Abstract
Biological particles are rarely individual organisms, but are clusters of organisms physically bound to one another, or bound to other material present in the environment. The size and composition of these bioclusters contribute to the protection of the organisms within the core of cluster from the harmful effects of ambient UV light. The use of ultraviolet irradiation has been evaluated in the past as an option for decontaminating surfaces and air; however, previous studies were conducted with single spores, or poorly characterized polydispersed aerosols making comparisons between studies difficult. This study is intended to evaluate the effect of UV-C irradiation on monodispersed particles of spore clusters with mean diameters of 2.8 μm and 4.4 μm, and single spores of Bacillus atrophaeus var. globigii on fixed surfaces and as aerosol. The D90, the UV-C irradiation doses at which 90% of the colony forming units were rendered nonculturable, for single spores and spore clusters of 2.8 and 4.4 μm on surfaces were 138, 725, and 1128 J/m2, respectively. The respective values for airborne spores were 27, 42, and 86–94 J/m2. The first-stage decay rate constant for the surface exposure ranged from 0.012 for single spores to 0.003 for 4.4 μm clusters. Similarly, the aerosol decay rate constant ranged from 0.12 for single spores to 0.04 for 4.4 μm clusters. The results of this study demonstrate that the decay rate of spores contained in clusters is proportional to the overall particle size, and that it is harder to inactivate large clusters on surfaces.
Copyright 2014 American Association for Aerosol Research
INTRODUCTION
The health risks associated with bioaerosols arise from a multitude of naturally occurring factors such as airborne allergens, spread of communicable diseases through coughing and sneezing, naturally occurring environmental releases, and the potential for unnatural releases of pathogens, accidental or intentional. The nature of these airborne bioparticles makes them exceedingly difficult to inactivate through physical or chemical means. Traditional disinfectant approaches commonly used for surface decontamination, such as contact with liquid bleach and other chemical disinfectants, are not practical for airborne particles. An alternative approach which has been investigated with various degrees of success, measured by the log reduction of culturable microorganisms, is the use of ultraviolet (UV) irradiation. Germicidal UV lights are increasingly being used indoors to disinfect both the air and surfaces due to the ease of use and perceived effectiveness of UV irradiation in indoor settings (CDC Citation1994).
The UV-C spectrum is defined as the wavelengths between 200 nm and 280 nm while UV-B and UV-A are defined as wavelengths between 280 to 315 nm and 315 to 400 nm, respectively, (McDonnell Citation2007). While UV-A and UV-B penetrate through the Earth's atmosphere from the Sun, UV-C is filtered by the Earth's atmosphere and fails to reach the Earth's surface (Coohill and Sagripanti Citation2008). The use of UV-C as a potential disinfectant technology, as opposed to UV-A and UV-B, is due to its higher DNA damaging energy that results in the formation of thymine dimers leading to inactivation of spores (Kowalski Citation2009). A number of health care facilities have taken advantage of the disinfectant properties of UV-C irradiation and have installed lights as supplemental microbial control due to their low cost, ease of application, and high kill efficiencies (CDC Citation1994; Kowalski Citation2009; Rutala et al. Citation2010).
The benefits of the germicidal effect of UV lights was demonstrated in an early study reporting a reduced number of cases of influenza infection in a veteran's hospital equipped with UV-C producing bulbs compared to nearby nonirradiated hospital rooms (McLean Citation1956). Since then, several additional studies have shown reduction of bacterial spores and vegetative cells due to upper room air UV irradiation (Miller and Macher Citation2000; Ko et al. Citation2002; Xu et al. Citation2003, Citation2005; Rutala et al. Citation2010). In addition to hospital rooms, installation of UV lights in office ventilation systems has also been shown to reduce the airborne bacterial load. Menzies et al. (Citation2003) reported that UV lights installed in office ventilation systems reduced 99% (2 Log10) of microbial and endotoxin concentrations on irradiated surfaces within the ventilation systems and simultaneous reduction was observed in respiratory and musculoskeletal symptoms of the workers of the office building. These findings indicate that UV light can contribute to the control of contagious disease in an environment outside of the controlled confines of a laboratory (CDC Citation1994).
Recent laboratory studies evaluating the kill effect of UV-C were conducted with single spores or close to single spore sizes on surfaces and in the air (Miller and Macher Citation2000; Ko et al. Citation2002; Lin and Li Citation2002; Xu et al. Citation2005; Foarde et al. Citation2006; Menetrez 2006; Coohill and Sagripanti Citation2009; King et al. Citation2011). However, by neglecting multispore clusters, little is known about the decay of potentially equally hazardous larger clusters such as those belonging to aerosols released by sneezing and coughing and other aerosols generated by crude or sophisticated methods. Larger particles consisting of multiple layers of spores are of particular concern due to the potential for the outer layers of spores forming a sacrificial protective sheathing around an inner core of viable and infectious pathogens. Although no empirical data exist to confirm or refute mathematical estimations of the penetration of UV light through multiple layers of spores, calculated UV penetration efficiencies through spores have been published (Coohill Citation1986). Based on those calculations, 61% of the incident light is predicted to penetrate through the first layer of spores and reach the second layer, and 61% of that energy is predicted to penetrate through the second layer and reach the third layer resulting in 37% of the incident light reaching the third layer (Coohill Citation1986). Therefore, only a fraction of the incident radiation is expected to reach spores in the center of a multilayer cluster.
The ability of UV-C to kill monodispersed aerosols consisting of well-characterized agglomerates has not been well researched, and therefore questions still remain as to the efficiency of UV-C to disinfect multiorganism biological clusters. The study presented here is designed to evaluate the effectiveness of UV-C on inactivating Bacillus atrophaeus var. globigii (BG) spores in clusters of two sizes and single spores, and on both surfaces and as an aerosol, to better develop UV-C induced bioaerosol decay rates for multispore clusters.
TEST METHODOLOGY
Ultraviolet Light Source
A low-pressure UV-C lamp (Sankyo Denki Co., LTD., Japan) producing a wavelength of 254.3 nm was used as the source of UV-C irradiation. The amount of irradiation emitted was recorded throughout the study and the dose received by the organisms was controlled by the duration of the UV light exposure at a given flux.
Spore Preparation
Stock spore solutions of BG (Dugway Proving Grounds, batch 040) were prepared by cleaning the powder and removing the large particles. The cleaning was conducted by adding approximately 5 g of dry powder into a 50 mL centrifuge tube filled to the 40 mL mark with deionized water, and centrifuging at 4000 RPM for 20 min to pelletize the spores. The supernatant was discarded, and replaced with 40 mL of clean deionized water. This cleaning procedure was repeated three more times. After the fourth resuspension, the spore mixture was allowed to sit at 4°C overnight to allow large particles consisting of more than one spore to settle to the bottom of the tube while single pores remained suspended in solution. The supernatant with single spores was removed for use in the experiment. The stock was kept at 4°C in ultrapure water for the remainder of the testing.
Aerosol Generation Methods
An ink jet aerosol generator (IJAG) (manufactured by US Army Edgewood Chemical Biological Center), described in Kesavan et al. (Citation2008) was used to generate clusters and single spores for the fixed-particle studies on surfaces. A 12-nozzle ink jet cartridge (HP 51612A) was purchased empty and filled with a slurry of BG spores, prepared as described earlier in the spore preparation section, and suspended in deionized water for particle production with the IJAG. The 67 μm droplets produced by the ink jet cartridge are passed through the heating tube of the IJAG to evaporate the water in the droplets resulting in smaller dry residue particles. The heating tube can be turned on or off depending on the particle (large wet or small dry) required for testing. A Collison nebulizer (BGI Inc., Waltham, MA, USA) was used to generate single spores for the aerosol studies and a SonoTek aerosol generator (Sono-Tek Corporation, Milton, NY, USA) was used to generate clusters for the aerosol testing.
Spore Quantification Method
The number of spores per particle was counted by producing droplets from the IJAG without the heater turned on. The wet droplets were deposited onto microscope slides for visualization and numeration of spores per droplet. Individual spores within each impact splat were visually counted under a 40× objective to determine the number of spores per particle. Based on the counting of spores on different size particles, the equation indicating the total number of spores in a cluster is given by Equation (1)
In addition, an experiment was conducted to show that the dry clusters disassociate into individual spores when wetted as this is important in quantification of spores. This experiment was conducted by collecting both droplets containing a liquid suspension of spores and the residue-dried clusters containing an identical number of particles for comparison. Approximately, 4371 droplets were collected in 20 mL of PBS with 0.01% Triton X by directly injecting the output of the IJAG close to the liquid surface in a 50 mL centrifuge tube. An identical number of dried clusters was deposited onto membrane filters residing within filter holders and connected to vacuum pumps. The filters were dried by pulling air for an additional 3 min. Eight samples containing droplets and eight samples containing dried clusters were collected for recovery, plating, and enumeration. The dry filters were put into 50 mL centrifuge tube with 20 mL of PBST with 0.01% Triton X and agitated on a multitube vortexer (VWR Scientific, Radnor, PA, USA) for 5 min. Samples were quantified by diluting (as needed) and plating 100 μL of samples in triplicate on tryptic soy agar and incubating overnight at 37°C and enumerating the next day. The results indicated that there was no statistically significant difference (p > 0.09) between the wet droplets and dried clusters. This indicates that the clusters were fully separating into individual spores during the 5 min of vortexing. The wet droplets, in general, provided an average of 830.0 ± 119.5 colony forming units (CFU)/mL compared to dried clusters providing an average of 955.8 ± 125.1 CFU/mL with an n = 8. The slight difference in number of CFUs may be due to changes in the IJAG cartridge during the test. IJAG cartridges were changed if they clogged during the test and the particle size produced might have been slightly different with different cartridges.
SURFACE EXPOSURE TEST METHODOLOGY
Surface exposure tests were conducted with single spores (length = 0.89–1.63 μm; diameter = 0.41–0.86 μm) (Carrera et al. Citation2006) and two different size clusters (2.8 and 4.4 μm). Single spores and BG spore cluster particles were generated by an IJAG and were deposited on polycarbonate membrane filters for the UV exposure. The spores deposited on filters varied based on size in order to deposit a consistent number of total particles for this experiment. The number of CFUs ranged from 1.3E6 to 1.1E7 per filter for all three particle sizes for the 8 h exposure. The size distribution of spore clusters was measured by an aerodynamic particle sizer (APS, TSI Inc., Shoreview, MN, USA). The mass mean diameter (geometric standard deviation) of the bioclusters measured 2.8 (1.1) μm and 4.4 (1.1) μm, respectively. The particle size distribution is provided in terms of mass distribution as the clusters separate into individual spores in a solution and the mass (volume) distribution represents this more accurately than the number distribution. The single spores suspended in water were pipetted on to filters or the wet droplets of the IJAG were deposited onto filters, so there is no APS size distribution to report for the single spores.
Single spores, visually verified by viewing under a light microscope, were deposited on membrane filters by pipetting a spore suspension or using the wet droplets produced with the IJAG. A pump sampled the air through the polycarbonate membrane filters for a minimum of 5 min to dry the particles before the UV exposure. The clusters generated by the IJAG were deposited on the filters by mostly impaction, and the filters were moved while under the IJAG output tube to prevent clusters falling on top of each other. The data points reported in this study are an average of ten runs or more for ≤60 min for single spores and 4.4 μm clusters, and ≤10 min for 2.8 μm clusters. All the 8 h exposure run results were an average of 10 runs or more, but the other data points were obtained with n = 2 or 4. The relative humidity (RH) value measured ranged from 45% to 67% during the surface exposure testing.
Test filters with deposited organisms for each experimental replicate were gently removed from the filter holders (F2, aluminum, BGI, Waltham, MA) and placed on a cardboard backing with the filter edges pinned to the cardboard to prevent the filters from curling during UV exposure. The test filters were placed inside the Plexiglass (U.S. Plastic Corp., Lima, OH, USA) exposure chamber, and exposed to UV-C for predetermined lengths of time which correlate with the desired irradiation dose (King et al. Citation2011). After irradiation exposure, filters were removed from the exposure chamber and spores were extracted using a validated recovery procedure developed by ECBC. This recovery procedure was validated by pippetting a known amount of liquid suspension of spores on to filters connected to a vacuum pump that was pulling air to mimic sampling. Similar amount of spores were put into centrifuge tubes containing liquid to make the reference samples. Filters containing spores were placed in centrifuge tubes with liquid and vortexed as described earlier to remove the spores from the filters into the liquid. All samples were plated, incubated, and enumerated to quantify the spores in the liquid. A statistical t-test showed that there is no difference between the test samples and the reference samples indicating that the procedure fully removed the spores from the filters.
This filter spore extraction procedure consists of placing the filters into 50 mL centrifuge tubes with 20 mL of phosphate-buffered saline with 0.01% Triton X, and vortexing on a multitube vortexer (VWR Scientific, Radnor, PA, USA) for 5 min. One hundred microliters of the extraction solution consisting of recovered spores was plated in triplicate on tryptic soy agar and incubated overnight at 37°C for enumeration of culturable fraction of the organisms. The survival fraction was determined by comparing the test filter results (ten filters) to reference filters (three filters) which were run and processed identically to the test filters in the absence of UV light.
Surface UV Dosage Calculations
The UV flux at the location of the filters was measured by an UV meter (Model UV-3718-4 Radiometric Detector, Gigahertz-Optik, Newburyport, MA, USA). The measurements were taken after at least 15 min of UV light warmup time as readings were not stable during the first 15 min. Similarly, exposures were also conducted after the 15 min of warm up. The UV dose, or fluence, received by spores was calculated by multiplying the flux by the exposure time. The experimental results are shown as culturable fraction as a function of UV dose.
AEROSOL EXPOSURE TEST METHODOLOGY
Aerosol experiments were conducted in a 0.14 m3 Plexiglass chamber as shown in King et al. (Citation2011). Single spores were generated with a Collison nebulizer and bacteria clusters were generated with a SonoTek aerosol generator. The size distribution measured by the APS indicated the mass mean diameter (GSD) for the single spores and the two cluster sizes as 1.2 (1.5) μm, 2.8 (1.3) μm, and 4.4 (1.3) μm, respectively. A Collison nebulizer generated the single-spore aerosol for 5–6 min into the aerosol chamber. For the 2.8 μm tests, a SonoTek nebulizer generated particles for 3–4 min and for the 4.4 μm tests the SonoTek nebulizer generated the particles for approximately 1 min. These aerosol generation times were chosen to produce enough spores for quantification purposes. The chamber air was mixed throughout the experiment to mix the aerosol in the chamber. A one-min air sample at an air flow rate of approximately 12.5 L/min was taken to determine the initial organism concentration. The initial concentration of single spores ranged from 4.9E5 to 4.8E6 CFU/L of air. The 2.8 μm cluster concentration ranged from 1.6E6 to 5.8E6 CFU/L of air and the 4.4 μm cluster concentration ranged from 1.4E6 to 5.7E6 CFU/L of air. The UV-C lamp was activated for different lengths of time to provide exposure to the organisms in the chamber followed by one-min air samples taken periodically at an air flow rate of approximately 12.5 L/min on gelatin filters (Sartorius, Goettingen, Germany). The organisms collected on filters were recovered using the recovery procedure recommended by the manufacturer which consists of placing the filter with PBST in a water bath at 37°C and shaking for 5 min using a Shak R. Bath (Model 3580, Lab-Line Instruments, Melrose Park, IL, USA). The samples were plated in triplicate on tryptic soy agar and incubated overnight at 37°C to determine the culturable quantity. These results were compared to samples taken from identical experiments with the UV lamps turned off to determine the natural bacteria decay in the chamber so that the UV-dependent decay, corrected for natural decay, can be calculated. All the single-spore tests were conducted with an n = 10, and the cluster tests were conducted with an n = 11.
The fluence of the aerosol test chamber was measured by dividing the chamber into a 5 × 5 × 3 matrix and by measuring the flux at each of those locations as shown in . From these measurements, the average flux of the chamber was calculated and the fluence was determined by multiplying the flux by the exposure time. For those calculations and for comparative purposes, the authors assume each particle spends identical amounts of time in each section of the previously described chamber matrix. The RH remained at 30%–40% during this test.
UV Disinfection Model
The survival fraction data of the surface exposures did not show significant shoulder region but showed two distinctive slopes for the curves as explained by Deguchi et al. (Citation2005). A two-stage decay model was fitted to surface exposure data, and the equation for the model is given in Equation (2)
The aerosol exposure data were slightly different in that the data had a shoulder region and were suitable for fitting with the one-stage multihit target model as shown in Equation (3) (Kowalski Citation2009):
Statistical Analysis
An analysis of variance (ANOVA) statistical test (Microsoft Excel, USA) determined the differences in survival fraction between the three particle sizes deposited on surfaces and in air. For the surface exposure tests, the 2 min and 8 h time points had the survival fraction data for all three particle sizes; therefore, the ANOVA statistical test was conducted for these time points. The other surface exposure time points were not similar for the three particle sizes as the decay slopes were different, and exposure times were selected to achieve more points in the fastest decay portion. On the other hand, for the aerosol exposure, all three particle sizes followed similar exposure conditions. The null hypothesis of this test was that the survival fraction was similar for all three particle sizes and it was rejected if the p values were less than 0.05.
RESULTS
The decay curves of single spores and spore clusters of 2.8 μm and 4.4 μm, exposed to UV-C light on surfaces are shown in . Single spores were inactivated significantly faster (p < 0.001 at time 2 min) compared to the 2.8 and 4.4 μm clusters which had similar overall survival fraction curves. Evaluating the first 1000 J/m2 exposure shows that 4.4 μm particles had a slower rate in loss of culturability compared to the 2.8 μm particles. An ANOVA conducted at 60 min exposure (equals to 2880 J/m2) showed that the survival fraction was significantly different for all three particle sizes (p < 0.001). The D 90 values (irradiation dose required to kill 90% of the organisms) of surface-bound single spores and 2.8 and 4.4 μm clusters were 138, 725, and 1128 J/m2, respectively.
FIG. 2 UV-C survival fraction (+ standard deviation) curves for the single and BG cluster particles (2.8 and 4.4 μm) deposited on membrane filter surfaces. (Top) Survival fraction for fluence up to 1,000 J/m2. (Bottom) Survival fraction for fluence up to approximately 25,000 J/m2.
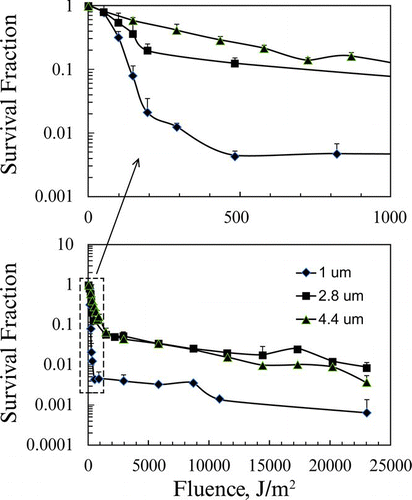
FIG. 3 The survival fraction (+ standard deviation) curve for aerosolized single and BG clusters (2.8 and 4.4 μm).
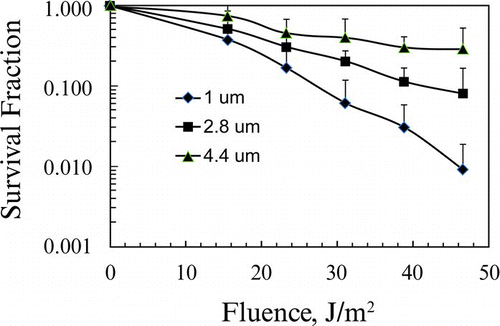
The survival fraction curves for the aerosolized single spores and clusters of 2.8 and 4.4 μm, are shown in . An ANOVA test at each exposure showed that the survival fractions for the three sizes were significantly different and the single spores are inactivated faster (p < 0.02 for all data points). Under the experimental conditions presented here, the 4.4 μm particles did not reach 1 log kill. Therefore, the survival fraction curve was estimated for the 4.4 μm particle to determine a range for the D 90 value. Two estimate curves were generated (data not shown) to obtain the D 90 values; one curve encompassing the entire data set and the second being composed of the last three data points. The D 90 values of the aerosolized spores for single spores, and clusters of 2.8 and 4.4 μm were 27, 42, and 86–94 J/m2, respectively. The D 90 values for the surface and aerosol exposures are given in and these results show that aerosolized spores, whether individual or as agglomerates, were inactivated faster compared with similar size organisms fixed onto surfaces.
TABLE 1 The fluence values for 90% kill (D 90) of BG spore clusters (2.8 and 4.4 μm) and individual spores on surfaces and in the air
The surface survival fraction data were fitted to a two-stage decay model as shown in Equation (2) and the values are given in . The results show that the rate constants were higher for single spores compared to the clusters and the rate constant increased as the cluster size decreased. The aerosol survival fraction data were fitted to a multihit target model as the slight shoulder region influenced the multihit target model to fit better. The multihit target model is shown in Equation (3) (methods section) and the values are given in . Here also, the rate constant was higher for the single spores and the rate constant increased as the cluster size decreased as seen in the surface exposure studies. The n value also indicates that the slope is flatter for the larger size clusters.
TABLE 2 The first-stage rate constant (k 1, m2/J) values for the two-stage decay curve for spores on surface, calculated using Equation (2)
DISCUSSION
The decay rates reported in this manuscript were calculated based on CFUs counted after sample recovery and plating. Under a number of circumstances, including being subjected to UV light, spores can be injured or otherwise inactivated for periods of time in which the spores are neither culturable nor dead. Repair mechanisms and activation mechanisms are not fully understood and continue to be extensively researched by the scientific community. Determining whether the decrease in culturable spores was a result of loss in culturability or death is well beyond the intended scope of this work and therefore no efforts were made to revive spores. Nonculturable spores in this effort consist of both dead and inactivated spores.
TABLE 3 The rate constant (k) and multitarget exponent (n) values for the multihit target model for the aerosol exposure, calculated using Equation (3)
This study evaluated the likelihood of whether spores located on the outermost layers of a spore cluster would provide a shielding or protective effect against UV-C irradiation allowing for the spores within the core of the cluster to remain unaffected by the irradiation. In both the surface-fixed and aerosol experiments, the data demonstrated a rapid decay in culturable single spores, while the larger particles composed of numerous spores produced data suggesting that loss in culturability of total spores proceeded more slowly and had larger D 90 values as the size of the particle increased. While there could be other unknown or untested factors which led to these results, the authors believe that the higher D 90 values and slower decay rate for spore clusters are a direct result of the outermost layer of spores protecting the core of the biological cluster from UV irradiation. This is supported by Coohill's (Citation1986) calculations that showed only 61% of the incident light penetrate through the first layer to reach the second layer. In our study, we determined that the 2.8 μm particles consisted of approximately 26 individual spores and the 4.4 μm particles consisted of 102 individual spores on average. Although the surface area of the 4.4 μm particles increased and would require more spores to make up the outer layer than the 2.8 μm particle, the inner core of the larger particle would consist of considerably more spores not directly exposed to, or exposed to significantly less, UV irradiation. It is likely for this reason, that we never actually achieved 90% loss in culturability for the 4.4 μm particles during the measurement time and are capable only of estimating the D 90 for aerosolized 4.4 μm spore clusters. Due to the nonlinear nature of the curve, the calculated D 90 value range from 86 to 94 J/m2 depending on whether all of the data were used to plot the graph or whether only the last three data points were used.
As previously discussed, a direct comparison of historical data and previous studies is difficult considering the following test variables: particle placement (air or surface), particle sizes, percent of vegetative cells and spores, experimental design, and test species studied. For example, Kowalski (Citation2009) summarized D 90 values from previous tests for Bacillus species such as Bacillus atrophaeus and Bacillus subtilis, and the results show the range to be from 85 to 149 J/m2 for aerosolized spores and 14 J/m2 for aerosolized vegetative cells. The differences in spore preparation, aerosolization, and sampling methods, or a combination of them, may have contributed to a more rapid decay of our samples than reported by Kowalski (Citation2009). Xue and Nicholson reported that Bacillus subtilis spores deposited on glass slides and exposed to UV-C had D 90 values of approximately 40–120 J/m2 compared to our filter samples with a D 90 average of 138 J/m2. Another study by Menetrez et al. (Citation2006) reported D 90 values of greater than 175 J/m2 for spores irradiated while resting on agar plates which is higher than our reported values. Menetrez used B. subtilus var. orange, B. subtilus var. cream, B. stearothemophilus, B. pumilus, B. megaterium, B. cereus, B. thuringiensis, and B. anthracis stern in their tests. Even higher D 90 values (260–290 J/m2) were summarized by Coohill and Sagripanti (2008) for Bacillus species (spores and vegetative cells of B. anthracis, B. megaterium, and B. subtilis) on surfaces. The differences in D 90 values of seemingly similar experiments could be a result of the surface types where the spores were resting. When exposed to UV irradiation, the glass slides may get heated and result in an additive effect in loss in culturability while BG spores resting on agar plates may be protected by uneven surfaces and a water layer on the surface. In addition, it may be possible for the organisms to perform a higher degree of repair in the nutrient-rich environment and have a higher culturable fraction. In general, B. subtilis spores in liquid require a high dose (D 90 of 245 J/m2) for loss in culturability compared to the spores on surfaces and in air (Nicholson and Galeano Citation2003). RH has been shown to influence susceptibility of both spores and vegetative bacteria to UV light. A study by Lin and Li (Citation2002) showed that organisms are less susceptible at high RH (80%) compared to low RH (50%). The RH values in our study were between 30% and 40% for the aerosol testing, and the organisms may be more susceptible to UV light.
The data of single spores and clusters on surfaces can be fitted to many models; however, the data were more appropriate to be fit to a two-stage decay curve as shown in Equation (2). The first-stage rate constant was significantly higher than the second-stage rate constant for all particle sizes. A two-stage decay model by Kowalski (Citation2009) showed k 1 of 0.0041 m2/J for Bacillus anthracis spores on surface. Our data with BG spores on surface indicate a higher k 1 value of 0.012 m2/J. On the other hand, Brickner et al. (Citation2003) reported even higher k 1 values (0.061–0.082 m2/J) for Bacillus species on surfaces. The difference may be due to the differences in strains, spore preparation, and surface materials. The decay constant values for clusters have not been reported in the literature but our data indicate that clusters have smaller decay constant values compared to the single spores and they are inversely proportional to particle size.
The single and cluster aerosol data were fitted to the multihit target model as shown in Equation (3) and this model provided the best fit compared with other models described by others (Kowalski et al. Citation2000). Our results show that the decay rate constant (k) for single spores BG was 0.12 m2/J and similar results have previously been reported for Staphylococcus aureus (Kowalski Citation2009) that had a decay rate constant of 0.1702 m2/J. Lower decay rate constant values have been reported by Brickner et al. (Citation2003), who showed values ranging from 0.019 to 0.038 m2/J for Bacillus species in the air. As expected, the decay constant values for clusters were smaller compared to the single spores and were inversely proportional to the particle size. Since this is the first study that determined the decay constant values for the clusters, our study results cannot be compared to others.
These study results indicate a potential limitation of UV irradiation as a decontamination technique for spore clusters in the air and on surfaces. Likewise, they also demonstrate the potential for significant biological agent persistence in the environment as an aerosol as well as being fixed to surfaces. The commonly accepted size ranges for respirable biological infectious particles lie between 1 μm and 10 μm. Our data show that aerosolized spores in 4.4 μm particles decayed by approximately one log when exposed to 86–94 J/m2 of UV-C irradiation; however, it required nearly 1128 J/m2 to reduce the number of surface-bound spores composing the 4.4 μm particles by one log. UV irradiation, in conjunction with application of bleach, is a standard and proven decontamination technology inside of biological safety cabinets and laboratory equipment. The use of UV-C as a stand-alone decontamination technology where biological particles are not exposed to long periods of high levels of irradiation, such as in filtration systems, HVAC systems, disinfectants, and air purifiers, may pose a false sense of confidence of air purification. Particle size, and whether the particle is an aerosol or is surface bound, play a major role in decontamination and should be considered in surface and air purification systems.
The results show that single and clustered spores are more rapidly killed when aerosolized than when fixed to a surface despite the point by point variation in irradiation levels while airborne compared to consistent levels of irradiation when fixed to a surface. This is likely due to the larger surface area of airborne clusters being exposed to the UV light due to their movement and rotation, and drying of spores in the air. In contrast, surface-fixed organisms are only exposed to the UV light from one direction and at limited angles. Previous studies examining the recovery efficiencies of spores from various surface substrates demonstrated that there was a significant difference in the recovery efficiencies depending on whether the spores were seeded onto coupons as an aerosol or as a liquid suspension (Edmonds et al. Citation2009). This has a dramatic impact on how technologies should be evaluated in laboratories and how that applies to fielded technologies and “real world” situations. For this reason, we argue that to assign a decay rate based on UV irradiation, the physical state of the spores, surface bound or aerosolized, should be taken into consideration with other obvious variables such as spectrum of light, intensity of irradiation, organism and strain used, and the use of a well-characterized particle size distribution.
REFERENCES
- Brickner , P. W. , Vincent , R. L. , First , M. , Nardell , E. , Murray , M. and Kaufman , W. 2003 . The Application of Ultraviolet Germicidal Irradiation to Control Transmission of Airborne Disease: Bioterrorism Countermeasure . Public Health Rep. , 118 : 99 – 114 .
- Carrera , M. , Zandomeni , R. O. , Fitzgibbon , J. and Sagripanti , J. L. 2006 . Difference between the Spore Sizes of Bacillus Anthracis and other Bacillus Species . J. Appl. Microbiol. , : 1364 – 5072 .
- CDC . 1994 . Guidelines for Preventing the Transmission of Mycobacterium Tuberculosis in Health-care Facilities, 1994. Centers for Disease Control and Prevention . MMWR Recomm. Rep. , 43 : 1 – 132 .
- Coohill , T. P. 1986 . Virus Cell Interactions as Probes for Vacuum Vltraviolet Radiation Damage and Repair . Photochem. Photobiol. , 44 : 359 – 363 .
- Coohill , T. P. and Sagripanti , J. L. 2008 . Overview of the Inactivation by 254 nm Ultraviolet Radiation of Bacteria with Particular Relevance to Biodefense . Photochem. Photobiol. , 84 : 1084 – 1090 .
- Coohill , T. P. and Sagripanti , J.-L. 2009 . Review: Bacterial Inactivation by Solar Ultraviolet Radiation Compared with Sensitivity to 254 nm Radiation . Photochem. Photobiol. , 85 : 1043 – 1052 .
- Deguchi , K. , Yamaguchi , S. and Ishida , H. 2005 . UV-disinfection Reactor Validation by Computational Fluid Dynamics and Relation to Biodosimetry and Actinometry . J. Water Environ. Technol. , 3 : 77 – 85 .
- Edmonds , J. M. , Collett , P. J. , Valdes , E. R. , Skowronski , E. W. , Pellar , G. J. and Emanuel , P. A. 2009 . Surface Sampling of Spores in Dry-deposition Aerosols . Appl. Environ. Microbiol. , 75 : 39 – 44 .
- Foarde , K. , Franke , D. , Webber , T. , Hanley , J. and Koglin , E. 2006 . Technology Evaluation Report: Biological Inactivation Efficiency by HVAC In-Duct Ultraviolet Light Systems, EPA Report 600/R-06/052 .
- Kesavan , J. , Bottiger , J. R. and McFarland , A. R. 2008 . Bioaerosol Concentrator Performance: Comparative Tests with Viable and with Solid and Liquid Nonviable Particles . J. Appl. Microbiol. , 104 : 285 – 295 .
- King , B. , Kesavan , J. and Sagripanti , J.-L. 2011 . Germicidal UV Sensitivity of Bacteria in Aerosols and on Contaminated Surfaces . Aerosol Sci. Technol. , 45 : 1 – 9 .
- Ko , G. , Melvin , F. W. and Burge , H. A. 2002 . The Characterization of Upper-Room Ultraviolet Germicidal Irradiation in Inactivating Airborne Microorganisms . Environ. Health Perspect. , 110 : 95 – 101 .
- Kowalski , W. 2009 . Ultraviolet Germicidal Irradiation Handbook: UVGI for Air and Surface Disinfection , New York : Springer .
- Kowalski , W. J. , Bahnfleth , W. P. , Witham , D. L. , Severin , B. F. and Whittam , T. S. 2000 . Mathematical Modeling of Ultraviolet Germicidal Irradiation for Air Disinfection . Quantitative Microbiol. , 2 : 249 – 270 .
- Lin , C.-Y. and Li , C.-S. 2002 . Control Effectiveness of Ultraviolet Germicidal Irradiation on Bioaerosols . Aerosol Sci. Technol. , 36 : 474 – 478 .
- McDonnell , G. E. 2007 . “ Physical Disinfection ” . In Antisepsis, Disinfection, and Sterilization: Types, Action, and Resistance , Edited by: McDonnell , G. E. 65 Washington , DC : ASM Press .
- McLean , R. L. 1956 . Comments on Reducing Influenza Epidemics Among Hospitalized Veterans by UV Irradiation of Droplets in the Air . Amer. Rev. Res. Dis. , 83 ( Suppl. ) : 36 – 38 .
- Menetrez , M. Y. , Foarde , K. K. , Webber , T. D. , Dean , T. R. and Betancourt , D. A. 2006 . Efficiency of UV Irradiation on Eight Species of Bacillus . J. Environ. Eng. Sci. , 5 : 329 – 334 .
- Menzies , D. , Popa , J. , Hanley , J. A. , Rand , T. and Milton , D. K. 2003 . Effect of Ultraviolet Germicidal Lights Installed in Office Ventilation Systems on Workers Health and Wellbeing: Double-blind Multiple Crossover Trial . The Lancet , 362 : 1785 – 1791 .
- Miller , S. L. and Macher , J. M. 2000 . Evaluation of a Methodology for Quantifying the Effect of Room Air Ultraviolet Germicidal Irradiation on Airborne Bacteria . Aerosol Sci. Technol. , 33 : 274 – 295 .
- Nicholson , L. W. and Galeano , B. 2003 . UV Resistance of Bacillus anthracis Spores Revisited: Validation of Bacillus Subtilis Spores as UV Surrogates for Spores of B. Anthracis Sterne . Appl. Environ. Microbiol. , 69 : 1327 – 1330 .
- Rutala , W. A. , Gergen , M. F. and Weber , D. J. 2010 . Room Decontamination with UV Radiation . Infect. Control Hosp. Epidemiol. , 31 : 1025 – 1029 .
- Xu , P. , Peccia , J. , Fabian , P. , Martyny , J. W. , Fennelly , K. P. , Hernandez , M. and Miller , S. L. 2003 . Efficacy of Ultraviolet Germicidal Irradiation of Upper-Room Air in Inactivating Airborne Bacterial Spores and Mycobacteria in Full-scale Studies . Atmos. Environ. , 37 : 405 – 419 .
- Xu , P. , Kujundzic , E. , Peccia , J. , Schafer , M. P. , Moss , G. , Hernandez , M. and Miller , S. L. 2005 . Impact of Environmental Factors on Efficacy of Upper-Room Air Ultraviolet Germicidal Irradiation for Inactivation Airborne Mycobacteria . Environ. Sci. Technol. , 39 : 9656 – 9664 .