Abstract
Using the nasal route to deliver pharmaceutical aerosols to the lungs has a number of advantages, including coadministration during noninvasive ventilation. The objective of this study was to evaluate the growth and deposition characteristics of nasally administered aerosol throughout the conducting airways based on delivery with streamlined interfaces implementing two forms of controlled condensational growth technology. Characteristic conducting airways were considered including a nose-mouth-throat (NMT) geometry, complete upper tracheobronchial (TB) model through the third bifurcation (B3), and stochastic individual path (SIP) model to the terminal bronchioles (B15). Previously developed streamlined nasal cannula interfaces were used for the delivery of submicrometer particles using either enhanced condensational growth (ECG) or excipient enhanced growth (EEG) techniques. Computational fluid dynamics (CFD) simulations predicted aerosol transport, growth, and deposition for a control (4.7 μm) and three submicrometer condensational aerosols with budesonide as a model insoluble drug. Depositional losses with condensational aerosols in the cannula and NMT were less than 5% of the initial dose, which represents an order-of-magnitude reduction compared to the control. The condensational growth techniques increased the TB dose by a factor of 1.1–2.6×, delivered at least 70% of the dose to the alveolar region, and produced final aerosol sizes ≥2.5 μm. Compared to multiple commercial orally inhaled products, the nose-to-lung delivery approach increased dose to the biologically important lower TB region by factors as large as 35×. In conclusion, nose-to-lung delivery with streamlined nasal cannulas and condensational aerosols was highly efficient and targeted deposition to the lower TB and alveolar regions.
Copyright 2014 American Association for Aerosol Research
INTRODUCTION
Pharmaceutical aerosols are typically delivered to the lungs through oral inhalation using metered dose inhalers (MDIs), dry powder inhalers (DPIs), nebulizers, and softmist inhalers (Finlay Citation2001; Newman Citation2009). Compared with oral inhalation, the administration of pharmaceutical aerosols through the nose to the lungs is advantageous in a number of situations (Bhashyam et al. Citation2008; Longest et al. Citation2011; Rubin Citation2011; Dhand Citation2012). Nose-to-lung aerosol delivery may improve patient comfort and compliance if a simple cannula can be used for medications with long delivery times and frequent dosing regimes. Considering patients receiving noninvasive ventilation (NIV), nasal interfaces are often employed for gas delivery with techniques that include nasal high-flow therapy (HFT), low-flow therapy (LFT), and nasal continuous positive airway pressure (NCPAP) (Hess Citation2007; Dhand Citation2012). Delivering inhaled medications through the gas administration interface of NIV systems allows for concurrent therapy and prevents interruption of gas delivery during aerosol administration (Dhand Citation2007, Citation2012; Hess Citation2007; Ari and Fink Citation2012).
While a number of advantages exist for nose-to-lung delivery of pharmaceutical aerosols, the nose is typically considered to be an effective particle filter (Cheng Citation2003). For conventional sized pharmaceutical aerosols (3–7 μm), depositional losses of the drug in the nose are expected to be 20% or higher at typical adult inhalation rates (Kelly et al. Citation2004a; Longest et al. Citation2011; Golshahi et al. Citation2013). Moreover, aerosol depositional losses in ventilation lines, connectors, and commercial nasal cannula interfaces are reported to be 75–98% (Bhashyam et al. Citation2008; Ari et al. Citation2011). In contrast with conventional delivery, the use of initially submicrometer aerosols and condensational growth techniques have recently been shown to provide very efficient nose-to-lung drug delivery (Longest et al. Citation2011; Golshahi et al. Citation2013).
Controlled condensational growth provides a method to nearly eliminate extrathoracic deposition, ensure lung retention of inhaled pharmaceutical aerosols, and can potentially target the region of deposition within the airways. In this approach, a submicrometer aerosol is initially inhaled (Hindle and Longest Citation2010; Longest et al. Citation2010), which has a very low deposition fraction in the extrathoracic airways (Cheng Citation2003; Xi and Longest Citation2008a,Citationb). Size increase of the aerosol in the airways is then fostered by either excipient enhanced growth (EEG) (Hindle and Longest Citation2012; Longest et al. Citation2012b) or enhanced condensational growth (ECG) (Hindle and Longest Citation2010; Longest and Hindle Citation2010). With EEG, size increase of the aerosol in the humid respiratory airways is generated by using combination particles or droplets composed of a drug and hygroscopic excipient (Hindle and Longest Citation2012; Son et al. Citation2013). In ECG delivery, the aerosol is administered with a stream of saturated water vapor a few degrees above body temperature (Tian et al. Citation2011a). Previous studies of controlled condensational growth delivery with oral administration have predicted negligible extrathoracic depositional loss (Hindle and Longest Citation2010; Longest et al. Citation2012b), significant size increase of the aerosol (Longest and Hindle Citation2010, Citation2011; Hindle and Longest Citation2012), full lung retention (Tian et al. Citation2011a), and the ability to target delivery within the airways (Tian et al. Citation2013). For example, Tian et al. (Citation2013) demonstrated that EEG delivery for an orally inhaled product could increase drug deposition in the lower tracheobronchial (TB) region by a factor of 20–30× with slow and deep inhalation compared with conventional DPIs. Using quick and deep inhalation with oral administration, 90% of the aerosol was delivered to the alveolar region with final aerosol sizes greater than 3 μm (Tian et al. Citation2013).
Controlled condensational growth was recently reported to enable the efficient delivery of aerosols to the lungs using nasal administration (Longest et al. Citation2011; Golshahi et al. Citation2013). Longest et al. (Citation2011) demonstrated the use of an ECG approach for efficient nose-to-lung delivery where separate streams of a submicrometer aerosol and warm (39°C) saturated air were delivered to individual nasal inlets. In vitro experiments and CFD simulations demonstrated nasal depositional losses of 5–15% with ECG compared with approximately 75% for a conventional 5 μm aerosol. Significant condensational growth occurred in the nasopharynx region where the septum ends and the aerosol and humidity streams were combined, resulting in an approximately 2 μm aerosol entering the lungs. Longest et al. (Citation2013a,Citationb) developed optimized cannula geometries for delivering aerosol to the lungs using ECG or EEG approaches based on a streamlining design approach that implemented CFD simulations and in vitro experiments. The optimized interfaces reduced cannula deposition fractions by a factor of 4× for conventional sized pharmaceutical aerosols (∼3.9 μm) and 3× for submicrometer particles (900 nm) compared with standard designs (Longest et al. Citation2013b). Golshahi et al. (Citation2013) considered streamlined nasal cannulas in nose-mouth-throat (NMT) models for both EEG and ECG lung aerosol delivery using in vitro experiments combined with CFD simulations. Under steady state delivery conditions, the condensational growth techniques provided <5% NMT deposition and ∼80% lung delivery of the pharmaceutical aerosol, which was 3–4× higher than with a conventional initial 5 μm droplet size.
As described, controlled condensational growth can significantly improve the lung delivery of nasally administered aerosols. Furthermore, EEG oral delivery can largely increase lower tracheobronchial (TB) or alveolar drug dose compared with conventional inhalers. While these findings are promising, a number of additional questions remain related to the development of nose-to-lung aerosol delivery. First, aerosol transport and deposition for nose-to-lung delivery needs to be evaluated throughout the conducting airways. The previous study of Golshahi et al. (Citation2013) considered aerosol transport from the nose through the mid-trachea level. A comparison of EEG and ECG delivery is also needed throughout the conducting airways to evaluate differences in regional deposition and the potential for targeted respiratory drug deposition with nasal administration. Furthermore, previous studies of nasal condensational growth delivery have implemented a single soluble drug (albuterol sulfate). Evaluation of transport and growth with an insoluble compound is needed. Finally, a comparison of nasally administered condensational aerosols with conventional oral delivery is needed in order to determine the potential benefits of nose-to-lung delivery throughout the respiratory tract.
The objective of this study is to evaluate the growth and deposition characteristics of nasally administered aerosol throughout the conducting airways based on delivery with streamlined interfaces implementing two forms of controlled condensational growth technology. The NMT extrathoracic geometry selected in this study provides a challenging delivery condition with mean adult nasal dimensions and a very thin (∼2.7 mm anterior to posterior distance) nasopharynx region. A new upper TB model through the third respiratory bifurcation (B3) is implemented which includes realistic tracheal and main bronchi curvatures. A stochastic individual path (SIP) model is evaluated extending from B4 into the left lower lobe of the lung, based on the previous work of Longest et al. (Citation2012a). Previously developed streamlined cannula interfaces are implemented for delivering both conventional size and submicrometer aerosols. For ECG and EEG delivery, the model drug is nonhygroscopic budesonide (BD) with NaCl included in some cases as the hygroscopic excipient. Accuracy of the CFD model is presented based on previous comparisons with experimental results of aerosol growth and deposition evaluated in models that were geometrically and thermodynamically identical between the simulations and experiments. Results are presented in terms of cannula depositional losses, regional NMT and TB deposition fractions, and aerosol size exiting selected airway regions. Comparisons are then described between the nose-to-lung administration results and previous predictions of oral conventional and controlled condensational growth respiratory drug delivery.
METHODS
Nasal Cannulas and Respiratory Geometries
Previously developed streamlined nasal cannulas were implemented for both EEG and ECG aerosol delivery, as illustrated in Figures and . The EEG cannula was developed by Longest et al. (Citation2013a) and is characterized by a single inlet for the combined aerosol and gas stream (). For ECG delivery (), the cannula has separate inlets for delivering the aerosol and gas saturated with water vapor to individual nostrils (Longest et al. Citation2013b). Only the cannula flow paths and not the external support structure are shown in the figure. In both geometries, the streamlined aerosol delivery pathways are characterized by smooth continuous curvatures without sudden changes in diameter and flow direction. The cannula outlet prongs have an elliptical shape (not visible in ) with dimensions of 5 × 7.5 mm and direct flow to the approximate middle of the nasal valve opening.
FIG. 1 Surface models of the interface device and conducting airway geometries including the (a) excipient enhanced growth (EEG) nasal cannula, (b) enhanced condensational growth (ECG) nasal cannula, (c) complete conducting airway model, and (d) expanded view of the stochastic individual path (SIP) model extending from the fourth bifurcation (B4) to the terminal bronchioles (B15) in the left lower (LL) lung lobe.
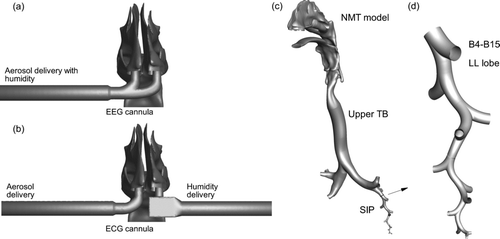
The conducting airway geometry consists of characteristic models of the NMT, upper TB airways from the trachea through bifurcation B3, and a SIP model extending into the left lower lobe of the lung (). The model represents the conducting airways of an adult male. However, a single CT scan was not available that included all regions of the conducting airways and could be used to resolve the lower TB region. Instead, a combination of previously developed characteristic models was employed to represent average adult airway conditions. The characteristic NMT model was previously described and implemented in the study of Golshahi et al. (Citation2013). Briefly, the NMT model included the nasal geometry given in Guilmette (Citation1989), which has been implemented in numerous in vitro and numerical studies (Kelly et al. Citation2004a,Citationb; Schroeter et al. Citation2006; Kimbell et al. Citation2007; Xi and Longest Citation2008b, Citation2009). Smoothing of the initial Guilmette et al. (Citation1989) data was employed to result in a physiologically realistic looking surface (Schroeter et al. Citation2011). The nasopharynx, pharynx, and larynx were developed from the anatomical CT data used in the previous study by Xi and Longest (Citation2007). The oral cavity region was also based on the previous CT data and approximates a nearly closed mouth position. Airflow is not included through the mouth opening, consistent with a subject breathing nasally with closed lips. However, the oral cavity was included in the geometry as a potential site of flow separation and recirculation. In the final NMT model, the hydraulic diameter of the glottis was 9.8 mm, which is consistent with one described in the Xi and Longest (Citation2007) study. A mouth-throat geometry with these glottal dimensions was demonstrated to accurately predict mouth-throat deposition from multiple inhalers compared with in vivo experiments (Delvadia et al. Citation2012, Citation2013).
A realistic model of the upper TB airways was employed, which was recently developed by Walenga et al. (Citation2013). The model begins at the tracheal inlet and continues through the third respiratory bifurcation (B3), with the trachea and main bronchi representing B1. Compared with a previous upper TB model (Longest et al. Citation2012c), the new geometry includes additional physiologically realistic features such as a representative coronal angle, tracheal and main bronchi curvatures, and noncircular bronchi cross-sections as described by Walenga et al. (Citation2013). The study by Walenga et al. (Citation2013) demonstrated that these features affect the deposition of monodisperse and realistic pharmaceutical aerosols in the upper TB airways. The upper TB model was based on a CT scan of a healthy 50-year-old male with a height of approximately 178 cm. As described by Walenga et al. (Citation2013), this scan was selected to represent average airway anatomy of an adult male based on comparisons to a database of upper airways from 21 individuals. For the upper TB model, average airway hydraulic diameters in the trachea, right main bronchus, and left main bronchus were 19.4, 14.9, and 11.6 mm, respectively.
Beginning at B3, a stochastic individual path (SIP) model was continued to the approximate end of the conducting airways, which was assumed to be B15 (terminal bronchioles; ). The SIP approach for conducting airway modeling was previously developed and described in a series of studies (Tian et al. Citation2011b; Longest et al. Citation2012a,Citationc). Using this method, one branch of each bifurcation is randomly selected for continuation and one branch is ended with an outflow boundary. In the SIP geometry of B4–B15, symmetric bifurcations were assumed with symmetric outflow and successive bifurcations were rotated at 90° around the parent longitudinal axis. Dimensions of the bifurcations were determined from the anatomical cast measurements reported by Yeh and Schum (Citation1980) for the left lower (LL) lobe scaled to a lung volume of 3.5 L. A single path into each lung lobe was previously shown to represent regional deposition in that lobe (Tian et al. Citation2011b). Furthermore, the LL lobe was previously found to approximate mean lobar lung deposition. Considering that there are five lung lobes, regional lobar deposition for the entire lung was approximated as the LL lobe value multiplied by a factor of 5.
TABLE 1 Description of the four delivery cases implementing budesonide (BD) as a model insoluble drug and sodium chloride (NaCl) as an effective hygroscopic excipient
Aerosol Delivery Conditions
Aerosol delivery conditions represented a control as well as ECG and EEG delivery, as shown in . In all cases, the drug was budesonide (BD), which is practically insoluble in water, and the hygroscopic excipient was NaCl. Previous studies have experimentally shown that particles composed of insoluble drugs combined with hygroscopic excipients experienced a size increase under humidity exposure that was predictable by the correlations presented in Longest and Hindle (Citation2011, Citation2012). The hygroscopic parameter of NaCl is 77.9 kmol/m3 (Longest and Hindle Citation2011), which is the highest of the hygroscopic excipients used in controlled condensational growth studies so far. As shown in , Case 1 was considered as a control to represent nose-to-lung delivery of a similar formulation nebulized to produce a 4.7 μm mass median aerodynamic diameter (MMAD) droplet aerosol, as measured by Golshahi et al. (Citation2013). Inlet conditions for Case 1 (25°C and 100% RH) were selected to minimize evaporation of the droplets before entering the nose (). ECG delivery was evaluated with Cases 2 (drug only) and 3 (drug and hygroscopic excipient) with separate aerosol particle and humidity inlets at flow rates consistent with typical nasal HFT. In Case 3, the hygroscopic excipient was included to further enhance the droplet growth occurring from the inclusion of supersaturated air with ECG delivery. Case 4 represents EEG delivery using combination particles of BD and NaCl with an initial 50:50 mass fraction. The control aerosol is delivered as a droplet with a low solute mass fraction (0.2%). For the controlled condensational growth cases, particles with an initial geometric diameter of 900 nm were employed. The density of the combined BD:NaCl particles at a mass fraction of 50:50 was 1380 kg/m3, and the density of BD was assumed to be 1000 kg/m3. In the numerical model for all cases, initially monodisperse droplets (Case 1) or particles (Cases 2–4) were injected at the aerosol inlet and allowed to evolve into polydisperse distributions as a result of condensational growth. Flow rates and thermodynamic conditions entering each of the cannula inlets are provided in . Aerosol temperature and humidity conditions () for Case 1 are consistent with delivery directly from a mesh nebulizer whereas conditions for Cases 2–4 are consistent with generation using a newly proposed mixture-heater device (Longest et al. Citation2013b). It is noted that EEG delivery Cases 1 and 4 employ the single inlet cannula shown in , whereas ECG delivery Cases 2 and 3 employ the two inlet cannula depicted in . Considering boundary conditions, the walls of the conducting airway model were held constant at 37°C and 100% RH. Only steady state flow was considered in this analysis.
Flow Field Solution
A CFD model was implemented that can accurately simulate local temperature and humidity fields, together with droplet trajectories, size change, and deposition within the NMT and TB model during nose-to-lung aerosol delivery. To effectively address both laminar and turbulent flow conditions, a low Reynolds number (LRN) k-ω turbulence model was selected (Wilcox Citation1998). This model has previously been well tested, and found to provide good estimates of aerosol transport and deposition in airway geometries provided that near-wall corrections are included (Longest and Vinchurkar Citation2007; Longest et al. Citation2008; Xi et al. Citation2008; Longest and Holbrook Citation2012; Longest et al. Citation2012c). To evaluate the variable temperature and RH fields in the conducting airway geometry, interconnected relations governing the transport of heat and mass (water vapor) were also included. These governing equations were presented in detail by Longest and Xi (Citation2008) and Longest et al. (Citation2007).
Particle and Droplet Transport
To address a broad range of particle and droplet sizes and to accommodate the calculation of aerosol evaporation and condensation, a Lagrangian tracking method was employed (Longest et al. Citation2004; Longest and Xi Citation2007). The Lagrangian transport equations can be expressed
Here vi and ui are the components of the droplet and local fluid velocity, xi represents components of the droplet position vector, gi denotes gravity components, and α is the ratio of mixture to droplet density ρ/ρd . The characteristic time required for a droplet to respond to changes in fluid motion, or the droplet relaxation time, is expressed as τp = Cc ρddd 2/18 μ, where Cc is the Cunningham correction factor for submicrometer aerosols based on the expression of Allen and Raabe (Citation1985), dd is the variable droplet diameter, and μ is the absolute viscosity of the continuous fluid. The pressure gradient or acceleration term for aerosols was neglected due to small values of the density ratio (Longest et al. Citation2004). The drag factor f, which represents the ratio of the drag coefficient to Stokes drag, is based on the expression of Morsi and Alexander (Citation1972). The effect of the Brownian motion on the trajectories of submicrometer particles and droplets has been included as a separate force per unit mass term at each time-step (Longest and Xi Citation2007).
To model the effects of turbulent fluctuations on particle and droplet trajectories, a random walk method was employed. The primary limitation of this eddy interaction model in conjunction with the LRN k-ω equations is that it does not account for reduced turbulent fluctuations in the wall-normal direction, which may result in an over-prediction of deposition (Matida et al. Citation2004). To better approximate turbulent effects on droplet deposition, an anisotropic turbulence model was applied where the near-wall fluctuating velocity is calculated as (Wang and James Citation1999; Matida et al. Citation2004)
In the above equations, u′ is the fluctuating component of the instantaneous velocity, n is the wall-normal coordinate, and uτ is the turbulent friction velocity (Wilcox Citation1998). The wall-normal damping function (fn ) is typically evaluated from the wall to a maximum n + value ranging from 10 to 100; otherwise it is assumed to be 1.0. In this study, fn was evaluated for n + values ranging from 0 to a maximum of 60.
The droplet evaporation and condensation model employed in this study is similar to previous approximations for salts (Ferron Citation1977; Ferron et al. Citation1988; Hinds Citation1999) and multicomponent aerosols (Li and Hopke Citation1993; Robinson and Yu Citation1998; Longest and Kleinstreuer Citation2005; Longest and Hindle Citation2010). The heat and mass transfer relations for multicomponent hygroscopic droplets in the respiratory airways were previously reported by Longest and Xi (Citation2008). This model employs a rapid mixing approach, which assumes conditions inside the droplet are constant and gradients are negligible compared with gradients in the air phase. The droplet model accounts for interdependent heat and mass transfer, which results in droplet heating during condensation and cooling during evaporation as a function of the surrounding temperature. Mass and heat fluxes at the droplet surface are modified for noncontinuum effects using the Knudsen correlation (Fuchs and Sutugin Citation1970; Finlay Citation2001). Blowing velocity effects were also evaluated (Longest and Xi Citation2008).
Considering particle and droplet properties, the densities of the multicomponent discrete elements are calculated as
In this expression, m and ρ are the masses and densities of water (), drug, and hygroscopic excipient (ex). The mass fraction of water vapor on the droplet surface is a critical variable, which is significantly influenced by both temperature and drug concentration. For a combination droplet of soluble drug and excipient, Yv,
surf is calculated as
Numerical Methods
In performing the CFD simulations, previously established best-practices were implemented to provide a high-quality solution (Longest and Holbrook Citation2012). To solve the transport governing equations, the CFD package ANSYS Fluent 12.0, (ANSYS Inc.) coupled with user-defined functions was employed. All transport equations were discretized to be at least second-order accurate in space. For the convective terms, a third-order QUICK scheme was used to interpolate values from cell centers to nodes. The diffusion terms were discretized using central differences. A segregated implicit solver was employed to evaluate the resulting linear system of equations. This solver uses the Gauss-Seidel method in conjunction with an algebraic multigrid approach. The PISO algorithm was employed to evaluate pressure-velocity coupling in the solutions. The outer iteration procedure was stopped when the global mass residual had been reduced from its original value by five orders of magnitude and when the residual-reduction-rates for both mass and momentum were sufficiently small. To ensure that a converged solution had been reached, residual and reduction rate factors were decreased by an order of magnitude and the results were compared. The stricter convergence criteria produced a negligible effect on both velocity and particle deposition fields. To improve accuracy, all calculations were performed in double precision.
The computational mesh was composed of approximately 1.1 million unstructured tetrahedral elements, 0.34 million hexahedral elements, and 0.9 million hexahedral elements in the NMT, upper TB, and SIP regions, respectively, resulting in a total of 2.34 million control volumes. Mesh construction software ICEM and Gambit 2.4 (ANSYS, Inc.) were used to build the grids. Grid density was increased near the walls in all cases and the NMT geometry included a very thin layer of near-wall wedge elements. Grid converged results based on negligible change in the velocity and water vapor concentration fields (<1%), as well as negligible differences in the total deposition fractions (<3%), were established for a mesh consisting of approximately 30% less control volumes. In order to produce convergent aerosol deposition results, 36 k initially monodisperse particles were injected at the aerosol inlet for each case considered. To improve numerical efficiency and accuracy, the SIP geometry was divided into segments B4–B7 and B8–B15. Flow fields were interpolated between the upstream outlets and the inlets of each of these sections. 90 k particles were injected at the inlet of each SIP section. The MMAD of the polydisperse distributions of the upstream outlets were used to define particle injections at the inlet of each SIP section including local droplet water and solute mass fractions, density, and hygroscopic properties. Mass median aerodynamic diameters after growth were calculated based on the midpoint diameters of a standard Andersen cascade impactor (Vinchurkar et al. Citation2009). Doubling the number of droplets considered had a negligible impact on both total and sectional deposition results for all model sections considered.
Validation Case Studies
Multiple case studies are described in the online supplementary information (SI) that illustrate the accuracy of the numerical methods employed in this study compared with identical in vitro experiments in terms of predicting both the size increase and deposition of droplets during controlled condensational growth. Briefly, previous studies have considered aerosol growth and deposition in a coiled tube geometry and models of both oral and nasal aerosol administration (Longest et al. Citation2011, Citation2012b; Longest and Hindle Citation2012; Golshahi et al. Citation2013). For example, with experiments involving orally administered submicrometer combination particles generated with a Respimat inhaler and having an initial diameter of 0.43 μm, experimentally determined and CFD predicted final MMADs were 1.53 (0.05) μm and 1.54 μm, respectively. Deposition fractions from the experiments and CFD predictions in a MT-TB model were both <1%. Additional details of this case study, other validation cases, and a discussion of two-way (Finlay Citation1998) and multiway (Nasr et al. Citation2009) coupling effects are provided in the SI.
RESULTS
Relative Humidity Conditions
Relative humidity values for the four delivery cases extending from the nasal inlet through the B15 outlet are displayed in . With Case 1, the relatively low delivery temperature of 25°C and inlet RH of 100% result in RH values near 99% for a majority of the NMT model and upper TB airways. Around B9, RH is observed to increase above 99.9% and remain constant. For ECG delivery Cases 2 and 3, supersaturated conditions with RH greater than 100% are observed in the left nostril (humidity delivery side) and a majority of the trachea and upper TB airways. A band of near 99% RH is also observed with Cases 2 and 3 in the trachea due to incomplete mixing of the flow streams in this region. Compared with the control case, ECG conditions produce higher RH values in the TB region due to the inlet conditions with values greater than 100% at B1 and continuing through the SIP model. In the nasopharynx region for Cases 2 and 3, cross-sectional averages of temperature and RH were 37°C and 100.8–101.1%, respectively, indicating supersaturation and a high potential for particle size increase. With EEG delivery (Case 4), a more gradual increase in RH is observed with values greater than 90% in the mid-tracheal level and greater than 99.9% at approximately B9.
FIG. 2 RH contours on coronal planes in the nasal model and airway midplanes for delivery conditions described in in the NMT and upper TB model with (a) Case 1, (b) Cases 2 and 3, and (c) Case 4. RH values in the LL lobe SIP model are presented for (d) Case 1, (e) Cases 2 and 3, and (f) Case 4. For ECG Cases 2 and 3, the aerosol is delivered to the right nostril and humid air is delivered to the left nostril from the perspective of the subject.
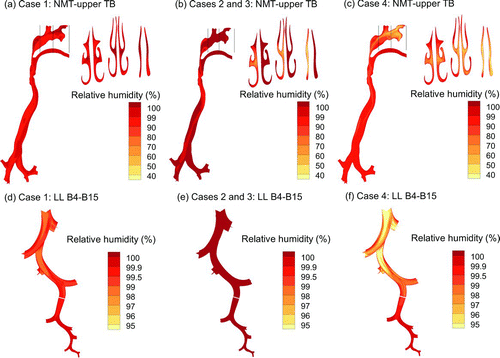
FIG. 3 Particle trajectories evaluated in the NMT and upper TB geometries contoured based on local geometric diameter for (a) Case 1: control, (b) Case 2: ECG with BD, (c) Case 3: ECG with BD:NaCl, and (d) Case 4: EEG with BD:NaCl. The calculated MMAD exiting B3 and entering the LL lobe is reported as well as a growth ratio (GR) calculated as MMADexit/(initial dgeo).
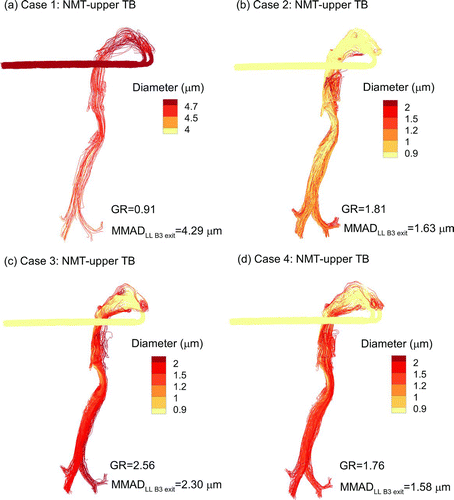
Aerosol Size
Aerosol size increase in the upper airway () and SIP model () is consistent with the RH field. For Case 1, near-saturated conditions and an initial liquid droplet with very low soluble mass fraction causes very little size-change of the aerosol in both the upper airway () and SIP geometry (). With ECG delivery of BD particles, gradual size increase is observed in the upper airways () where supersaturation is most prevalent resulting in a growth ratio (GR) of 1.81 at the B3 exit leading to the LL lobe. The GR is defined in this study as the MMAD at the exit of a region divided by the initial geometric size (900 nm) of the aerosol. Relatively slow growth is observed for Case 2 through a majority of the upper TB region and the LL lobe, resulting in an MMAD exiting B15 of 2.53 μm (). With Case 3, the growth trend is similar to Case 2, but with larger droplet diameters due to the inclusion of hygroscopic excipients (Figures and ). The Case 3 final GR is near 6.7 with a B15 exit MMAD of 6.0 μm. EEG delivery (Case 4) provides a slower and potentially more controlled aerosol growth pattern through the airways (Figures and ) with sizes of 1.58 μm and 4.8 μm at the B3 and B15 exits, respectively.
A continuous view of droplet growth trends based on MMAD is displayed in for all delivery cases. The MMAD at the exit of each bifurcation is a complex combination of droplet size growth, which increases the MMAD, and deposition, which decreases the MMAD through selective filtering. Values of MMADs exiting multiple selected regions of interest are provided in . Considering droplet sizes exiting B15 and entering the alveolar region, all cases produce MMADs greater than or equal to 2.5 μm (). Case 3 is found to provide the largest aerosol at B15, with an MMAD of 6.0 μm, due to the inclusion of both supersaturated air and a hygroscopic excipient.
FIG. 4 Particle trajectories contoured based on local geometric diameter evaluated in the LL lobe SIP model extending from B4-B15 for (a) Case 1: control, (b) Case 2: ECG with BD, (c) Case 3: ECG with BD:NaCl, and (d) Case 4: EEG with BD:NaCl. The calculated MMAD exiting B15 and entering the alveolar region is reported as well as a growth ratio (GR) calculated as MMADexit/(initial dgeo).
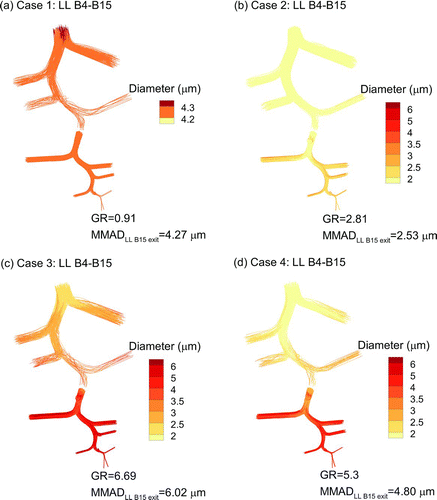
Deposition Fractions and Alveolar Delivery
Deposition fractions (DF) as a percentage of the initially delivered dose are reported for the delivery tubing and cannula, and the upper airway model in and the SIP geometry in . For the control case, total aerosol loss in the 20 cm delivery tube and cannula is approximately 42.4% with an additional 22% deposited in the NMT (). In contrast, Cases 2–4 provide cannula deposition fractions of 1.4% or below and NMT deposition below 3% (). As a result, the controlled condensational growth approach is observed to reduce combined tubing and cannula, as well as NMT, depositional losses by 1–2 orders of magnitude. Considering deposition in the LL lobe (), Case 2 is observed to increase the DF in B8-B15 by a factor of 1.3× compared with Case 1. Case 3 further increases DF throughout the SIP geometry by a factor of 2.4× compared with Case 2. Deposition fraction values for Case 4 are between those of Cases 2 and 3.
Deposition fractions in all regions are presented in graphical form in and tabulated in for regional lung delivery. Compared with the control, Cases 2–4 result in a reduction in B1-B3 deposition and produce similar deposition in B4–B7 (). In B8–B15, Cases 2–4 increase DF compared with the control by factors ranging from 1.3 to 3.4× with Case 3 providing the highest DF of approximately 20% (). Considering total TB delivery, the condensational growth approach increases dose by a factor of 1.1 to 2.6× compared with the control (). also reports fraction remaining (FR), which is the airborne fraction of the inhaled dose at the exit of B15 that enters the alveolar region. Cases 2–4 all provide alveolar delivered doses greater than 70% with Case 2 providing a maximum of 87.1%. These values are approximately 3× higher than observed with the control.
TABLE 2 Mass median aerodynamic diameters (MMADs) exiting individual sections of the conducting airway model
TABLE 3 Deposition fraction (DF) as a percentage of initial delivered dose within individual regions of the respiratory airways. Comparisons to previously reported commercial products (Flovent MDI, Diskus DPI, Respimat, and Novolizer DPI) (Longest et al. Citation2012a,Citationc) with slow and deep (SD) and quick and deep (QD) inhalations are also included. Fraction remaining (FR) is the percentage of the initial dose that enters the alveolar region
DISCUSSION
A primary outcome of this study was the characterization of nose-to-lung aerosol drug delivery throughout the conducting airways for anatomical conditions consistent with a medium size healthy adult male. Considering both ECG and EEG delivery methods, results indicated that nose-to-lung aerosol delivery was practical and highly efficient. As previously reported by Golshahi et al. (Citation2013), depositional losses in the streamlined cannulas and NMT models were <1% and <3%, respectively, for submicrometer condensational aerosols. Considering aerosol loss in the delivery tubing, cannula, and NMT model combined, the condensational growth approaches reduced deposition fractions by an order of magnitude compared with the control aerosol. In the TB region, condensational growth techniques increased delivery by a factor of 1.1–2.6× compared with 4.7 μm droplets. Moreover, the ECG and EEG techniques maximized TB delivery to the B8–B15 region. The aerosol size exiting B15 was at least 2.5 μm in all cases, which is expected to be retained in the alveolar airways (Stahlhofen et al. Citation1990; Tian et al. Citation2011a). Furthermore, aerosol delivery to the alveolar region was highly efficient for both condensational growth methods with all values >70%.
FIG. 6 Particle deposition locations and deposition fractions (DF) as percentages of the total delivered dose in the 20-cm delivery tube, cannula interface, NMT geometry, and upper TB region B1–B3. Contours represent the geometric diameter of the particle at the point of deposition.
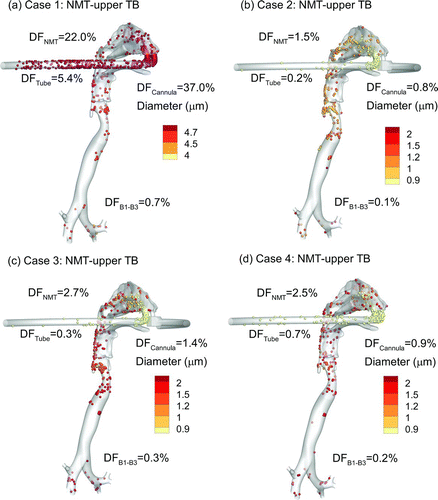
Results of the current study can be used to directly compare the delivery characteristics of ECG versus EEG nose-to-lung aerosol administration. Each of these techniques has inherent advantages and disadvantages. For example, ECG delivery can be used with any aerosol (i.e., the particles or droplets do not need to be hygroscopic); however, a separate saturated vapor line is required for delivery. With the EEG approach the stream of saturated water vapor is not required, but hygroscopic excipients are necessary. In the current study, practically no difference was observed in cannula and NMT depositional losses between these two submicrometer delivery approaches. This is likely because growth does not typically occur in the nasal passages with ECG delivery until the aerosol mixes with the supersaturated humidity stream and growth is slower and continuous with the EEG method. Considering deposition in the TB region, drug only ECG delivery (Case 2) was approximately 40–50% less than with EEG delivery (Case 4). For example, Case 2 and Case 4 deposition fractions in the region of B8–B15 were 7.3% and 14.0%, respectively. This is likely due to the effect of the hygroscopic excipient. The ECG delivery method that included the hygroscopic excipient (Case 3) provided the highest deposition fractions in all regions of the TB airways. For example, total TB DF with Case 3 was 24.7% versus 10.4% with Case 2 and 17.8% with Case 4. Therefore, Case 3 may be the most favorable for maximizing TB dose. For targeting delivery to the alveolar region, Cases 2 and 4 provided similar maximum values of 87.1 and 78.1%, respectively. While Case 2 provided slightly more alveolar delivery, Case 4 resulted in a larger aerosol entering the alveolar region (MMAD = 2.53 μm for Case 2 vs. 4.8 μm for Case 4). As a result, both methods appear ideal for targeting medications to the lower TB and alveolar airways. In addition, deposition targeting could be further optimized by altering the drug to hygroscopic excipient ratio, which was not considered in this study.
One concern with nasal condensational growth delivery is that it may not be as efficient as orally administered condensational methods. This is because of the perceived large hygroscopic growth of submicrometer aerosols in the nose (Schroeter et al. Citation2001), expected high nasal filtration of aerosols (Kelly et al. Citation2004b), and gas conditioning function of the nasal airways. To evaluate oral versus nasal condensational growth administration, comparisons to previous oral ECG (Tian et al. Citation2011a) and EEG (Tian et al. Citation2013) studies are made. Considering ECG oral delivery for a saturated water vapor temperature of 39°C and inhalation flow rate of approximately 30 LPM, Tian et al. (Citation2011a) previously reported a maximum TB DF of 39%, a 2.2 μm aerosol entering the alveolar region, and alveolar retention of 50%. In comparison, maximum TB delivery in the current study was 25% with approximately 71% entering the alveolar airways. As a result, it appears that the deposition pattern of nasally administered condensational aerosols is shifted more toward the alveolar region. For EEG oral delivery, Tian et al. (Citation2013) reported 20% TB deposition, 80% alveolar delivery and a 5.7 μm aerosol entering the alveolar region with a slow and deep inhalation waveform that had a mean flow rate of 37 LPM. These results are very similar to the EEG nose-to-lung administration findings with Case 4 at a similar flow rate in the current study. Therefore, EEG delivery is not affected by nose-to-lung administration compared with oral inhalation. Tian et al. (Citation2013) also found that alveolar delivery with EEG could be increased with higher flow rates. This trend is also likely to be true for nose-to-lung aerosol administration.
FIG. 7 Particle deposition locations and deposition fractions as percentages of the total delivered dose in the LL lobe SIP model. Contours represent the geometric diameter of the particle at the point of deposition.
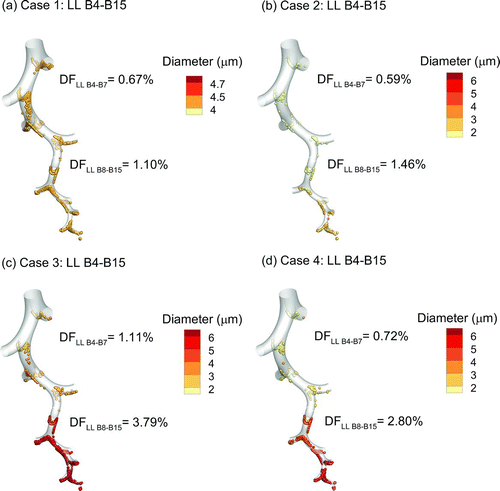
FIG. 8 Comparison of deposition fractions in individual regions of the conducting airways for Cases 1–4.
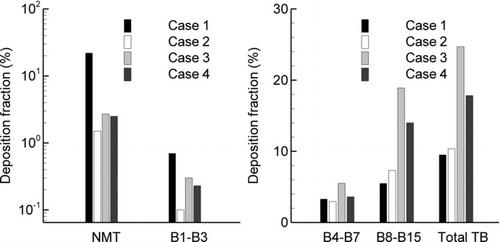
Comparisons of controlled condensational growth nose-to-lung delivery versus commercial oral inhalation performance are provided in . These estimates for commercial products are based on previous CFD simulations with the SIP method through the TB airways for the Flovent HFA MDI (GSK, Raleigh, NC, USA) (Longest et al. Citation2012c), Flovent Diskus DPI (GSK, Raleigh, NC, USA) (Longest et al. Citation2012c), Respimat SMI (Boehringer Ingelheim, Ingelheim, Germany) (Longest et al. Citation2012a), and Novolizer DPI (Meda Pharmaceuticals, Somerset, NJ, USA) (Longest et al. Citation2012a). Considering total TB deposition with the commercial devices, the Respimat SMI provided the best deposition with 13.1% and the two DPIs provided the lowest deposition with approximately 4% each. In comparison, nose-to-lung delivery Case 3 improved TB dose by a factor of 2 to 6×. For alveolar delivery, the nose-to-lung approach provided a range of 70.9–87.1% versus the commercial oral products range of 26–52%. As a result, it can be concluded that the submicrometer nose-to-lung delivery approach is significantly more efficient at delivering medicines to the lungs than current oral inhalation products. It should be recognized that each of these devices also has significant extrathoracic and device deposition losses unlike the ECG/EEG nose to lung approach.
A surprising finding of this study is that nose-to-lung delivery with a conventional aerosol performed relatively well compared to orally inhaled commercial products (). Considering total TB deposition, Case 1 delivery was similar to the MDI and Respimat SMI and approximately 2× greater than the DPIs. Alveolar delivery with Case 1 was similar to the Diskus at approximately 26%, but less than the other commercial products. However, a potential disadvantage of using nose-to-lung delivery with conventional sized aerosols is the exposure of the nose to high concentrations of the drug, which may be important for some medications.
An increasing body of evidence indicates a large role of the lower TB airways in both systemic absorption of inhaled medications (Patton and Byron Citation2007) and the manifestation of airway diseases including asthma and COPD (Lahaije et al. Citation2012; Postma and van den Berge Citation2013). For example, several studies have illustrated that the lower TB airways, with diameters less than approximately 2.4 mm, are most responsible for the functional airway ventilation changes observed with asthma (Kotaru et al. Citation2005; Tgavalekos et al. Citation2005). Airways with diameters less than 2.4 mm correspond to B12 and below in the SIP model of the LL lobe considered in this study. As a second example, idiopathic pulmonary fibrosis is described as starting in the peripheral airways and progressing to the center of the lungs (Ebina et al. Citation2004; Souza et al. Citation2005; Patton et al. Citation2010). Targeting therapeutic aerosols to the lower TB airways is expected to improve the effectiveness of inhaled medications for multiple lung conditions. Results of lower TB dose in the current study are compared to previous studies of commercial products in . Data are presented in terms of bifurcations B8–B11 and B12–B15. Of the commercial products, the best performing case targeting the lower TB region is the Respimat inhaler with a B12–B15 dose of approximately 4%. In comparison, Case 3 improves lower TB dose in B12–B15 to a value of 12%. In the region of B12–B15, the other commercial products deliver approximately 0.5%, and the condensational growth techniques increase deposition by factors as large as 35×.
FIG. 9 Comparison of deposition fractions in the lower TB airways for nose-to-lung delivery with predictions of oral inhalation delivery from commercial inhalers (Longest et al. Citation2012a,Citationc).
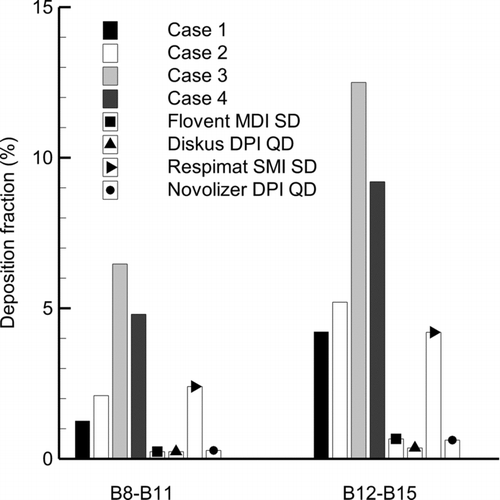
Limitations of the current study include steady state inhalation flow, consideration of one SIP pathway, symmetric branching in the SIP model of B4–B15, evaluation of a single airway geometry, application of a two-equation turbulence model, and use of initially monodisperse aerosols. Previous studies have indicated that both transient inhalation (Sosnowski et al. Citation2006; Li et al. Citation2007; Tian et al. Citation2011b) and polydisperse aerosol size (Longest et al. Citation2012a) influence deposition predictions in the extrathoracic airways. However, the assumptions of steady state flow and initially monodisperse particles in all cases allowed for relative comparisons of the results. Accurate predictions of lung deposition in vivo may require considering more than one SIP geometry and asymmetric airway branching in B4–B15. Furthermore, high intersubject variability in the NMT geometry (Cheng Citation2003; Storey-Bishoff et al. Citation2008; Garcia et al. Citation2009; Golshahi et al. Citation2011) and upper TB anatomy (Choi et al. Citation2009; Walenga et al. Citation2013) are expected to influence deposition. Temperature of the nasal airway surface is also expected to vary and not be a constant 37°C (Keck et al. Citation2000). Considering the geometry selected, use of this single characteristic airway model allows for a relative comparison of nose-to-lung delivery among the four cases evaluated. Additional studies are required to address the effects of a more complete TB geometry and intersubject variability in the NMT and TB regions. Implementation of large eddy simulation (LES) turbulence models have been shown to improve flow field predictions and particle deposition profiles compared with two-equation turbulence models that lack anisotropic near-wall predictions or corrections (Matida et al. Citation2006; Jayaraju et al. Citation2008). However, the current study corrected for anisotropic effects, and LES simulations are difficult to progress toward the inclusion of transient breathing profiles, even more complex airway models including additional SIP geometries, and airway wall motion.
In conclusion, nose-to-lung aerosol administration was characterized for two condensational growth techniques and shown to provide highly effective lung delivery. The implementation of submicrometer aerosols resulted in depositional losses in the cannula and NMT region below 5%. Both the ECG and EEG methods were highly effective with maximum TB delivery occurring for a combination of ECG delivery with a hygroscopic excipient. Alveolar delivery fractions with condensational growth were all above 70% and aerosols entering the alveolar region were above 2.5 μm. Nasal administration of submicrometer condensational aerosols was found to be superior to current oral inhalation products in terms of lung delivery efficiency. In the biologically important lower TB region, nose-to-lung delivery increased drug deposition by factors as high as 35× compared to commercial products. Future work is needed to improve realism of the CFD model including more SIP geometries, transient inhalation, wall motion, and simulation of the alveolar region. However, the current estimates indicate that nose-to-lung delivery with controlled condensational growth is highly efficient, targets aerosol to critical regions within the airways, and should be further investigated with in vitro and in vivo experiments.
FUNDING
This study was supported by Award R01 HL107333 from the National Heart, Lung, and Blood Institute. The content of this article is solely the responsibility of the authors and does not necessarily represent the official views of the National Heart, Lung, and Blood Institute or the National Institutes of Health.
SUPPLEMENTAL MATERIAL
Supplemental data for this article can be accessed on.
ABBREVIATIONS
AS | = |
albuterol sulfate |
B# | = |
bifurcation number (1 is trachea plus main bronchi) |
BD | = |
budesonide |
CFD | = |
computational fluid dynamics |
CT | = |
computed tomography |
dgeo | = |
initial geometric particle or droplet diameter |
DF | = |
deposition fraction |
DPI | = |
dry powder inhaler |
ECG | = |
enhanced condensational growth |
EEG | = |
excipient enhanced growth |
FR | = |
fraction remaining |
HFT | = |
high flow therapy |
LES | = |
large eddy simulation |
LFT | = |
low flow therapy |
LRN | = |
low Reynolds number |
MDI | = |
metered dose inhaler |
MMAD | = |
mass median aerodynamic diameter |
MT | = |
mouth-throat |
NCPAP | = |
nasal continuous positive airway pressure |
NIV | = |
noninvasive ventilation |
NMT | = |
nose-mouth-throat |
QD | = |
quick and deep inhalation waveform |
RH | = |
relative humidity |
SD | = |
slow and deep inhalation waveform |
SIP | = |
stochastic individual path |
SMI | = |
soft mist inhaler |
TB | = |
tracheobronchial |
Supplimental_files_for_publication.zip
Download Zip (17.7 KB)ACKNOWLEDGMENTS
Ross Walenga of the VCU School of Engineering is gratefully acknowledged for construction of the upper TB geometry used in this study.
REFERENCES
- Allen , M. D. and Raabe , O. G. 1985 . Slip Correction Measurements of Spherical Solid Aerosol Particles in an Improved Millikan Apparatus . Aerosol Sci. Technol. , 4 : 269 – 286 .
- Ari , A. and Fink , J. B. 2012 . Inhalation Therapy in Patients Receiving Mechanical Ventilation: An Update . J. Aerosol Med. Pulm. Drug Deliv. , 25 ( 6 ) : 319 – 332 .
- Ari , A. , Harwood , R. , Sheard , M. , Dailey , P. and Fink , J. B. 2011 . In Vitro Comparison of Heliox and Oxygen in Aerosol Delivery Using Pediatric High Flow Nasal Cannula . Pediatr. Pulmonol. , 46 ( 8 ) : 795 – 801 .
- Bhashyam , A. R. , Wolf , M. T. , Marcinkowski , A. L. , Saville , A. , Thomas , K. Carcillo , J. A. 2008 . Aerosol Delivery Through Nasal Cannulas: An In Vitro Study . J. Aerosol Med. Pulm. Drug Deliv. , 21 ( 2 ) : 181 – 187 .
- Cheng , Y. S. 2003 . Aerosol Deposition in the Extrathoracic Region . Aerosol Sci. Technol. , 37 : 659 – 671 .
- Choi , J. , Tawhai , M. , Hoffman , E. A. and Lin , C. L. 2009 . On Intra- and Intersubject Variabilities of Airflow in the Human Lungs . Phys. Fluids , 21 : 101901 – 1 . -17
- Delvadia , R. , Hindle , M. , Longest , P. W. and Byron , P. R. 2013 . In Vitro Tests for Aerosol Deposition II: IVIVCs for Different Dry Powder Inhalers in Normal Adults . J. Aerosol Med. Pulm. Drug Deliv. , 26 ( 3 ) : 138 – 144 .
- Delvadia , R. , Longest , P. W. and Byron , P. R. 2012 . In Vitro Tests for Aerosol Deposition. I. Scaling a Physical Model of the Upper Airways to Predict Drug Deposition Variation in Normal Humans . J. Aerosol Med. , 25 ( 1 ) : 32 – 40 .
- Dhand , R. 2007 . Inhalation Therapy in Invasive and Noninvasive Mechanical Ventilation . Curr. Opin. Crit. Care , 13 ( 1 ) : 27 – 38 .
- Dhand , R. 2012 . Aerosol Therapy in Patients Receiving Noninvasive Positive Pressure Ventilation . J. Aerosol Med. Pulm. Drug Deliv. , 25 ( 2 ) : 63 – 78 .
- Ebina , M. , Shimizukawa , M. , Shibata , N. , Kimura , Y. , Suzuki , T. Endo , M. 2004 . Heterogeneous Increase in CD34-positive Alveolar Capillaries in Idiopathic Pulmonary Fibrosis . Am. J. Respir. Crit. Care Med. , 169 ( 11 ) : 1203 – 1208 .
- Ferron , G. A. 1977 . The Size of Soluble Aerosol Particles as a Function of the Humidity of the Air: Application to the Human Respiratory Tract . J. Aerosol Sci. , 3 : 251 – 267 .
- Ferron , G. A. , Kreyling , W. G. and Haider , B. 1988 . Inhalation of Salt Aerosol Particles–II. Growth and Deposition in the Human Respiratory Tract . J. Aerosol Sci. , 19 ( 5 ) : 611 – 631 .
- Finlay , W. H. 1998 . Estimating the Type of Hygroscopic Behavior Exhibited by Aqueous Droplets . J. Aerosol Med. , 11 ( 4 ) : 221 – 229 .
- Finlay , W. H. 2001 . The Mechanics of Inhaled Pharmaceutical Aerosols , San Diego : Academic Press .
- Fuchs , N. A. and Sutugin , A. G. 1970 . Highly Dispersed Aerosols , Ann Arbor : Ann Arbor Science Publ. .
- Garcia , G. J. M. , Tewksbury , E. W. , Wong , B. A. and Kimbell , J. S. 2009 . Interindividual Variability in Nasal Filtration as a Function of Nasal Cavity Geometry . J. Aerosol Med. Pulm. Drug Deliv. , 22 ( 2 ) : 139 – 155 .
- Golshahi , L. , Noga , M. L. , Thompson , R. B. and Finlay , W. H. 2011 . In Vitro Deposition Measurement of Inhaled Micrometer-Sized Particle in Extrathoracic Airways of Children and Adolescents During Nose Breathing . J. Aerosol Sci. , 42 : 474 – 488 .
- Golshahi , L. , Tian , G. , Azimi , M. , Son , Y.-J. , Walenga , R. L. Longest , P. W. 2013 . The Use of Condensational Growth Methods for Efficient Drug Delivery to the Lungs During Noninvasive Ventilation High Flow Therapy . Pharmaceutical Res. , 30 : 2917 – 2930 .
- Green , D. W. 1997 . Perry's Chemical Engineers’ Handbook , New York : McGraw-Hill .
- Guilmette , R. A. , Wicks , J. D. and Wolff , R. K. 1989 . Morphometry of Human Nasal Airways In Vivo Using Magnetic Resonance Imaging . J. Aerosol Med. , 2 ( 4 ) : 365 – 377 .
- Hess , D. R. 2007 . The Mask of Noninvasive Ventilation: Principles of Design and Effects on Aerosol Delivery . J. Aerosol Med. , 20 : S85 – S99 .
- Hindle , M. and Longest , P. W. 2010 . Evaluation of Enhanced Condensational Growth (ECG) for Controlled Respiratory Drug Delivery in a Mouth-Throat and Upper Tracheobronchial Model . Pharmaceutical Res. , 27 ( 9 ) : 1800 – 1811 .
- Hindle , M. and Longest , P. W. 2012 . Condensational Growth of Combination Drug-Excipient Submicrometer Particles for Targeted High Efficiency Pulmonary Delivery: Evaluation of Formulation and Delivery Device . J. Pharm. Pharmacol. , 64 ( 9 ) : 1254 – 1263 .
- Hinds , W. C. 1999 . Aerosol Technology: Properties, Behavior, and Measurement of Airborne Particles , New York : John Wiley and Sons .
- Jayaraju , S. T. , Brouns , M. , Lacor , C. , Belkassem , B. and Verbanck , S. 2008 . Large Eddy and Detached Eddy Simulations of Fluid Flow and Particle Deposition in a Human Mouth-Throat . Aerosol Sci. , 39 : 862 – 875 .
- Keck , T. , Leiacker , R. , Riechelmann , H. and Rettinger , G. 2000 . Temperature Profile in the Nasal Cavity . Laryngoscope , 110 ( 4 ) : 651 – 654 .
- Kelly , J. T. , Asgharian , B. , Kimbell , J. S. and Wong , B. 2004a . Particle Deposition in Human Nasal Airway Replicas Manufactured by Different Methods. Part I: Inertial Regime Particles . Aerosol Sci. Technol. , 38 : 1063 – 1071 .
- Kelly , J. T. , Asgharian , B. , Kimbell , J. S. and Wong , B. 2004b . Particle Deposition in Human Nasal Airway Replicas Manufactured by Different Methods. Part II: Ultrafine Particles . Aerosol Sci. Technol. , 38 : 1072 – 1079 .
- Kimbell , J. S. , Segal , R. A. , Asgharian , B. , Wong , B. A. Schroeter , J. D. 2007 . Characterization of Deposition from Nasal Spray Devices Using a Computational Fluid Dynamics Model of the Human Nasal Passages . J. Aerosol Med. , 20 ( 1 ) : 59 – 74 .
- Kotaru , C. , Coreno , A. , Skowronski , M. , Muswick , G. , Gilkeson , R. C. and McFadden , E. R. 2005 . Morphometric Changes after Thermal and Methacholine Bronchoprovocations . J. Appl. Physiol. , 98 ( 3 ) : 1028 – 1036 .
- Lahaije , A. , Heijdra , Y. F. , Willems , L. M. , van Helvoort , H. A. C. and Dekhuijzen , P. N. R. 2012 . COPD Anno 2011: Emphasis on Bronch(iol)odilation . J. Aerosol Med. Pulm. Drug Deliv. , 25 ( 3 ) : 148 – 153 .
- Li , W. and Hopke , P. K. 1993 . Initial Size Distributions and Hygroscopicity of Indoor Combustion Aerosol Particles . Aerosol Sci. Technol. , 19 : 305 – 316 .
- Li , Z. , Kleinstreuer , C. and Zhang , Z. 2007 . Particle Deposition in the Human Tracheobronchial Airways Due to Transient Inspiratory Flow Patterns . Aerosol Sci. , 38 : 625 – 644 .
- Longest , P. W. , Golshahi , L. and Hindle , M. 2013a . Improving Pharmaceutical Aerosol Delivery During Noninvasive Ventilation: Effects of Streamlined Components . Ann. Biomed. Eng. , 41 ( 6 ) : 1217 – 1232 .
- Longest , P. W. and Hindle , M. 2010 . CFD Simulations of Enhanced Condensational Growth (ECG) Applied to Respiratory Drug Delivery with Comparisons to In Vitro Data . J. Aerosol Sci. , 41 : 805 – 820 .
- Longest , P. W. and Hindle , M. 2011 . Numerical Model to Characterize the Size Increase of Combination Drug and Hygroscopic Excipient Nanoparticle Aerosols . Aerosol Sci. Technol. , 45 : 884 – 899 .
- Longest , P. W. and Hindle , M. 2012 . Condensational Growth of Combination Drug-excipient Submicrometer Particles: Comparison of CFD Predictions with Experimental Results . Pharmaceutical Res. , 29 ( 3 ) : 707 – 721 .
- Longest , P. W. , Hindle , M. , Das Choudhuri , S. and Byron , P. R. 2007 . Numerical Simulations of Capillary Aerosol Generation: CFD Model Development and Comparisons with Experimental Data . Aerosol Sci. Technol. , 41 ( 10 ) : 952 – 973 .
- Longest , P. W. , Hindle , M. , Das Choudhuri , S. and Xi , J. 2008 . Comparison of Ambient and Spray Aerosol Deposition in a Standard Induction Port and More Realistic Mouth-Throat Geometry . J. Aerosol Sci. , 39 ( 7 ) : 572 – 591 .
- Longest , P. W. and Holbrook , L. T. 2012 . In Silico Models of Aerosol Delivery to the Respiratory Tract—Development and Applications . Adv. Drug Deliver. Rev. , 64 : 296 – 311 .
- Longest , P. W. and Kleinstreuer , C. 2005 . Computational Models for Simulating Multicomponent Aerosol Evaporation in the Upper Respiratory Airways . Aerosol Sci. Technol. , 39 : 124 – 138 .
- Longest , P. W. , Kleinstreuer , C. and Buchanan , J. R. 2004 . Efficient Computation of Micro-Particle Dynamics Including Wall Effects . Comput. Fluids , 33 ( 4 ) : 577 – 601 .
- Longest , P. W. , McLeskey , J. T. and Hindle , M. 2010 . Characterization of Nanoaerosol Size Change During Enhanced Condensational Growth . Aerosol Sci. Technol. , 44 : 473 – 483 .
- Longest , P. W. , Tian , G. , Delvadia , R. and Hindle , M. 2012a . Development of a Stochastic Individual Path (SIP) Model for Predicting the Deposition of Pharmaceutical Aerosols: Effects of Turbulence, Polydisperse Aerosol Size, and Evaluation of Multiple Lung Lobes . Aerosol Sci. Technol. , 46 ( 12 ) : 1271 – 1285 .
- Longest , P. W. , Tian , G. and Hindle , M. 2011 . Improving the Lung Delivery of Nasally Administered Aerosols During Noninvasive Ventilation—An Application of Enhanced Condensational Growth (ECG) . J. Aerosol Med. Pulm. Drug Deliv. , 24 ( 2 ) : 103 – 118 . doi: 10.1089/jamp.2010.0849
- Longest , P. W. , Tian , G. , Li , X. , Son , Y.-J. and Hindle , M. 2012b . Performance of Combination Drug and Hygroscopic Excipient Submicrometer Particles from a Softmist Inhaler in a Characteristic Model of the Airways . Ann. Biomed. Eng. , 40 ( 12 ) : 2596 – 2610 .
- Longest , P. W. , Tian , G. , Walenga , R. L. and Hindle , M. 2012c . Comparing MDI and DPI Aerosol Deposition Using In Vitro Experiments and a New Stochastic Individual Path (SIP) Model of the Conducting Airways . Pharmaceutical Res. , 29 : 1670 – 1688 .
- Longest , P. W. and Vinchurkar , S. 2007 . Validating CFD Predictions of Respiratory Aerosol Deposition: Effects of Upstream Transition and Turbulence . J. Biomech. , 40 ( 2 ) : 305 – 316 .
- Longest , P. W. , Walenga , R. L. , Son , Y.-J. and Hindle , M. 2013b . High Efficiency Generation and Delivery of Aerosols Through Nasal Cannula During Noninvasive Ventilation . J. Aerosol Med. Pulm. Drug Deliv. , 26 ( 5 ) : 266 – 279 .
- Longest , P. W. and Xi , J. 2007 . Effectiveness of Direct Lagrangian Tracking Models for Simulating Nanoparticle Deposition in the Upper Airways . Aerosol Sci. Technol. , 41 ( 4 ) : 380 – 397 .
- Longest , P. W. and Xi , J. 2008 . Condensational Growth May Contribute to the Enhanced Deposition of Cigarette Smoke Particles in the Upper Respiratory Tract . Aerosol Sci. Technol. , 42 : 579 – 602 .
- Matida , E. A. , Finlay , W. H. , Breuer , M. and Lange , C. F. 2006 . Improving Prediction of Aerosol Deposition in an Idealized Mouth Using Large-Eddy Simulation . J. Aerosol Med. , 19 ( 3 ) : 290 – 300 .
- Matida , E. A. , Finlay , W. H. and Grgic , L. B. 2004 . Improved Numerical Simulation of Aerosol Deposition in an Idealized Mouth-Throat . J. Aerosol Sci. , 35 : 1 – 19 .
- Morsi , S. A. and Alexander , A. J. 1972 . An Investigation of Particle Trajectories in Two-Phase Flow Systems . J. Fluid Mech. , 55 ( 2 ) : 193 – 208 .
- Nasr , H. , Ahmadi , G. and McLaughlin , J. B. 2009 . A DNS Study of Effects of Particle-Particle Collisions and Two-Way Coupling on Particle Deposition and Phasic Fluctuations . J. Fluid Mech. , 640 : 507 – 536 .
- Newman , S. 2009 . Respiratory Drug Delivery: Essential Theory and Practice , Richmond : RDD Online .
- Patton , J. S. , Brain , J. D. , Davies , L. A. , Fiegel , J. , Gumbleton , M. Kim , K. J. 2010 . The Particle has Landed-Characterizing the Fate of Inhaled Pharmaceuticals . J. Aerosol Med. Pulm. Drug Deliv. , 23 : S71 – S87 .
- Patton , J. S. and Byron , P. R. 2007 . Inhaling Medicines: Delivering Drugs to the Body Through the Lungs . Nat. Rev. Drug Discov. , 6 : 67 – 74 .
- Postma , D. S. and van den Berge , M. 2013 . Small Airway Dysfunction in Asthma and COPD: Consequences for Therapy and the Future . Respiratory Drug Delivery Europe 2013 , 1 : 1 – 12 .
- Robinson , R. and Yu , C. P. 1998 . Theoretical Analysis of Hygroscopic Growth Rate of Mainstream and Sidestream Cigarette Smoke Particles in the Human Respiratory Tract . Aerosol Sci. Technol. , 28 : 21 – 32 .
- Rubin , B. K. 2011 . Pediatric Aerosol Therapy: New Devices and New Drugs . Respiratory Care , 56 ( 9 ) : 1411 – 1421 .
- Schroeter , J. D. , Garcia , G. J. M. and Kimbell , J. S. 2011 . Effects of Surface Smoothness on Inertial Particle Deposition in Human Nasal Models . J. Aerosol Sci. , 42 : 52 – 63 .
- Schroeter , J. D. , Kimbell , J. S. and Asgharian , B. 2006 . Analysis of Particle Deposition in the Turbinate and Olfactory Regions Using a Human Nasal Computational Fluid Dynamics Model . J. Aerosol Med. , 19 ( 3 ) : 301 – 313 .
- Schroeter , J. D. , Musante , C. J. , Hwang , D. M. , Burton , R. , Guilmette , R. and Martonen , T. B. 2001 . Hygroscopic Growth and Deposition of Inhaled Secondary Cigarette Smoke in Human Nasal Pathways . Aerosol Sci. Technol. , 34 ( 1 ) : 137 – 143 .
- Son , Y.-J. , Longest , P. W. and Hindle , M. 2013 . Aerosolization Characteristics of Dry Powder Inhaler Formulations for the Excipient Enhanced Growth (EEG) Application: Effect of Spray Drying Process Conditions on Aerosol Performance . Int. J. Pharm. , 443 : 137 – 145 .
- Sosnowski , T. R. , Moskal , A. and Gradon , L. 2006 . Dynamics of Oropharyngeal Aerosol Transport and Deposition with the Realistic Flow Pattern . Inhalation Toxicol. , 18 ( 10 ) : 773 – 780 .
- Souza , C. A. , Muller , N. L. , Flint , J. , Wright , J. L. and Churg , A. 2005 . Idiopathic Pulmonary Fibrosis: Spectrum of High-Resolution CT Findings . Am. J. Roentgenol. , 185 ( 6 ) : 1531 – 1539 .
- Stahlhofen , W. , Kobrich , R. , Rudolf , G. and Scheuch , G. 1990 . Short-Term and Long-Term Clearance of Particles form the Upper Human Respiratory Tract as a Function of Particle Size . J. Aerosol Sci. , 21 ( Suppl. 1 ) : S407 – S410 .
- Storey-Bishoff , J. , Noga , M. and Finlay , W. H. 2008 . Deposition of Micrometer-Sized Aerosol Particles in Infant Nasal Airway Replicas . Aerosol Sci. , 39 : 1055 – 1065 .
- Tgavalekos , N. T. , Tawhai , M. , Harris , R. S. , Mush , G. , Vidal-Melo , M. Venegas , J. G. 2005 . Identifying Airways Responsible for Heterogeneous Ventilation and Mechanical Dysfunction in Asthma: An Image Functional Modeling Approach . J. Appl. Physiol. , 99 ( 6 ) : 2388 – 2397 .
- Tian , G. , Longest , P. W. , Li , X. and Hindle , M. 2013 . Targeting Aerosol Deposition to and Within the Lung Airways Using Excipient Enhanced Growth . J. Aerosol Med. Pulm. Drug Deliv. , 26 ( 5 ) : 248 – 265 .
- Tian , G. , Longest , P. W. , Su , G. and Hindle , M. 2011a . Characterization of Respiratory Drug Delivery with Enhanced Condensational Growth (ECG) Using an Individual Path Model of the Entire Tracheobronchial Airways . Ann. Biomed. Eng. , 39 ( 3 ) : 1136 – 1153 .
- Tian , G. , Longest , P. W. , Su , G. , Walenga , R. L. and Hindle , M. 2011b . Development of a Stochastic Individual Path (SIP) Model for Predicting the Tracheobronchial Deposition of Pharmaceutical Aerosols: Effects of Transient Inhalation and Sampling the Airways . J. Aerosol Sci. , 42 : 781 – 799 .
- Vinchurkar , S. , Longest , P. W. and Peart , J. 2009 . CFD Simulations of the Andersen Cascade Impactor: Model Development and Effects of Aerosol Charge . J. Aerosol Sci. , 40 : 807 – 822 .
- Walenga , R. L. , Tian , G. and Longest , P. W. 2013 . Development of Characteristic Upper Tracheobronchial Airway Models for Testing Pharmaceutical Aerosol Delivery . ASME J. Biomech. Eng. , 135 ( 9 ) : 091010
- Wang , Y. and James , P. W. 1999 . On the Effect of Anisotropy on the Turbulent Dispersion and Deposition of Small Particles . Int. J. Multiphase Flow , 22 : 551 – 558 .
- Wilcox , D. C. 1998 . Turbulence Modeling for CFD (2nd ed.) , California : DCW Industries, Inc. .
- Xi , J. and Longest , P. W. 2007 . Transport and Deposition of Micro-Aerosols in Realistic and Simplified Models of the Oral Airway . Ann. Biomed. Eng. , 35 ( 4 ) : 560 – 581 .
- Xi , J. and Longest , P. W. 2008a . Effects of Oral Airway Geometry Characteristics on the Diffusional Deposition of Inhaled Nanoparticles . ASME J. Biomech. Eng. , 130 : 011008
- Xi , J. and Longest , P. W. 2008b . Numerical Predictions of Submicrometer Aerosol Deposition in the Nasal Cavity Using a Novel Drift Flux Approach . Int. J. Heat Mass Transfer , 51 : 5562 – 5577 .
- Xi , J. and Longest , P. W. 2009 . Characterization of Submicrometer Aerosol Deposition in Extrathoracic Airways During Nasal Exhalation . Aerosol Sci. Technol. , 43 : 808 – 827 .
- Xi , J. , Longest , P. W. and Martonen , T. B. 2008 . Effects of the Laryngeal Jet on Nano- and Microparticle Transport and Deposition in an Approximate Model of the Upper Tracheobronchial Airways . J. Appl. Physiol. , 104 ( 6 ) : 1761 – 1777 .
- Yeh , H. C. and Schum , G. M. 1980 . Models of Human Lung Airways and Their Application to Inhaled Particle Deposition . Bull. Math. Biol. , 42 : 461 – 480 .