate of bacterial inactivation (Hwang et al. Citation2012). However, the effects of the surrounding temperature on antimicrobial filters coated with natural-product nanoparticles have not been reported to the best of our knowledge.
In this study, we investigated the changes in the various characteristics of the antimicrobial filter coated with S. flavescens natural-product nanoparticles under various temperatures (20, 50, 75, 100, and 125°C) and treatment times (0, 1, 6, 19, and 24 h).
MATERIALS AND METHODS
Preparation of Antimicrobial S. flavescens Extract
Extract of S. flavescens, which is commonly used in treatments for viral hepatitis, enteritis, cancer, viral myocarditis, gastrointestinal hemorrhage, and skin diseases (De Naeyer et al. Citation2004), was used as a natural test product. In particular, this extract has great commercial value as a natural antimicrobial material due to both an abundant supply and high antimicrobial activity. Dried roots of S. flavescens were purchased from a Kyungdong oriental herbal market in Seoul, Korea. The voucher specimen was stored at the Functional Food Center, KIST Gangneung Institute, Korea. Dried roots of S. flavescens (600 g) were extracted three times with ethanol (1 L) (111,727; 99.9% purity, Merck KGaA, Darmstadt, Germany) by refluxing for 3 h. After filtration, the ethanol extract was evaporated in vacuo and freeze-dried. To prepare an antimicrobial filter using the extract, 0.25-g S. flavescens powder was suspended in 40 mL ethanol and sonicated for 10 min. The suspension was filtered through a cellulose acetate membrane filter (0.24-μm pore size) to eliminate insoluble residue.
Preparation of Natural-Product Nanoparticle Coated Filters
The nanosized natural-product particles can display greater antimicrobial activity than the bulk natural-product because their specific surface area for contact with surrounding antigens can be maximized by decreasing their size. In our previous study (Jung et al. Citation2011b), we reported the characteristics of nanoparticles generated from natural-product extracts using a nebulization-thermal drying method and its applications for antimicrobial air filtration. In the gas phase process, the nebulization-thermal drying method for natural-product nanoparticle generation allows pure, steady and continuous particle generation, which is favorable for the mass production of nanoparticles used in antimicrobial air filters.
The S. flavescens suspension was loaded into a six-jet Collison nebulizer (BGI Inc., Waltham, MA, USA), and was sprayed as an ethanol extract (). The droplets were passed through both an activated carbon absorber tube and a thermal heating tube (75°C) (∼3 s) to remove the ethanol. Details of this drying process have been reported previously (Jung et al. Citation2009b; Jung et al. Citation2011b). Finally, the nebulization—thermal drying process produced natural-product nanoparticles with a broad size distribution, ranging from a few to several hundred nanometers. According to Jung et al. (2011b), the chemical composition is unchanged when S. flavescens extract is converted into nanoparticles by nebulization and rapid thermal-drying (Jung et al. Citation2011b).
FIG. 1 Schematic of the experimental setup. (a) The nebulization—thermal drying process (solid line) and filtration test (dotted line), (b) outline of the experimental procedure.
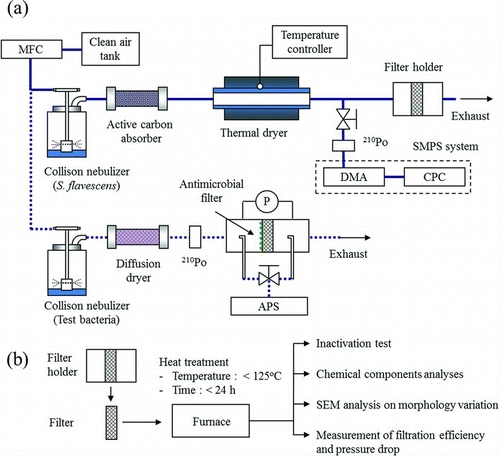
The log-normal particle size distribution was measured using a scanning mobility particle size system (SMPS) consisting of a differential mobility analyzer (DMA 3081, TSI Inc., Shoreview, MN, USA) and an ultra-condensation particle counter (CPC 3025; TSI Inc.) (Wang and Flagan Citation1990). The particles had a peak diameter of 117.6 nm, a geometric mean diameter (GMD) of 125.1 nm, and a geometric standard deviation (GSD) of 1.6. In addition, our previous study showed that the morphology of these nanoparticles was mostly noncoalesced and spherical (Hwang et al. Citation2012). The generated nanoparticles were deposited continuously onto a polypropylene resin fiber filter with a fiber diameter of 10–20 μm and thickness of 0.45 mm. The nanoparticle concentration deposited on the filter per unit cross-sectional filter surface area was ∼5.2 × 1010 particles/cm2 filter (∼90 g/m2 filter). In this study, we experimented all processes of nanoparticle generation and particle deposition onto filters in a clean bench environment to prevent exposure to the external environment. Additionally, we investigated whether the natural-product nanoparticles remained attached to the filter under continuous-flow conditions, because they can become a secondary pollutant if they detach from the filter. The clean and filtered air was passed through the antimicrobial filter for 1 h. During this process, we measured the concentrations of natural nanoparticles at the filter outlet but detected only low levels (<1 particle/cm3). All filters were stored in a diffusion dryer box packed with silica beads for 24 h at room temperature.
Behavior of Antimicrobial Filters at Different Temperatures
To investigate the effects of temperature on the filters, they were exposed to various temperatures (20, 50, 75, 100, and 125°C) and treatment times (0, 1, 6, 19, and 24 h) to identify significant physical/chemical changes in the natural-product nanoparticles on air filter. The temperature range of 75–125°C does not represent actual operating conditions for the air purification system because indoor air temperature is generally controlled within a limited range. These conditions were selected to identify significant changes of natural-product nanoparticles on air filters at temperature extremes. Temperature was controlled using a thermal electric furnace, and the internal temperature was maintained at a constant level (±3%).
Generation of Bacterial Bioaerosol
The microorganism selected for this study was E. coli (ATCC 8739), which has widely been used as a test bacterial species in various fields of bioaerosol research, including bioaerosol sampling (Henningson and Ahlberg Citation1994; Li Citation1999a; Citation1999b; Lee and Kim Citation2003), control (Damar et al. Citation2002; Lin and Li Citation2002; Kowalski et al. Citation2003; Lee et al. Citation2008; Pal et al. Citation2008; Simon et al. Citation2011; King and McFarland Citation2012), and detection (Speight et al. Citation1997; Pinnick et al. Citation1998; Hernandez Citation1999; Pinnick et al. Citation1999; Xu and Yao Citation2013). As representative Gram-negative bacteria, their biophysical characteristics and genomic information are well-defined in the literature. Almost all airborne E. coli are sensitive to adverse environmental conditions. In particular, pathogenic E. coli O157:H7 are hazardous to humans and can be spread in an airborne manner (Varma et al. Citation2003). E. coli cultures were grown in tryptic soy broth (Becton Dickinson, Franklin Lakes, NJ, USA) at 37°C for 24 h. Stationary-phase organisms were harvested by centrifugation (5000 × g, 10 min). Pellets of test bacteria were carefully washed three times with sterilized deionized water (SDW) and subsequently diluted to obtain the final bacterial suspension to generate a bioaerosol. A 30 mL aliquot at a concentration of approximately 5 × 107 colony forming units (CFU)/mL was placed in a six-jet Collison nebulizer (BGI) with a pressure of 12.5 psi. Using the nebulizer, the bacteria were aerosolized at 5 L/min in the dry and filtered airstream. The dispersed bioaerosol was passed through the diffusion dryer to remove moisture and through a 210Po neutralizer to reduce the electrical charge of the bioaerosol. Then the bacteria were introduced into the filter holder where a prepared antimicrobial filter was installed. The size distribution and concentration of airborne bacteria were analyzed using an aerodynamic particle sizer (APS; 3321, TSI Inc.), which sizes airborne particles in the range 0.5–20 μm using a time-of-flight technique by measuring the aerodynamic diameter. The peak particle concentrations of the test bacteria bioaerosols were clearly recognizable with an aerodynamic mode diameter of approximately 0.84 μm. The GMD and GSD were 0.9 ± 0.1 μm and 1.3 ± 0.1, respectively. The total concentration deposited onto the antimicrobial filter was approximately 1.12 × 107 particles/cm2 filter. We evaluated the performance of the filter via an antimicrobial test in which we measured filtration efficiency and the change in pressure drop.
Measurement of Pressure Drop Changes and Filtration Efficiencies
To investigate the filtration efficiencies of the antimicrobial filters, the concentration of bacterial bioaerosol at the filter inlet (C inlet) and outlet (C outlet) was measured using an APS. The filtration efficiency (η) was defined as:
The measurement was conducted for 5 min at 25°C and 25% relative humidity. The pressure drop between the filter inlet and outlet was determined under various face velocities (3.3–7.2 cm/s) using a micromanometer (FCO12; Furness Controls Ltd., Bexhill, UK).
Antimicrobial Tests
Antimicrobial tests were conducted after treating the filters at various temperatures. Bioaerosols were deposited continuously onto filters (in filter holders) during a period of approximately 5 min in all experiments to obtain a similar loading on all filters (about 1.12 × 107 particles/cm2 filter). Each filter was removed from the filter holder immediately after bioaerosol sampling and placed into a Petri dish for 1 min. Then, each filter was soaked in a 50 mL conical tube containing 5 mL (V extraction) phosphate-buffered saline (pH 7.4) with 0.05% Tween 80. Samples in extraction fluid were vortexed for 2 min. Samples in solution then underwent an agitation step in an ultrasonic bath with ice for 10 min. The bacterial suspension was serially diluted and plated onto nutrient agar (NA, Becton Dickinson), followed by incubation at 37°C for 24 h. Colonies that grew on the plate were counted. A relative microbial survival (RMS) was calculated using the following equations:
Chemical Analysis of S. flavescens Nanoparticles
To confirm the effects of temperature on the chemical composition of S. flavescens nanoparticles, filters treated to different temperatures were extracted three times with pure ethanol. The major antimicrobial components of the S. flavescens extract were kurarinone, sophoraflavanone G, and kuraridin, as described previously (Kuroyanagi et al. Citation1999; Kim et al. Citation2003; Jung et al. Citation2011b).
Each filter was extracted in ethanol (2 mL) for 1 min using an ultrasonic cleaning bath (Model RK 158s, Bandelin), and the ethanol extract was filtered and evaporated. Each extract was redissolved with ethanol (1 mL) and analyzed by high-performance liquid chromatography (HPLC). The HPLC system was equipped with an Agilent Series 1200 liquid chromatography system, vacuum degasser, binary pump, autosampler, column oven, and diode array detection detectors (Agilent, Waldbronn, Germany). A Shiseido Capcell Pak MGII column (4.6 × 250 mm, 5 μm, Shiseido, Tokyo, Japan) was used. The gradient profile was as follows: 0–10 min, initial mobile phase 0.1% trifluoroacetic acid in acetonitrile/water (20:80,% v/v); 10–40 min, linear gradient 20:80; 40–45 min, isocratic 80:20 and reconditioning steps to the initial condition for 15 min. Chromatograms were recorded at 254 and 280 nm. The sample injection volume was 20 μL, and the flow rate was 1 mL/min.
Thermogravimetric Analysis
Thermogravimetric analysis (TGA, TA Instruments Q500) was used to measure changes in the mass of S. flavescens extract powder with increasing temperature. A TGA curve was generated using a heating rate of 1°C/min under constant airflow at 50 mL/min. Samples were heated from 20°C to 600°C.
Scanning Electron Microscopy
To observe and compare the effects of high temperature, the antimicrobial filters were coated with Pt–Pd using an ion-sputtering coater (model IB-3; Eiko Co., Ltd., Ibaraki, Japan) and visualized under high vacuum (10−5 mbar) by scanning electron microscopy (SEM; XL30 ESEM-FEG; Philips Electron Optics, Eindhoven, Netherlands).
Statistical Analysis
The data were analyzed statistically by an analysis of variance, t-test, and linear regression using the SAS software (ver. 9.1 for Microsoft Windows).
RESULTS
Effects of Temperature on Antimicrobial Activity
shows the inactivation of E. coli bacterial bioaerosols by the antimicrobial filters. The inactivation rates at 20°C (or at 0 h of each temperature condition) were ∼97–98%, and were maintained during a 24 h period. However, the antibacterial activity decreased rapidly with increasing treatment time at temperatures >75°C. At 125°C, inactivation decreased to 65.4 ± 7.61% at 1 h, 51.6 ± 9.45% at 6 h, and 5.7 ± 5.80% at 24 h (p < 0.05).
Effect of Temperature on the Chemical Composition of the Antimicrobial Filters
shows the levels of the major antimicrobial chemicals (kurarinone, kuraridin, and sophoraflavanone G) in the nanoparticles on the antimicrobial filters. The levels in filters treated at 50°C for 1, 6, and 20 h were similar to those in filters at room temperature (20°C). However, the chemical composition changed dramatically at >100°C. At 100°C, the concentration of kurarinone decreased sharply with increasing treatment time, whereas that of kuraridin increased and then started to decrease after 6 h. The sophoraflavanone G level decreased slightly compared to that of the other chemical components. Despite the same treatment time, both kurarinone and sophoraflavanone G increased more rapidly at 125°C than at 100°C. Moreover, kuraridin concentration increased at 125°C and remained constant after 6 h; this concentration was similar to that observed at 100°C after 24 h. These results suggest that degradation of major antimicrobial compounds may be the cause of the decreased bacterial inactivation as a function of time and temperature.
Thermogravimetric Analysis of S. flavescens Powder
shows the relative TGA curve for the S. flavescens extract powder. This curve represents the entire vapor flux of a solid sample at a heating rate of 1°C/min (Jung et al. Citation2010). The mass loss of S. flavescens extract powder increased to approximately 2.01% at 75°C, 2.74% at 100°C, and 3.48% at 125°C.
Effects of Temperature on Nanoparticle Morphology
The effects of temperature and treatment time on the surface morphologies of the antibacterial filters were evaluated. shows SEM micrographs of antimicrobial filters exposed to 20°C (a) and 50°C for 1 h (b) and 24 h (c), and 100°C for 5 min (d), 10 min (e) and 30 min (f). The morphology of the pristine filter was retained, after treatment at 125°C for 24 h (data not shown). However, S. flavescens nanoparticles on the filter were very finely melted at 50°C. In addition, particles at the surface changed gradually, and this effect increased at 100°C (). According to Hwang et al. (Citation2012), morphological changes in S. flavescens nanoparticles on a filter surface can the affect antimicrobial activity of the filter (Hwang et al. Citation2012) In this case, only dissolved in surface changes were observed, as shown in .
Effect of Temperature on Filtration Performance
As shown in , the pressure drop across the antimicrobial filters was measured after treatment at the different temperatures. The pressure drops increased as face velocity increased. The pressure drop across antimicrobial filters was about sevenfold higher than across pristine filters for all face velocities (p < 0.05). However, at a 7.13 cm/s face velocity, the pressure drop of antimicrobial filter after 1-h heat treatment was ∼4.9-fold lower than that of the antimicrobial filter without heat treatment, and under identical conditions, the pressure drop across the antimicrobial filter treated at elevated temperatures for 24 h was 5.3-fold lower than that of the antimicrobial filter without heat treatment. Therefore, the pressure drop of filters exposed to elevated temperatures was similar to that of pristine filters (1.04 to 2.7 mm H2O).
FIG. 6 Variation in filtration performance of antimicrobial filters exposed to 100°C for various times (n = 3). (a) Pressure drop, and (b) filtration efficiency.
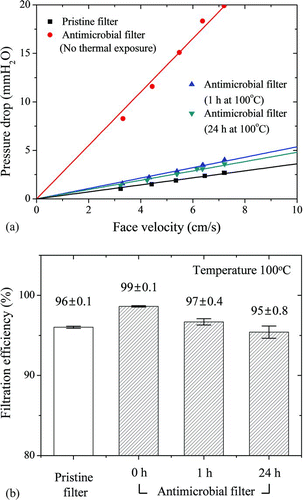
E. coli bioaerosols were used to assess filtration efficiency under the experimental conditions. The filtration efficiency of a pristine filter (96 ± 0.1%) was 3% lower than that of an antimicrobial filter without thermal treatment (99 ± 0.1%) (p < 0.05). However, the filtration efficiency of the antimicrobial filter decreased over time at 100°C. For antimicrobial filters, the filtration efficiencies were 96.7 ± 0.4% after 1 h and 95.4 ± 0.8% after 24 h.
DISCUSSION
shows the variation in the inactivation rate of antimicrobial filters under various conditions. The inactivation efficiency decreased gradually with increasing treatment time and temperature. Our previous study reported the natural decay of test microorganisms, such as S. epidermidis, B. subtilis, and E. coli on the control filter, at various residence times. The natural decay of E. coli decreased to ∼32% after a 5-min residence time (Jung et al. Citation2011b). From our previous results, we can predict that the survival rate of E. coli cells after about 1-min residence time would be larger than 70%. The relative inactivation rate of E. coli on antimicrobial filters () demonstrates the antimicrobial performance of natural-product nanoparticles eliminating the influence of natural decay. The treatment at elevated temperatures affected the chemical composition, thermal weight loss, and physical morphology of the S. flavescens nanoparticles (Figures –). The filtration efficiency and the pressure drop across the antimicrobial filters decreased with increasing time at 100°C. These changes were likely related to the chemical and physical properties of the S. flavescens nanoparticles.
Natural extracts of S. flavescens include a volatile flavonoid component (Kuroyanagi et al. Citation1999). Previous studies have shown that the major antimicrobial components of S. flavescens are kurarinone, kuraridin, and sophoraflavanone G (De Naeyer et al. Citation2004; Piao et al. Citation2006; Han et al. Citation2007), and antimicrobial activity is related to the quantities of these three major components (Chong et al. Citation2013). However, this chemical composition can be altered by environmental factors, particularly by temperature.
The speed of volatility of a chemical can also be affected by temperature. In the present study, as temperature and treatment time increased, the contents of both kurarinone and sophoraflavanone G decreased, whereas that of kuraridin increased and then decreased (). Kurarinone and sophoraflavanone G have almost identical structures; kurarinone is a 5-methoxyflavanone, whereas sophoraflavanone G is a 5-hydroxyflavanone (Sohn et al. Citation2004). They also have a similar spectrum of antimicrobial activity and minimum inhibitory concentrations against bacteria. In addition, the melting point of kurarinone is 115–117°C (Jeong et al. Citation2008); therefore, in the present study, we expected that the components of antimicrobial chemicals would decrease rapidly with increasing temperature (>100°C) and treatment time. Thermal energy degrades kurarinone: in one study, 25.54% and 16.0% of total kurarinone was degraded after 2 h at 80°C and at 60°C, respectively, whereas only slight changes occurred after 48 h at 25°C (Liu et al. Citation2009). Our study also shows similar results.
As shown in Figures and , antimicrobial activity decreased with increasing temperature and time, despite increased kuraridin levels. These results suggest that reduced sophoraflavanone G and kurarinone levels are more closely associated with a reduction in antimicrobial activity. This phenomenon may be explained by the chemical change from kurarinone to kuraridin at elevated temperatures. González et al. (2002) reported on the interconversion between kurarinone (flavanone) and kuraridin (chalcone); both temperature and solvent composition at the isomerization equilibrium between flavanone and chalcone led to changes in both the activation enthalpy and entropy related to the ring-opening reaction (González et al. Citation2002). Few studies have addressed the mechanism of interconversion between kurarinone and kuraridin under elevated atmospheric temperatures; therefore, more detailed studies are required.
Several studies have investigated the antimicrobial mechanism of S. flavescens (Cushnie and Lamb Citation2005). Sohn et al. (Citation2004) demonstrated that Gram-positive bacteria react specifically with kurarinone, and both Gram-positive and -negative bacteria display strong activity toward sophoraflavanone G. Both of these compounds may act by damaging the membrane and/or cell wall function (Sohn et al. Citation2004). It has also been reported that sophoraflavanone G is considered to exert an antibacterial effect by reducing the fluidity of bacterial cellular membranes (Tsuchiya and Iinuma Citation2000). Additionally, S. flavescens has a superior efficacy toward fungus such as the gray and white molds that fatally blight crops. Previous studies have also reported that kuraridin, sophoraflavanone D and sophoraisoflavanone A exhibit a good antifungal activity alongside their strong antibacterial activity (Sohn et al. Citation2004; Chen et al. Citation2005). Direct antimicrobial effects were also observed in four prenylated flavonoids (kuraridin, kurarinone, 5-methylsophoraflavanone B, and sophoraflavanone G), which were isolated from radix Sophorae flavescentis against the fungal strains Candida albicans and Saccharomyces cerevisiae (Chen et al. Citation2005).
shows the coalescence and morphological changes in S. flavescens nanoparticles treated at 100°C. The morphology of the nanoparticles changed at higher temperatures. Coalescence was caused by an adherent force originating from the surface tension of the melted nanoparticles (Riera-Franco de Sarabia et al. Citation2003). The nanoparticles melted and then coated the filter surface, leading to a reduction in antimicrobial activity due to the reduced area of contact between microorganisms and antimicrobial substances. The same occurred under high relative humidity. Hwang et al. (Hwang et al. Citation2012) demonstrated that the coagulation of S. flavescens nanoparticles on filter fiber at >90% relative humidity affected the antimicrobial activity of filters.
The pressure drop and filtration efficiency of antimicrobial filters at 20°C were higher than for pristine filters, but decreased following treatment at 100°C (). Morphological changes affect not only antimicrobial activity but also the filtration performance of an air filter. A change in the structure of a filter, such as its fiber porosity and surface roughness, causes a change in both the efficiency for a given particle size and the pressure drop (Hinds Citation1999). As shown in , the thermal environment can cause the melting and coagulation of nanoparticles deposited onto filter fibers. As the structural changes due to natural-product nanoparticles increases the porosity of an air filter, the filtration efficiency and filter pressure drop decreases (Podgórski et al. Citation2006; Boskovic et al. Citation2007).
In this study, our data suggest that antimicrobial air filters coated with S. flavescens nanoparticles maintain their antimicrobial activity under an appropriate temperature. The nanoparticles sustained antimicrobial activities >50% of the baseline at <75°C for 30 h. Antimicrobial activity was linked to temperature and treatment time at a given temperature, both of which can physically and chemically modify S. flavescens nanoparticles. The antimicrobial filters tested in the present study would be suitable for use in air cleaners, small offices, and vehicles rather than ventilation systems in large buildings; the latter require stability at a range of temperatures. Our results provide useful information for practical application of filters that incorporate natural antimicrobial products. Additionally, our study provides a method for the experimental evaluation of the variation in antimicrobial activity at specific temperatures and treatment times using different natural materials.
FUNDING
This research was supported by the National Research Foundation of Korea (NRF) funded by the Ministry of Science, ICT and Future Planning, South Korea (2013K000386).
REFERENCES
- Boskovic , L. , Agranovski , I. E. and Braddock , R. D. 2007 . Filtration of Nanosized Particles with Different Shape on Oil Coated Fibres . J. Aerosol Sci. , 38 : 1220 – 1229 .
- Chen , L. , Cheng , X. , Shi , W. , Lu , Q. , Go , V. L. Heber , D. 2005 . Inhibition of Growth of Streptococcus Mutans, Methicillin-Resistant Staphylococcus Aureus, and Vancomycin-Resistant Enterococci by Kurarinone, a Bioactive Flavonoid Isolated from Sophora Flavescens . J. Clin. Microbiol. , 43 : 3574 – 3575 .
- Chong , E. S. , Hwang , G. B. , Nho , C. W. , Kwon , B. M. , Lee , J. E. Seo , S. 2013 . Antimicrobial Durability of Air Filters Coated with Airborne Sophora Flavescens Nanoparticles . Sci. Total Environ. , 444 : 110 – 114 .
- Cushnie , T. and Lamb , A. J. 2005 . Antimicrobial Activity of Flavonoids . Int. J. Antimicrob. Ag. , 26 : 343 – 356 .
- Damar , S. , Bozoglu , F. , Hizal , M. and Bayindirli , A. 2002 . Inactivation and Injury of Escherichia coli O157: H7 and Staphylococcus Aureus by Pulsed Electric Fields . World J. Microbiol. Biotechnol. , 18 : 1 – 6 .
- De Naeyer , A. , Berghe , W. V. , Pocock , V. , Milligan , S. , Haegeman , G. and De Keukeleire , D. 2004 . Estrogenic and Anticarcinogenic Properties of Kurarinone, a Lavandulyl Flavanone from the Roots of Sophora flavescens . J. Nat. Prod. , 67 : 1829 – 1832 .
- Després , V. R. , Huffman , J. A. , Burrows , S. M. , Hoose , C. , Safatov , A. S. Buryak , G. 2012 . Primary Biological Aerosol Particles in the Atmosphere: A Review . Tellus B. , : 64 15598
- Donaldson , J. R. , Warner , S. L. , Cates , R. G. and Gary Young , D. 2005 . Assessment of Antimicrobial Activity of Fourteen Essential Oils When Using Dilution and Diffusion Methods . Pharm. Biol. , 43 : 687 – 695 .
- Douwes , J. , Thorne , P. , Pearce , N. and Heederik , D. 2003 . Bioaerosol Health Effects and Exposure Assessment: Progress and Prospects . Ann. Occup. Hyg. , 47 : 187 – 200 .
- González , E. A. , Nazareno , M. A. and Borsarelli , C. D. 2002 . Enthalpy–Entropy Compensation Effect in the Chalcone Formation from Naringin in Water–Ethanol Mixtures . J. Chem. Soc., Perkin Trans , 2 : 2052 – 2056 .
- Grinshpun , S. , Mainelis , G. , Trunov , M. , Adhikari , A. , Reponen , T. and Willeke , K. 2005 . Evaluation of Ionic Air Purifiers for Reducing Aerosol Exposure in Confined Indoor Spaces . Indoor Air , 15 : 235 – 245 .
- Hackenberg , S. , Scherzed , A. , Kessler , M. , Hummel , S. , Technau , A. Froelich , K. 2011 . Silver Nanoparticles: Evaluation of DNA Damage, Toxicity and Functional Impairment in Human Mesenchymal Stem Cells . Toxicol. Lett. , 201 : 27 – 33 .
- Han , J. , Sun , M. , Cui , Y. , Wang , T. , Zhang , W. Guo , M. 2007 . Kushen Flavonoids Induce Apoptosis in Tumor Cells by Inhibition of NF-κB Activation and Multiple Receptor Tyrosine Kinase Activities . Phytother. Res. , 21 : 262 – 268 .
- Henningson , E. W. and Ahlberg , M. S. 1994 . Evaluation of Microbiological Aerosol Samplers: A Review . J. Aerosol Sci. , 25 : 1459 – 1492 .
- Hernandez , M. 1999 . A Combined Fluorochrome Method for Quantitation of Metabolically Active and Inactive Airborne Bacteria . Aerosol Sci. Technol. , 30 : 145 – 160 .
- Hinds , W. C. 1999 . Aerosol Technology: Properties, Behavior, and Measurement of Airborne Particles , New York : Wiley-Interscience .
- Huang , R. , Pyankov , O. V. , Yu , B. and Agranovski , I. E. 2010 . Inactivation of Fungal Spores Collected on Fibrous Filters by Melaleuca Alternifolia (Tea Tree Oil) . Aerosol Sci. Tech. , 44 : 262 – 268 .
- Hwang , G. B. , Jung , J. H. , Jeong , T. G. and Lee , B. U. 2010 . Effect of Hybrid UV-Thermal Energy Stimuli on Inactivation of S. Epidermidis and B. Subtilis Bacterial Bioaerosols . Sci. Total Environ. , 408 : 5903 – 5909 .
- Hwang , G. B. , Lee , J. E. , Nho , C. W. , Lee , B. U. , Lee , S. J. Jung , J. H. 2012 . Short-Term Effect of Humid Airflow on Antimicrobial Air Filters Using Sophora Flavescens Nanoparticles . Sci. Total Environ , 421–422 : 273 – 279 .
- Jeong , T.-S. , Ryu , Y. B. , Kim , H. Y. , Curtis-Long , M. J. , An , S. J. Lee , J. H. 2008 . Low Density Lipoprotein (LDL)-Antioxidant Flavonoids from Roots of Sophora Flavescens . Biol. Pharm. Bull. , 31 : 2097 – 2102 .
- Jung , J. H. , Hwang , G. B. , Lee , J. E. and Bae , G. N. 2011a . Preparation of Airborne Ag/CNT Hybrid Nanoparticles Using an Aerosol Process and Their Application to Antimicrobial Air Filtration . Langmuir , 27 : 10256 – 10264 .
- Jung , J. H. , Hwang , G. B. , Park , S. Y. , Lee , J. E. , Nho , C. W. Lee , B. U. 2011b . Antimicrobial Air Filtration Using Airborne Sophora Flavescens Natural-Product Nanoparticles . Aerosol Sci. Tech. , 45 : 1510 – 1518 .
- Jung , J. H. , Lee , J. E. and Kim , S. S. 2009a . Thermal Effects on Bacterial Bioaerosols in Continuous Air Flow . Sci. Total Environ. , 407 : 301 – 317 .
- Jung , J. H. , Lee , J. E. , Kim , S. S. and Bae , G. N. 2010 . Size Reduction of Aspergillus Versicolor Fungal Bioaerosols During a Thermal Heating Process in Continuous-Flow System . J. Aerosol Sci. , 41 : 602 – 610 .
- Jung , J. H. , Lee , J. E. , Lee , C. H. , Kim , S. S. and Lee , B. U. 2009b . Treatment of Fungal Bioaerosols by a High-Temperature, Short-Time Process in a Continuous-Flow System . Appl. Environ. Microbiol. , 75 : 2742 – 2749 .
- Kim , S. J. , Son , K. H. , Chang , H. W. , Kang , S. S. and Kim , H. P. 2003 . Tyrosinase Inhibitory Prenylated Flavonoids from Sophora Flavescens . Biol. Pharm. Bull. , 26 : 1348 – 1350 .
- King , M. D. and McFarland , A. R. 2012 . Bioaerosol Sampling with a Wetted Wall Cyclone: Cell Culturability and DNA Integrity of Escherichia coli Bacteria . Aerosol Sci. Technol. , 46 : 82 – 93 .
- Kowalski , W. , Bahnfleth , W. , Striebig , B. and Whittam , T. 2003 . Demonstration of a Hermetic Airborne Ozone Disinfection System: Studies on E. coli . AIHA J. , 64 : 222 – 227 .
- Kuroyanagi , M. , Arakawa , T. , Hirayama , Y. and Hayashi , T. 1999 . Antibacterial and Antiandrogen Flavonoids from Sophora Flavescens . J. Nat. Prod. , 62 : 1595 – 1599 .
- Lee , B. , Yun , S. , Ji , J. and Bae , G. 2008 . Inactivation of S. epidermidis, B. subtilis, and E. coli Bacteria Bioaerosols Deposited on a Filter Utilizing Airborne Silver Nanoparticles . J. Microbiol. Biotechnol. , 18 : 176 – 182 .
- Lee , B. U. 2011 . Life Comes from the Air: A Short Review on Bioaerosol Control . Aerosol Air Quality Res. , 11 : 921 – 927 .
- Lee , B. U. and Kim , S. S. 2003 . Sampling E. coli and B. subtilis Bacteria Bioaerosols by a New Type of Impactor with a Cooled Impaction Plate . J. Aerosol Sci. , 34 : 1097 – 1100 .
- Lee , D. H. , Jung , J. H. and Lee , B. U. 2013 . Effect of Treatment with a Natural Extract of Mukdenia Rossii (Oliv) Koidz and Unipolar Ion Emission on the Antibacterial Performance of Air Filters . Aerosol Air Qual. Res. , 13 : 771 – 776 .
- Li , C.-S. 1999a . Evaluation of Microbial Samplers for Bacterial Microorganisms . Aerosol Sci. Technol. , 30 : 100 – 108 .
- Li , C.-S. 1999b . Sampling Performance of Impactors for Bacterial Bioaerosols . Aerosol Sci. Technol. , 30 : 280 – 287 .
- Lin , C.-Y. and Li , C.-S. 2002 . Control Effectiveness of Ultraviolet Germicidal Irradiation on Bioaerosols . Aerosol Sci. Technol. , 36 : 474 – 478 .
- Lin , W. , Huang , Y.-w. , Zhou , X.-D. and Ma , Y. 2006 . In vitro Toxicity of Silica Nanoparticles in Human Lung Cancer Cells . Toxicol. Appl. Pharm. , 217 : 252 – 259 .
- Liu , P. , Deng , T. , Ye , C. , Qin , Z. , Hou , X. and Wang , J. 2009 . Identification of Kurarinone by LC/MS and Investigation of its Thermal Stability . J. Chil. Chem. Soc. , 54 : 80 – 82 .
- Mandal , J. and Brandl , H. 2011 . Bioaerosols in Indoor Environment-A Review with Special Reference to Residential and Occupational Locations . Open Environ. Biol. Monit. J. , 4 : 83 – 96 .
- Moon , H.-S. , Lee , J.-H. , Kwon , K. and Jung , H.-I. 2012 . Review of Recent Progress in Micro-Systems for the Detection and Analysis of Airborne Microorganisms . Anal. Lett. , 45 : 113 – 129 .
- Pal , A. , Pehkonen , S. O. , Yu , L. E. and Ray , M. B. 2008 . Photocatalytic Inactivation of Airborne Bacteria in a Continuous-Flow Reactor . Ind. Eng. Chem. Res. , 47 : 7580 – 7585 .
- Piao , X.-L. , Piao , X. S. , Kim , S. W. , Park , J. H. , Kim , H. Y. and Cai , S.-Q. 2006 . Identification and Characterization of Antioxidants from Sophora Flavescens . Biol. Pharm. Bull. , 29 : 1911 – 1915 .
- Pibiri , M. , Goel , A. , Vahekeni , N. and Roulet , C. 2006 . Indoor Air Purification and Ventilation Systems Sanitation with Essential Oils . Int. J. Aromatherapy , 16 : 149 – 153 .
- Pinnick , R. G. , Hill , S. C. , Nachman , P. , Videen , G. , Chen , G. and Chang , R. K. 1998 . Aerosol Fluorescence Spectrum Analyzer for Rapid Measurement of Single Micrometer-Sized Airborne Biological Particles . Aerosol Sci. Technol. , 28 : 95 – 104 .
- Pinnick , R. G. , Hill , S. C. , Niles , S. , Garvey , D. M. , Pan , Y. L. Holler , S. 1999 . Real-Time Measurement of Fluorescence Spectra from Single Airborne Biological Particles . Field Anal. Chem. Technol. , 3 : 221 – 239 .
- Podgórski , A. , Bałazy , A. and Gradoń , L. 2006 . Application of Nanofibers to Improve the Filtration Efficiency of the most Penetrating Aerosol Particles in Fibrous Filters . Chem. Eng. Sci. , 61 : 6804 – 6815 .
- Price , D. , Simmons , R. , Ezeonu , I. , Crow , S. and Ahearn , D. 1994 . Colonization of Fiberglass Insulation used in Heating, Ventilation and Air Conditioning Systems . J. Ind. Microbiol. Biot. , 13 : 154 – 158 .
- Pyankov , O. V. , Agranovski , I. E. , Huang , R. and Mullins , B. J. 2008 . Removal of Biological Aerosols by Oil Coated Filters . CLEAN–Soil, Air, Water , 36 : 609 – 614 .
- Pyankov , O. V. , Usachev , E. V. , Pyankova , O. and Agranovski , I. E. 2012 . Inactivation of Airborne Influenza Virus by Tea Tree and Eucalyptus Oils . Aerosol Sci. Technol. , 46 : 1295 – 1302 .
- Riera-Franco de Sarabia , E. , Elvira-Segura , L. , Gonzalez-Gomez , I. , Rodríguez-Maroto , J. , Munoz-Bueno , R. and Dorronsoro-Areal , J. 2003 . Investigation of the Influence of Humidity on the Ultrasonic Agglomeration of Submicron Particles in Diesel Exhausts . Ultrasonics , 41 : 277 – 281 .
- Sharma , V. , Shukla , R. K. , Saxena , N. , Parmar , D. , Das , M. and Dhawan , A. 2009 . DNA Damaging Potential of Zinc Oxide Nanoparticles in Human Epidermal Cells . Toxicol. Lett. , 185 : 211 – 218 .
- Simon , X. , Duquenne , P. , Koehler , V. , Piernot , C. , Coulais , C. and Faure , M. 2011 . Aerosolisation of Escherichia coli and Associated Endotoxin Using an Improved Bubbling Bioaerosol Generator . J. Aerosol Sci. , 42 : 517 – 531 .
- Sohn , H. , Son , K. , Kwon , C. , Kwon , G. and Kang , S. 2004 . Antimicrobial and Cytotoxic Activity of 18 Prenylated Flavonoids Isolated from Medicinal Plants: Morus alba L., Morus Mongolica Schneider, Broussnetia Papyrifera (L.) Vent, Sophora Flavescens Ait and Echinosophora Koreensis Nakai . Phytomedicine , 11 : 666 – 672 .
- Speight , S. , Hallis , B. , Bennett , A. and Benbough , J. 1997 . Enzyme-Linked Immunosorbent Assay for the Detection of Airborne Microorganisms Used in Biotechnology . J. Aerosol Sci. , 28 : 483 – 492 .
- Tsuchiya , H. and Iinuma , M. 2000 . Reduction of Membrane Fluidity by Antibacterial Sophoraflavanone G Isolated from Sophora Exigua . Phytomedicine , 7 : 161 – 165 .
- Upadhyayula , V. K. , Deng , S. , Smith , G. B. and Mitchell , M. C. 2009 . Adsorption of Bacillus Subtilis on Single-Walled Carbon Nanotube Aggregates, Activated Carbon and NanoCeram(TM) . Water Res. , 43 : 148 – 156 .
- Varma , J. K. , Greene , K. D. , Reller , M. E. , DeLong , S. M. , Trottier , J. Nowicki , S. F. 2003 . An Outbreak of Escherichia coli O157 Infection Following Exposure to a Contaminated Building . JAMA , 290 : 2709 – 2712 .
- Walker , C. M. and Ko , G. 2007 . Effect of Ultraviolet Germicidal Irradiation on Viral Aerosols . Environ. Sci. Technol. , 41 : 5460 – 5465 .
- Wang , S. C. and Flagan , R. C. 1990 . Scanning Electrical Mobility Spectrometer . Aerosol Sci. Technol. , 13 : 230 – 240 .
- Xu , S. , and Yao , M. 2013 . NanoPCR Detection of Bacterial Aerosols . J. Aerosol Sci. , 65 : 1 – 9 .
- Yoon , K. Y. , Jeong , H. B. , Park , J. H. and Hwang , J. H. 2007 . Susceptibility Constants of Escherichia coli and Bacillus Subtilis to Silver and Copper Nanoparticles . Sci. Total Environ. , 373 : 572 – 575 .