Abstract
The performance of electrostatically charged blown microfiber filter media was characterized for high-volume sampling applications. Pressure drop and aerosol collection efficiency were measured at air pressures of 55.2 and 88.7 kilopascals (kPa) and filter face velocities ranging from 2.5 to 11.25 meters per second (m/s). Particle penetration was significant for particles above 0.5 micrometers (μm) in aerodynamic diameter where the onset of particle rebound was observed as low as 200 nanometers (nm). Particle retention was enhanced by treating filters in an aqueous solution of glycerol. Adding this retention agent eliminated electrostatic capture mechanisms but mitigated inertial rebound. Untreated filters had higher nanoparticle collection efficiencies at lower filter face velocities where electrostatic capture was still significant. At higher filter face velocities, nanoparticle collection efficiencies were higher for treated filters where inertial capture was dominant and particle rebound was mitigated. Significant improvements to microparticle collection efficiency were observed for treated filters at all air flow conditions. At high air pressure, filter efficiency was greater than 95% for particles less than 5 μm. At low air pressure, performance enhancements were not as significant since air velocities were significantly higher through the fiber mat. Measured single fiber efficiencies were normalized by the theoretical single fiber efficiency to calculate adhesion probability. The small fiber diameter (1.77 μm) of this particular filter gave large Stokes numbers and interception parameters forcing the single fiber efficiency to its maximum theoretical value. The adhesion probability was plotted as a function of the ratio of Stokes and interception parameter similar to the works of others. Single fiber efficiencies for inertial nanoparticle collection were compared to existing theories and correlations.
Copyright 2014 American Association for Aerosol Research
INTRODUCTION
A large number of works have examined the performance of fibrous filtration media at filter face velocities greater than 1.0 meter per second (m/s). In a previous work, we have shown that nanoparticle collection efficiencies via impaction and interception deviate from the predictions of Stechkina et al. (Citation1969) for viscous flow when filter face velocities are significantly higher than 0.01–0.1 m/s (Hubbard et al. Citation2012). Increased penetration has been observed and attributed to inertial particle rebound from the fiber surface. The objectives of this study were to (1) characterize the performance of eBMF (electrostatically charged blown microfiber filter media) at filter face velocities ranging from 2.5 to 11.25 m/s at air pressures of 55.2 and 82.7 kilopascals (kPa), (2) formulate quantitative correlations for inertial rebound, and (3) investigate the use of retention agents to mitigate particle rebound thereby extending the use of this particular filter media for high volume sampling applications.
Ellenbecker et al. (Citation1980) measured the performance of stainless steel filters having an average fiber diameter of 8 micrometers (μm). They sampled fly ash nanoparticles at velocities ranging from 1 to 8 m/s and used particle-fiber adhesion probabilities to correlate inertial particle rebound to particle kinetic energies greater than 10−13 J. Ptak and Jarosczcyk (Citation1990) correlated empirical data for adhesion probability to the dimensionless ratio of particle Stokes and interception parameter. Schweers et al. (Citation1994) characterized the performance of stainless steel filters with 30–40 μm fibers and also utilized the adhesion probability to represent deviations from theoretical collection efficiencies. Schweers et al. used experimental data to augment the single fiber efficiency of Suneja and Lee (Citation1974) which characterized collection in the impaction-interception regime for nonviscous fiber flow fields. Rembor et al. (Citation1999) also characterized stainless steel fibers with an average fiber diameter of 20–30 μm using 1–3 μm polystyrene latex spheres (PSL) at filter face velocities ranging from 1–5 m/s. Their measured adhesion efficiencies were less than those predicted by Ptak and Jarosczcyk, although this is not unsurprising since there is a large dependence of particle rebound on particle and fiber properties. Kasper et al. (Citation2010) studied the collection of PSL spheres (1–5 μm) at filter face velocities ranging from 1–5 m/s for stainless steel filter fibers with fiber diameters 8 and 30 μm. They formulated a bounce parameter with similar functionality as Ptak and Jarosczcyk and allowed dendritic structures to form through prolonged filter exposures. Sanchez et al. (Citation2013) observed moderate rebound of silicon dioxide particles from small (1.77 μm) fibers around 1.5 m/s and qualitatively showed that electrostatically charged fibers mitigated particle rebound by comparing collection efficiencies of charged and uncharged fibers.
A number of studies have also shown that coating fibers with retention agents, similar to greasing impaction plates, effectively mitigates particle rebound. To enhance particle retention for micrometer sized aerosols, Mullins et al. (Citation2003) coated polypropylene and polyester fibers (approximately 20 μm in diameter) with water. They saw good improvements in collection efficiency at filter face velocities near 0.5 m/s, although the choice of water as a retention agent has limited use. They also compared oil droplet challenge aerosols to PSL microspheres to assess the role of particle deformation on the absorption of impact energy and mitigation of particle rebound. Pyankov et al. (Citation2008) applied a similar method in the sampling of bioaerosols where they coated polypropylene fibers in tea tree oil to reduce bounce and inactivate viable biological particles. Klouda et al. (Citation2011) deposited oil on to 20–30 μm metal fibers via aerosol deposition. Their analysis of measured single fiber efficiencies utilized the adhesion parameter to represent deviations from theory at a filter face velocity of 10 m/s. They noted that the development of a particle monolayer would result in the recurrence of particle rebound unless the coating wicked over the surface of particles residing on the filter fibers. This suggests that surface tension may be an important parameter in the selection of filter retention agents.
The interested reader is referred to the works of Dahneke (Citation1971, Citation1975, Citation1995) and Tsai et al. (Citation1990) for fundamental reviews of interfacial physical phenomena that govern particle rebound. In summary, conservation of energy arguments are employed to facilitate calculations of coefficients of restitution (ratio of final to initial particle velocity) considering material properties, plastic and elastic deformation, surface adhesion, surface roughness, and the flexural work done if the surface is a thin flexible body (e.g., fiber).
EXPERIMENT
A filter test system capable of operating at high filter face velocities, low air pressures, and low air temperatures, was constructed during the work of Hubbard et al. (Citation2012) to characterize inertial filtration at filter face velocities ranging from 5.0 to 19.0 m/s and at air pressures of 20.2 and 81.0 kPa. The system was modified during the work of Sanchez et al. (Citation2013) to characterize the performance of electrostatically charged filter media at moderate filter face velocities ranging from 0.5 to 2.5 m/s at atmospheric pressure (82.7 kPa in Albuquerque, NM, USA). In the current work, the performance of the same electrostatically charged filter media (eBMF) was measured at higher filter face velocities (2.5–11.25 m/s) and at air pressures of 55.2 and 82.7 kPa (approximately 8.0 and 12.0 psia, respectively) to assess its performance in the high volume sampling regime.
eBMF is an electrostatically charged filter media designed to enhance nanoparticle collection at low filter face velocities with Coulombic and polarization forces. Filter media characteristics are given in where the reader is referred to Sanchez et al. (Citation2013) for a description of methodologies used to measure each filter property.
TABLE 1 Filter properties for eBMF taken from Sanchez et al. (Citation2013)
Three filter face velocities were tested at high and low air pressures. The fiber Reynolds number, Re f , was calculated with the following equation:
TABLE 2 Experimental conditions used to assess filter performance and the influence of inertial particle rebound at high filter face velocities
HEPA filtered air was drawn in to a 5.08 cm (2 inch) diameter stainless steel tube where test aerosol was injected. Diaphragm valves were used to control the filter face velocity and air pressure in the filter test section. Air flow rates were measured using two laminar flow elements (Merriam Process Technologies, Cleveland, OH, USA) with maximum air flow rates of 27.0 and 170.0 m3/h at a pressure drop of 8 inches of water. Two aerosols were generated to test collection efficiencies of eBMF filter media: Arizona road dust (ISO 12103-1 A2, 0.5–10.0 μm) dispersed with a fluidized bed, and sodium chloride (NaCl, 30–400 nanometers) atomized from solution. Particles were electrically neutralized with a bipolar ionizing device prior to injection in to the filter test system (Hubbard et al. Citation2012). Test aerosols were previously shown to possess a charge distribution similar to the Boltzmann equilibrium charge distribution (Sanchez et al. Citation2013). Scanning electron microscope (SEM) images of NaCl and ISO test aerosols, collected on eBMF, are shown in the online supplemental information, Figures S1 and S2, respectively. Particle densities and shape factors for each particle type are given in where NaCl data were taken from the work of Spencer et al. (Citation2007) and ISO 12103-1 data were taken from Endo et al. (Citation1998).
TABLE 3 Particle properties for sodium chloride and ISO test aerosol particles: density and shape factor taken from the works of Spencer et al. (Citation2007) and Endo et al. (Citation1998)
Iso-axial sampling probes were used upstream and downstream of the filter section to collect aerosol samples (Baron and Willeke Citation2001). Air samples were extracted from the filter test system and brought to atmospheric pressure in the constant volume sampling piston apparatus described in Hubbard et al. (Citation2012). A scanning mobility particle sizer (SMPS, TSI, Inc., Shoreview, MN, USA) and Aerodynamic Particle Sizer (APS, TSI Inc.) were then used to measure aerosol concentrations and particle size distributions of sub-micrometer and supra-micrometer particles, respectively. More information on the test setup can be found in Hubbard et al. (Citation2012) and Sanchez et al. (Citation2013).
Particle rebound and increased filter penetration was previously observed for large particles (>1.0 μm) at high filter face velocities (1.5 m/s). We, therefore, tested dry filters (where electrostatic effects are intended to be significant) and “treated” filters modified to reduce particle rebound for particles with large inertia. Treated filters were thus used to assess the influence of retention agents (i.e., surface films on fibers) on collection efficiency. Treated filters were first soaked in isopropanol to remove electrostatic charge (Sanchez et al. Citation2013). Qualitatively this step appeared to promote filter wetting. It also enabled subsequent analysis of filter capture mechanisms by eliminating Coulombic and polarization forces from consideration (Brown Citation1993). Filters were then placed in wide mouth jars and soaked in a 50/50% aqueous solution of glycerol for 5 min, and were then soaked for another 5 min while being sonicated (Model 75HT, VWR International, West Chester, PA, USA) at low power. Sonication was considered to promote fiber wetting for fibers deeper within the filter mat. Each filter was dried for 30 min by passing approximately 100 L/min of dry air through the filter mat prior to its insertion in to the filter test system. The total mass of glycerol solution on each specimen prior to filter efficiency testing was approximately 140 ± 20 milligrams (mg). This is approximately 35.0% of the dry filter weight (400 ± 20 mg). After filter efficiency testing, the mass of glycerol on a filter was 100 ± 20 mg. Glycerol appeared to be lost (approximately 30%) due to re-entrainment in the flow which we hypothesized to occur over a very short duration once the air flow velocities reached steady-state conditions. Glycerol loss due to evaporation was not expected since it has a low vapor pressure.
Glycerol has a density of 1260 kg/m3 and the specific surface area of this filter media was measured at 1.75 m2/g (Sanchez et al. Citation2013). We can thereby calculate the approximate film thickness for these experiments: 107 nm. This represents a 12% increase in average fiber diameter from untreated to treated specimens although it is within the measurement uncertainty of fiber diameter (±330 nm). If we assume constant inter-fiber spacing, the treated filter specimen possesses a solidity (calculated) of 0.11 rather than 0.09 which is also not considerably different than the measurement uncertainty in solidity (±0.01). Pressure drop and single fiber efficiencies will be shown with error bars encompassing the growth of fibers with glycerol treatment; measured filter properties () will be used in calculations. Filter treatment with propylene glycol was also attempted but test results showed that glycerol was a more effective retention agent. Only results for glycerol filter treatment will be shown in this work.
DATA
Filter Pressure Drop
Filter pressure drop, ΔPf , is shown as a function of filter face velocity in . Filter face velocities at an air pressure of 55.2 kPa were higher than those tested at 82.7 kPa. The low, middle, and high flow conditions were selected such that each flow condition had equivalent fiber Reynolds numbers at high and low pressure. This filter media required substantial pressure drop to attain filter face velocities greater than a few m/s.
FIG. 1 Filter pressure drop (ΔPf ) as a function of filter face velocity (U) at air pressures of 55.2 and 82.7 kPa. Theoretical predictions of Franklin and Robinson (Citation1972) were used to model pressure drop for compressible (solid line) and incompressible (dashed line) flows through the filter.
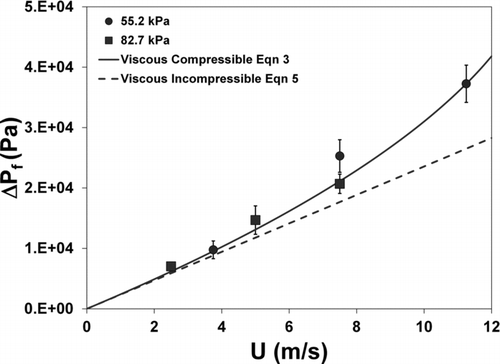
Robinson and Franklin (Citation1972) formulated an equation for pressure drop under atypical filtration conditions: (1) when the fiber Reynolds number is not much less than one (i.e., nonviscous flow around the fiber) and (2) when the filter pressure drop is not much less than the absolute air pressure (i.e., compressible flow). The viscous-compressible filter pressure drop equation is given by the following:
EquationEquation (4) comes from the Kuwabara flow field derivation and gives k=8.5·1011m−2 (within 10% of the fitted value). Jackson and James stated that permeability relations from viscous flow field derivations were good below Re f =10. We see good agreement between data and EquationEquation (3) since our fiber Reynolds numbers are less than one. Filter pressure drop is substantial when compared to the absolute air pressure (10–40%). Compressibility effects cannot be ignored. The viscous-compressible equation of Robinson and Franklin, Equation (3), is shown alongside data in . We also plot the viscous-incompressible equation, i.e., Darcy's law,
Untreated Filter Efficiency
Aerosol collection efficiencies were calculated according to


Total collection efficiency, E, at an air pressure of 55.2 kPa is shown in for untreated filters. Electrical mobility diameters given by the SMPS system were converted to aerodynamic diameters (Sanchez et al. Citation2013). Error bars show the standard deviation of the three independent measurements. For NaCl aerosol, and a filter face velocity of 3.75 m/s, total collection efficiency increased as a function of aerodynamic diameter until approximately 300 nm where we observed the onset of inertial particle rebound. Inertial particle rebound occurs when the energy of impact overcomes the energy of adhesion and the particle does not adhere upon contact. Nanoparticle collection efficiencies were higher at 7.5 m/s due to enhanced inertial capture. At 11.25 m/s, collection efficiency appeared to decrease around 80–100 nm. For ISO aerosol at 2.5 m/s, total collection efficiency decreased from approximately 90% to 70% between 0.5 and 5.0 μm. Particle rebound losses were the largest at the intermediate filter face velocity, 7.5 m/s, and then filter efficiency improved at 11.25 m/s. This appears peculiar although all measurements at 7.5 m/s were consistent. We speculate that plastic deformation of fibers for large particle kinetic energies may contribute to increasing collection efficiencies above 7.5 m/s. Additional experiments at intermediate velocities, 5.63 and 9.38 m/s for instance, would help determine if there is a true minimum in collection efficiency which depends on filter face velocity.
FIG. 2 Total collection efficiency (E) as a function of aerodynamic diameter (d a) for an air pressure of 55.2 kPa. Data are shown for NaCl and ISO test aerosols, for untreated filters.
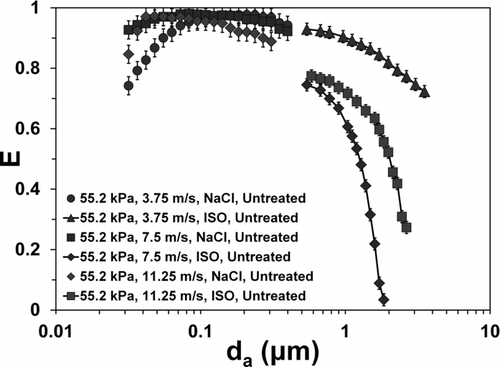
FIG. 3 Total collection efficiency (E) as a function of aerodynamic diameter (d a) for an air pressure of 82.7 kPa. Data are shown for NaCl and ISO test aerosols, for untreated filters.
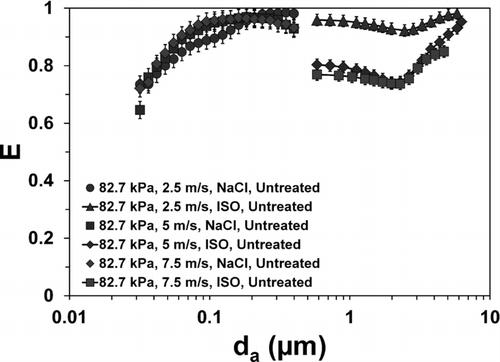
Total collection efficiency at 82.7 kPa is shown in . Collection efficiencies ranged from 60–70% at 30 nm. At 2.5 m/s, filter efficiency did not decrease as it approached 400 nm. Higher nanoparticle collection efficiencies were observed between 50 nm and 200 nm as filter face velocity increased above 2.5 m/s due to enhanced inertial capture. At 5.0 and 7.5 m/s, the onset of inertial rebound appeared near 300–400 nm. For ISO test particles a local minimum in collection efficiency was observed between 2.0 and 3.0 μm similar to the results of Sanchez et al. (Citation2013). The collection efficiency decreased with increasing filter face velocity but was nominally the same for 5.0 and 7.5 m/s. Filter collection efficiency increased again above approximately 3.0 μm which we attributed to increasing interception effects. Nanoparticle collection efficiencies at an air pressure of 55.2 kPa were slightly higher than efficiencies at 82.7 kPa. Gas-nanoparticle slip is more significant at lower pressures and reduces the drag force which would otherwise cause the particle to follow the flow around the fiber; inertial impaction of nanoparticles is more pronounced at lower air pressures.
Microparticle collection efficiency fell significantly as air pressure dropped from 82.7 to 55.2 kPa. We attributed this to higher velocities through the filter mat and not the decrease in pressure. Pressure drop across the filter resulted in flow expansion and an increase in air velocity. The ratio of upstream and downstream air velocities was equal to the ratio of upstream and downstream air pressures. The ratio of upstream and downstream air pressures and filter face velocities is given in Table S1. The low air pressure condition gave much higher flow expansion (an approximate factor of three to four) through the filter and we therefore observed a significant drop in collection efficiency since filter face velocities were significantly higher.
Treated Filter Efficiency
Total collection efficiencies for treated filters are shown in and at air pressures of 55.2 and 82.7 kPa, respectively. Nanoparticle collection efficiencies below 100 nm were higher for untreated filter media. This suggests there were electrostatic capture mechanisms contributing to total collection efficiency at a convection velocity of 3.75 m/s. Particle rebound near 400 nm was reduced for treated filter media. Above 500 nm, collection efficiency was greater than 95% up to approximately 5.0 μm. Here we observed the glycerol filter treatment was an effective retention agent.
FIG. 5 Total collection efficiency (E) as a function of aerodynamic diameter (d a) for an air pressure of 82.7 kPa. Data are shown for NaCl and ISO test aerosols, for treated filters.
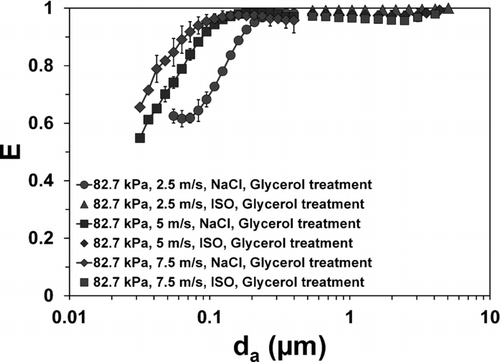
At 55.2 kPa and 7.5 m/s, collection efficiency was nominally the same below 300 nm for treated and untreated filters. This suggests that electrostatic effects were negligible at this convection velocity. Particle rebound near 400 nm appeared to be reduced. Treated filter collection efficiency was greater than 90% up to 2.0 μm but dropped below 90% for particles between 2.0 and 5.0 μm. However, this still represents a significant enhancement in particle retention with respect to untreated filters.
At 55.2 kPa and 11.25 m/s, nanoparticle collection efficiencies increased by approximately 5–10% for treated filters where particle rebound was observed in untreated filters. The collection efficiency of treated filters dropped from 95% to 80% between 0.5 to 3.0 μm. Again we observed good improvement in collection efficiency by the treatment of filters with glycerol.
At an air pressure of 82.7 kPa and a filter face velocity of 2.5 m/s, untreated filter collection efficiencies were 10–20% higher between 30 and 200 nm. We attributed this difference to electrostatic capture mechanisms not present in treated filter media. Collection efficiency above 0.5 μm was greater than 98%.
The collection efficiency of untreated filters was 5–10% higher than for treated filters between 30 and 100 nm at 82.7 kPa and 5.0 m/s again showing subtle electrostatic effects. Glycerol treatment mitigated the onset of NaCl particle rebound near 300 nm and increased collection efficiency to greater than 95% for ISO particles above 0.5 μm.
At 82.7 kPa and 7.5 m/s, NaCl particle collection efficiencies were nominally the same. Electrostatic forces were negligible at this convection velocity and inertial impaction was the dominant capture mechanism. ISO aerosol capture efficiency for particles greater than 0.5 μm was greater than 95%.
SEM images of the upstream and downstream faces of a single filter specimen were taken after ISO aerosol challenge. The upstream filter face SEM image is shown in Figure S3. The first layer of fibers exposed to aerosol showed heavy loading on the upstream face (front 180°). This was consistent with the physical image of inertial and interception particle capture. We did not see particles with diameters much greater than 0.5 μm. This may suggest that larger particles rebound off the first few layers of fibers and adhere downstream once kinetic energy has dissipated. The last layer of fibers was nearly free of captured particles and was consistent with the theory of exponentially decaying particle removal with distance through the filter.
DISCUSSION
Measured total collection efficiencies (E) were converted to single fiber efficiencies (η) using the following equation:
Particle Rebound
The probability that a particle adheres to a fiber upon contact is given by the ratio of measured, ηmeasured, and theoretical, ηtheoretical, single fiber efficiencies. The adhesion probability,φ, was adopted in this work to characterize particle rebound from dry fibers for particles above 0.5 μm (ISO test particles).
We adopted the theoretical single fiber efficiency outlined by Hinds (Citation1999) to describe particle collection, although our airflows were slightly above what might conventionally be considered viscous (Re f < 0.1), and these theories do not account for inhomogeneous fibers and fiber mats. Interception occurs when the limiting particle trajectory comes within a single particle radius of the fiber. The single fiber interception efficiency is given by
In EquationEquation (10), we used the volume equivalent diameter, dve , for particle diameter since it is a closer measure of the geometrical diameter. The single fiber efficiency due to inertial impaction (Stechkina et al. Citation1969) is given by
In EquationEquation (14), either the standard density (ρ0=1000 kg/m3) and aerodynamic diameter may be used, or the actual particle density, ρ p , volume equivalent diameter, and shape factor, χ, may be used. The factor J accounts for the flow field (Stechkina et al. Citation1969) and is given by
The effects of diffusional collection were not considered significant at these conditions. The single fiber efficiency for diffusion is given by the following (Lee and Liu Citation1982):
The sum of η I and η R cannot exceed the theoretical maximum of ηmax=1+R. For all air pressures and filter face velocities tested in this study, the theoretical single fiber efficiency was limited to its maximum value for particles above 0.5 μm. Thus, although our filter air flows were slightly non-viscous, we only used the viscous filter predictions to show that the single fiber efficiency is at its theoretical maximum.
Inertial impaction and interception are more dominant as fiber diameter decreases. eBMF fibers are approximately an order of magnitude smaller those tested by Hubbard et al. (Citation2012), Rembor et al. (Citation1999), Mullins et al. (Citation2003), and Klouda et al. (Citation2011). This makes particle Stokes numbers and interception parameters an order of magnitude larger and gives higher single fiber efficiencies. It also contributes to deviations in the fiber flow field, with respect to the Kuwabara flow field, due to gas-fiber slip.
Ptak and Jarosczcyk (Citation1990) derived the following approximation for adhesion probability:
Curve-fitting software (MATLAB, The Mathworks Inc., Natick, Massachusetts) was used to examine the functionality of measured adhesion probabilities with respect the Stokes number and interception parameter. We adopted a similar form as Ptak and Jarosczcyk although the fit to the data did not improve by allowing the powers of Stk and 1/R to differ. The fit to current experimental data was given by
FIG. 6 Particle-fiber adhesion probability (ϕ) as a function of the ratio of Stokes and interception parameters (Stk/R) following the formulation of Ptak and Jaroszczyk (Citation1990). Mean response (EquationEquation (18)) shown as a solid line with 95% confidence interval limits shown as dashed lines. Only data for ISO test aerosol, and untreated filters, are shown.
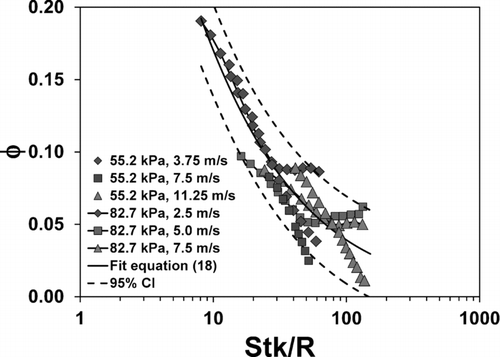
We should note that the adhesion probability function, EquationEquation (18), reflects some combination of rebound from the surface as well as rebound from particles previously deposited on the filter fibers (re-entrainment). Particles deposited directly on to other particles can be seen in Figure S3. As previously discussed by Klouda et al. (Citation2011), the retention agent could potentially wick over the particles and maintain its effectiveness even when particles are not in direct contact with the fiber. The effects of particle rebound and particle entrainment could potentially be decoupled by exposing filters for very short durations (less than five minutes). However, we needed exposures of approximately 30 min to quantify uncertainties in upstream and downstream aerosol concentrations.
Inertial Capture
For discussion of inertial capture, we limited our analysis to treated filters where the role of electrostatic forces could be ignored. Electrostatic effects will be discussed separately below. A number of semi-empirical correlations have been proposed to predict single fiber efficiencies due to impaction and interception for fiber Reynolds numbers greater than one. Suneja and Lee (Citation1974) proposed the following equation:
Hubbard et al. (Citation2012) showed that the total filter efficiency in the inertially dominated regime could be represented with an equation similar to an impactor curve with cutpoint Stokes number, Stk50, and slope, b,
For the range 1<Re f <15 their data gave a power, b, of 2.5 with a standard deviation of 0.5, and Stk50 to be approximately 0.34 for Re f =1.
FIG. 7 Single fiber efficiency for glycerol treated filters and sodium chloride test aerosol where particle Stokes numbers are less than one. Experimental data points (Stk, R) were used to predict values from other correlations: (1) impaction and interception efficiency for viscous flows (EquationEquation (9) + EquationEquation (12)), (2) the correlation of Suneja and Lee (Citation1974) where a Reynolds number of one was assumed, (3) the correlation of Schweers et al. (Citation1994) where a Reynolds number of one was assumed, and (4) in the fit equation of Hubbard et al. (EquationEquation (22)).
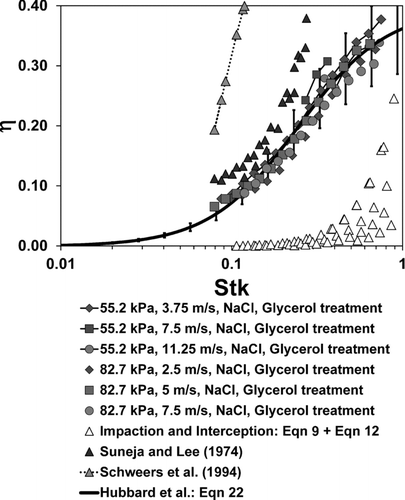
Single fiber efficiencies from treated filters were analyzed to compare the correlations described above for nanoparticle collection in the inertially dominated regime. NaCl particle data were used in the increasing portion of the total collection efficiency curve (E) which corresponds to about Stk<0.6. Data are shown in . Alongside data from this study, the predictions of others are plotted where data points (Stk, R) are inserted in to the following: (1) the sum of impaction and interception efficiencies from Equations Equation(9) and Equation(12), (2) the correlation of Suneja and Lee (Citation1974) where a Reynolds number of one is assumed, and (3) the correlation of Schweers et al. (Citation1994) where a Reynolds number of one is assumed. The sum of impaction and interception mechanisms in a viscous flow field underpredicts measured values considerably. The correlation of Suneja and Lee is close to experimental data for Stk≈0.1 but begins to deviate with increasing Stokes number. The correlation of Schweers et al. (Citation1994) overestimates measured values considerably. We acknowledge that the correlations of Suneja and Lee, and Schweers et al., were not intended for fiber Reynolds numbers less than one.
The cutpoint fit method of Hubbard et al. (Citation2012), EquationEquation (21), was used to fit the data. Single fiber efficiency due to inertial impaction was given by the following:
The correlation coefficient was R 2=0.94. The exponent on Stokes number, 2.0, agreed with the results of Hubbard et al. (Citation2012), b=2.5±0.5. EquationEquation (22) can be expanded as a series to remove the natural logarithm, however, three series terms were required to represent the function and we did not consider this to be a simplification with respect to the functional form of (22).
EquationEquation (21) is plotted alongside data in . Uncertainties in single fiber efficiency were calculated according to Romay et al. (Citation1998) and were approximately 20% of the magnitude of the single fiber efficiency, Δη/η≈20%. Error bars were added to the fit equation to represent uncertainty in single fiber efficiency. Uncertainty bars encompass the majority of our experimental data. The single fiber efficiency developed here, EquationEquation (22), is valid over the following range of filtration parameters: 0.1<Stk<1.0, 0.01<R<0.1, 0.25<Re f <0.75, and 0.09<Kn f <0.14.
Influence of Electrostatic Capture
Electrostatic forces, and particle and fiber charge, were included in the analysis of Sanchez et al. (Citation2013) regarding filtration efficiency of eBMF at filter face velocities ranging from 0.5–2.5 m/s. In past and current work, an alternating current static ionizer was used to neutralize electrostatic particle charge and create conditions very close to the Boltzmann equilibrium charge distribution. Up to approximately 30 nm, the majority of particles have zero charge. The remaining particles have charges distributed about zero with an equal number having positive and negative charge states. The surface charge density, σ, of eBMF fibers was back-calculated from filter efficiency measurements taken at 0.5 m/s: σ=1.2·10−4 C/m2 (Sanchez et al. Citation2013). Here we perform an analysis of electrostatic collection efficiency to assess its influence with respect to inertial capture at filter face velocities greater than 2.5 m/s.
Brown (Citation1993) provides the single fiber efficiency due to induced particle charge (i.e., particle polarization) when the particle charge is negligible,
In Equation (23), N σ is the dimensionless polarization parameter given by
FIG. 8 Single fiber efficiencies for inertial and electrostatic capture mechanisms. EquationEquation (22) was used to calculate inertial capture as a function of particle Stokes numbers. The sum of Coulombic and polarization capture mechanisms were calculated according to EquationEquations (23) and Equation(25) for filter face velocities of 2.5, 5.0, and 7.5 m/s for NaCl particles with diameters greater than 30 nm. The difference between untreated and treated filter specimen single fiber efficiency is plotted for data taken at 82.7 kPa. Reasonable agreement between data and predictions is observed if the fiber charge density is assumed to have a value of 0.6 × 10−4 C/m2.
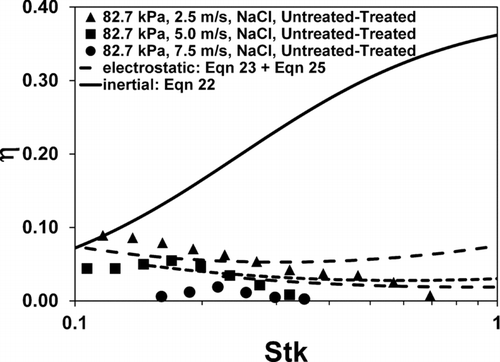
In EquationEquation (26), q is the average charge for a particle of size Dp in the Boltzmann equilibrium charge distribution. For these simple calculations, we adopted the tabulated data presented in Hinds (Citation1999) for average charge q. The Coulombic capture mechanism is dominant for small particles when the particles are in charge equilibrium. As with polarization, the Coulombic capture mechanism is also inversely proportional to convection velocity.
At 82.7 kPa, single fiber efficiencies for treated filters were subtracted from single fiber efficiencies for treated filters. The differences give capture efficiencies attributed to electrostatic attraction and are plotted in . The sum of ησq and ησ was also plotted alongside data for filter face velocities of 2.5, 5.0, and 7.5 m/s. An assumed charge of σ=1.2·10−4 C/m2 predicted significantly higher electrostatic collection than experimentally observed. A value of σ=0.6·10−4 C/m2 gave better agreement with data. At 2.5 m/s, for particles near 30 nm, electrostatic and inertial capture are of similar magnitude. Electrostatic effects are less significant at 5.0 and 7.5 m/s. At larger face velocities and for larger particles, inertial capture becomes the prominent capture mechanism. Predicted electrostatic capture increases for larger Stokes numbers due to the increase in particle diameter. Data do not appear to show this increase which suggests EquationEquation (23) may overpredict the polarization effect for large particles.
SUMMARY
The performance of eBMF was characterized for use in high volume sampling applications. Sanchez et al. (Citation2013) measured collection efficiencies of this media at atmospheric pressure (82.7 kPa) and filter face velocities ranging from 0.5 to 2.5 m/s. We extended characterization to filter face velocities ranging from 2.5 to 11.25 m/s at air pressures of 55.2 and 82.7 kPa. Particle penetration due to inertial rebound was observed at aerodynamic diameters as low as a few hundred nanometers. Microparticle (>0.5 μm) collection efficiencies were reduced at 82.7 kPa and complete penetration was observed for particles above approximately 3.0 μm at 55.2 kPa. Filters were treated in glycerol solution to add a non-volatile film to each fiber and mitigate particle bounce. This negated electrostatic capture mechanisms of eBMF but was an effective retention agent in the inertially dominated filtration regime. For lower velocities nanoparticle collection efficiency was higher for untreated filters signifying the presence of electrostatic capture at moderate convection velocities (2.5 m/s). At higher velocities nanoparticle collection efficiency was greater for treated filters where the onset of particle rebound occurred near several hundred nanometers. Microparticle collection efficiency was significantly higher for all treated filters although low air pressure resulted in collection efficiencies lower than 95% for particles in the 3.0–5.0 μm range. The theoretical single fiber efficiency was calculated for microparticle collection and showed that due to small fiber diameter (i.e., large Stokes and interception parameter), single fiber efficiency was forced to its maximum value of one plus the interception parameter. Measured single fiber efficiencies were normalized by the theoretical single fiber efficiency and plotted as the adhesion probability. The form of Ptak and Jarosczcyk (Citation1990) was adopted and used to fit measured data. Overall adhesion magnitude varied with respect to other works, but that was expected since each particle-fiber material pair will result in differing rebound and adhesive behavior. Single fiber efficiencies for inertially dominated nanoparticle collection were fit with a cutpoint type model where the Stokes number at 50% collection and the slope were used to characterize collection. The empirical correlation given in this work is valid over the following range of filtration parameters: 0.1<Stk<1.0, 0.01<R<0.1, 0.25<Re f <0.75, and 0.09<Kn f <0.14.
SUPPLEMENTAL MATERIALS
Supplemental data for this article can be accessed on the .
2014_02_12_Hubbard_et_al_Supplementary_info.zip
Download Zip (2.9 MB)Acknowledgments
Sandia National Laboratories is a multiprogram laboratory managed and operated by Sandia Corporation, a wholly owned subsidiary of Lockheed Martin Corporation, for the U.S. Department of Energy's National Nuclear Security Administration under contract DE-AC04-94AL85000.
REFERENCES
- Baron , P. A. and Willeke , K. 2001 . Aerosol Measurement: Principles, Techniques, and Applications , New York : Wiley .
- Brown , R. C. 1993 . Air Filtration: An Integrated Approach to the Theory and Applications of Fibrous Filters , New York : Pergamon Press .
- Dahneke , B. 1971 . Measurements of Bouncing of Small Latex Spheres . J. Colloid Interface Sci. , 45 ( 3 ) : 584 – 590 .
- Dahneke , B. 1975 . Further Measurements of Bouncing of Small Latex Spheres . J. Colloid Interface Sci. , 51 ( 1 ) : 58 – 65 .
- Dahneke , B. 1995 . Particle Bounce or Capture—Search for an Adequate Theory . Aerosol Sci. Technol. , 23 : 25 – 39 .
- Ellenbecker , M. J. , Leith , D. and Price , J. M. 1980 . Impaction and Particle Bounce at High Stokes Numbers . J. Air Pollut. Control Assoc. , 30 ( 11 ) : 1224 – 1227 .
- Endo , Y. , Chen , D. R. and Pui , D. Y. H. 1998 . Effects of Particle Polydispersity and Shape Factor During Dust Cake Loading on Air Filters . Powder Technol. , 98 ( 3 ) : 241 – 249 .
- Hinds , W. C. 1999 . Aerosol Technology: Properties, Behavior, and Measurement of Airborne Particles , New York : Wiley .
- Hubbard , J. A. , Brockmann , J. E. , Dellinger , J. , Lucero , D. A. , Sanchez , A. L. and Servantes , B. L. 2012 . Fibrous Filter Efficiency and Pressure Drop in the Viscous-Inertial Transition Flow Regime . Aerosol Sci. Technol. , 46 ( 2 ) : 138 – 147 .
- Jackson , G. W. and James , D. F. 1986 . The Permeability of Fibrous Porous Media . Can. J. Chem. Eng. , 64 : 364 – 374 .
- Kasper , G. , Schollmeier , S. and Meyer , J. 2010 . Structure and Density of Deposits Formed on Filter Fibers by Inertial Particle Deposition and Bounce . J. Aerosol Sci. , 41 ( 12 ) : 1167 – 1182 .
- Klouda , G. A. , Fletcher , R. A. , Gillen , J. G. and Verkouteren , J. R. 2011 . Aerosol Collection Efficiency of a Graded Metal-Fiber Filter at High Airflow Velocity (10 m s(-1)) . Aerosol Sci. Technol. , 45 ( 3 ) : 336 – 342 .
- Kuwabara , S. 1959 . The Forces Experienced by Randomly Distributed Parallel Circular Cylinders or Spheres in aViscous Flowat Small Reynolds Numbers . J. Phys. Soc. , 14 : 527 – 532 .
- Lee , K. W. and Liu , B. Y. H. 1982 . Theoretical Study of Aerosol Filtration by Fibrous Filters . Aerosol Sci. Technol. , 1 : 147 – 161 .
- Mullins , B. J. , Agranovski , I. E. and Braddock , R. D. 2003 . Particle Bounce During Filtration of Particles on Wet and Dry Filters . Aerosol Sci. Technol. , 37 ( 7 ) : 587 – 600 .
- Pich , J. 1971 . Pressure Characteristics of Fibrous Aerosol Filters . J. Coll. Inter. Sci. , 37 : 912 – 917 .
- Ptak , T. and Jaroszczyk , T. 1990 . “ Theoretical-Experimental Aerosol Filtration Model for Fibrous Filters at Intermediate Reynolds Numbers ” . In Proceedings 5thWorld Filtration Congress, Nice 566 – 572 . France
- Pyankov , O. V. , Agranovski , I. E. , Huang , R. and Mullins , B. J. 2008 . Removal of Biological Aerosols by Oil Coated Filters . Clean , 36 ( 7 ) : 609 – 614 .
- Rembor , H. J. , Maus , R. and Umhauer , H. 1999 . Measurements of Single Fibre Efficiencies at Critical Values of the Stokes Number . Part. Part. Syst. Char. , 16 ( 2 ) : 54 – 59 .
- Robinson , M. and Franklin , H. 1972 . The Pressure Drop of a Fibrous Filter at Reduced Ambient Pressures . J. Aerosol Sci. , 3 : 413 – 427 .
- Romay , R. J. , Liu , B. Y. H. and Chae , S. J. 1998 . Experimental Study of Electrostatic Capture Mechanisms in Commercial Electret Filters . Aerosol Sci. Technol. , 28 ( 3 ) : 224 – 234 .
- Sanchez , A. L. , Hubbard , J. A. , Dellinger , J. G. and Servantes , B. L. 2013 . Experimental Study of Electrostatic Aerosol Filtration at Moderate Filter Face Velocity . Aerosol Sci. Technol. , 47 ( 6 ) : 606 – 615 .
- Schweers , E. , Umhauer , H. and Loffler , F. 1994 . Experimental Investigation of Particle Collection on Single Fibers of Different Configurations . Part. Part. Syst. Char. , 11 ( 4 ) : 275 – 283 .
- Spencer , M. T. , Shields , L. G. and Prather , K. A. 2007 . Simultaneous Measurement of the Effective Density and Chemical Composition of Ambient Aerosol Particles . Environ. Sci. Technol. , 41 ( 4 ) : 1303 – 1309 .
- Stechkina , I. B. , Kirsch , A. A. and Fuchs , N. A. 1969 . Studies on Fibrous Aerosol Filters – IV. Calculation of Aerosol Deposition in Model Filters in the Range of Maximum Penetration . Ann. Occup. Hyg. , 12 : 1 – 8 .
- Suneja , S. K. and Lee , C. H. 1974 . Aerosol Filtration by Fibrous Filters at Intermediate Reynolds Numbers (<100) . Atmos. Env. , 8 : 1081 – 1094 .
- Tsai , C. J. , Pui , D. Y. H. and Liu , B. Y. H. 1990 . Capture and Rebound of Small Particles Upon Impact with Solid-Surfaces . Aerosol Sci. Technol. , 12 ( 3 ) : 497 – 507 .