Abstract
This article investigates the black carbon (BC) content of soot formed in premixed and diffusion flames and emitted by light duty gasoline and diesel vehicles. BC is measured photoacoustically and compared with particulate mass collected by filter and calculated from particle size distributions. The BC fraction of soot from rich premixed ethylene flames increases with height above the burner, but can remain well below unity in modestly sooting flames. The BC fraction produced by a propane diffusion flame soot generator (combustion aerosol standard, CAST) falls as the fuel is diluted with nitrogen, the principal means used to adjust the desired particle size. Thermally treating the soot to remove possible condensed semivolatile species does little to change these trends. Transmission electron microscopy (TEM) images show that despite low BC content, these particles display the characteristic fractal-like agglomerate morphology of soot. Particle mass spectra reveal polycyclic aromatic hydrocarbon (PAH) and fullerene fragments associated with low BC soot, which disappear as the BC fraction approaches unity. The results suggest that low BC content reflects immature solid soot that has not carbonized. Particulate matter (PM) measurements from current technology diesel and gasoline vehicles exhibit a high, >80% BC fraction. This is attributed to effective soot carbonization during the expansion and exhaust strokes of the engine, and to the substantial reductions of condensable hydrocarbons by catalytic aftertreatment. These results are discussed with respect to using light absorption-based instruments to monitor engine exhaust PM and using flame-generated soot for PM instrument calibration.
INTRODUCTION
Black carbon (BC) is a measure of carbon-rich particulate matter (PM) defined by optical absorption (Petzold et al. Citation2013). This property immediately makes it relevant to environmental impact in the areas of climate change and visibility. BC contributes to radiative forcing directly by the absorption of sunlight, and indirectly through its effects on cloud albedo and snow and icepack reflectivity (Bond et al. Citation2013). It impairs visibility through the absorption and scattering of light (Watson Citation2002), which is a regulatory concern in the United States (US EPA Citation2012). More broadly, BC is a component of soot emitted from combustion processes used for power generation and transportation and, as such, is of concern for potential adverse air quality and health effects (Janssen et al. Citation2011, 2012).
Table 1 Parameters for min-CAST flames
Soot, BC, and elemental carbon (EC) are often used interchangeably to characterize the solid component of engine exhaust particulate emissions. The standard picture of exhaust aerosol describes a bimodal distribution of particles. There is an accumulation mode of ∼30–200 nm soot agglomerates coated with some degree of condensed hydrocarbons and sulfate that is often accompanied by a nucleation mode of small liquid particles of ∼20 nm created during dilution cooling of the exhaust (Kittelson Citation1998). The amounts of soot, condensed semivolatiles, and nuclei particles depend on engine technology, operating conditions, fuels, etc. But the soot component is generally assumed to be BC, and this serves as the basis of many PM emission measurement methods, including the photoacoustic soot sensor (PASS), laser-induced incandescence (LII), and widely used smoke meter (Schindler et al. Citation2004; Snelling et al. Citation2005; Giechaskiel et al. Citation2014).
A recent study of the miniature Combustion Aerosol Standard (mini-CAST; Mamakos et al. Citation2013) revealed that under many operating conditions the generator produces soot with a BC content substantially below the EC recorded by thermal analysis of filter-collected samples (NIOSH Citation1999). Previous work also observed low BC fractions form a CAST burner and interpreted this as arising from a nucleation mode of condensed organic particles, presumably polycyclic aromatic hydrocarbons (PAHs), produced when operating at high C/O ratios (Schnaiter et al. Citation2006). The study by Mamakos et al. (Citation2013), however, reports that the low BC content persists even after removal of condensed and nucleated organic species by a catalytic stripper (Khalek and Bougher Citation2011). The results suggest that low BC is inherent to the solid soot particles themselves, but no clear connection with CAST operating parameters was identified. This raises concern because of the reliance on light absorption by many PM emission measurement methods and standards, such as used for motor vehicles and aircraft (Ng et al. Citation2007; Stettler et al. Citation2013) and because flame-generated soot is increasingly being considered as a method for instrument calibration and cross comparison (Schindler et al. Citation2004; Ghazi et al. Citation2013; Giechaskiel et al. Citation2013).
The aim of the present article is to take a deeper look into how BC correlates with soot and to understand the conditions leading to low BC fractions. Because this aim extends beyond the specific photoacoustic absorption method used here, we retain the broader designation “BC” while acknowledging that the recommended nomenclature is “equivalent BC” (Petzold et al. Citation2013). To this end we examine BC in premixed ethylene–air flames, the mini-CAST, and motor vehicle exhaust. Premixed flames have provided a wealth of data on nascent soot formation (Wang Citation2011) and offer a means to track how BC evolves in flames. Their study by light scattering and extinction assumes that the soot refractive index varies little with flame conditions (Xu et al. Citation1997; Gothaniya et al. Citation2009). But other studies have revealed the existence of small 3–15 nm particles characterized as spherical and transparent to electron beam (Dobbins Citation2007, Citation2009; Sgro et al. Citation2008; D’Alessio et al. 2009) and which presumably contribute little to BC. The CAST generator is examined to understand better the reasons for soot with low BC values. This is necessary if this otherwise stable source is to provide a useful reference material for solid carbon PM (Baumgardner et al. Citation2012). Finally, we examine PM emissions from four current model light duty vehicles: one port fuel injection (PFI) gasoline, two gasoline direct injection, and one diesel vehicle. There has been disparity in the BC emission factors reported from recent ambient studies of motor vehicle emissions (Strawa et al. Citation2010; Liggio et al. Citation2012; Forestieri et al. Citation2013). Given the recent changes in engine technology and exhaust aftertreatment, it is important to explore their potential impact on BC inventories.
EXPERIMENTAL METHOD
Sources and Sampling
Premixed ethylene–air flames with equivalence ratios of Φ = 2.05 and 2.3 are produced using a McKenna burner. The fuel–air mixture flows through a 6 cm diameter, water cooled, sintered bronze plate surrounded by a sheath of ∼40 L/min nitrogen to shield the flame from the surrounding air. The total fuel–air flow rate is 9.8 L/min, resulting in a velocity of 34 cm/s at an estimated flame temperature of 1700 K. Soot is sampled using a pinhole probe (Maricq Citation2004). An ejector pump pulls a flow of nitrogen past the pinhole that immediately dilutes the entering flame aerosol to quench further soot growth. The dilution factor is ∼400. Secondary dilution by a factor 2–10 is used for the particle instruments as needed.
The mini-CAST soot generator used here is the model 5201c. Soot is produced using a propane–air co-flow diffusion flame. A stream of nitrogen across the top of the flame quenches flame chemistry and sweeps the soot aerosol into a flow of dilution air. Propane, air, and dilution air flows are user settable to adjust the amount and size of soot produced. An additional adjustment is the option to dilute the propane fuel with nitrogen, which is how the six factory default operating points vary soot size. Five of these are examined here, with operating parameters listed in . Propane dilution by N2 increases from flames 1–5, but is not entirely compensated for by the accompanying reduction in air flow. Thus, besides a 14% reduction in oxygen concentration, there is a ∼6% decrease in residence time in this progression. A Dekati FPS (fine particle sampler; Mikkanen et al. Citation2001) is used to dilute the CAST output by a factor of ∼20, with secondary dilution added for the PM instruments.
Four light duty vehicles are tested: (1) 2013 model year gasoline PFI 3.7 L, (2) 2013 gasoline turbocharged direct injection (GTDI) 1.6 L, (3) 2013 GTDI 2.0 L, and (4) 2012 diesel 6.7 L. The gasoline vehicles are equipped with three-way catalysts and were fueled with California certification E10 gasoline, with 10% ethanol, 9.5 ppm sulfur, and 91.7 research octane. The diesel vehicle has oxidation and urea NOx catalysts and a partially drilled out diesel particulate filter (DPF) (used for on-board diagnostics (OBD) PM sensor development). It was run on ultra-low sulfur fuel, with 13.5 ppm sulfur and 40.9 cetane number. The vehicles were tested on a chassis dynamometer with the exhaust connected to a full flow constant volume sampling (CVS) system. Diluent air is filtered, temperature controlled, and conditioned to a −9°C dew point. The total flow of exhaust plus diluent is fixed at 14.2, 17.0, or 29.7 m3/min depending on engine displacement.
PM Measurement
Particles are measured by scanning mobility particle sizer (SMPS), PASS, Dekati mass monitor (DMM), thermal EC/organic carbon (OC), and gravimetric mass. SMPS and PASS measurements of the flame aerosols are conducted with and without thermal pretreatment to remove semivolatile components. Transmission electron microscopy (TEM) and laser ablation mass spectrometry are used to examine the morphology and composition of the mini-CAST soot.
The SMPS records mobility equivalent size distributions by neutralizing the aerosol to a Boltzmann charge distribution, passing the particles through a differential mobility analyzer (DMA), and recording the number of particles exiting as a function of the electric field of the analyzer. Two SMPS are used here: the TSI long DMA run with 10 L/min sheath and 1 L/min aerosol to cover 7.6–278 nm, and the TSI nano-DMA at 5 and 10 L/min sheath and 0.5 and 1 L/min aerosol to cover the 3–79 nm and 3.5–118 nm ranges. The nano-DMA is operated with a 4 L/min bypass to shorten the transport time of the sample to the analyzer and, thereby, reduce diffusion losses of small particles.
The PASS is the Micro Soot Sensor produced by AVL (Graz, Austria) (Schindler et al. Citation2004). It measures aerosol light absorption at 808 nm, chosen at a minimum in the NO2 visible spectrum to avoid interference by this engine exhaust species. Photoacoustic detection enables the light absorbing PM component to be recorded independently from scattering. The Micro Soot Sensor employs a resonant cell; thus, care must be taken to ensure that the same diluent (N2 or air) is used for both setup and measurement.
The DMM determines PM mass from a combination of mobility and aerodynamic size measurement (Mamakos et al. Citation2006). Entering particles are charged by a corona discharge, pass through a parallel plate electric field with a transmission D50 of 50 nm, and proceed through a six-stage low-pressure cascade impactor. The mobility and aerodynamic data are used to estimate particle effective density and thereby calculate PM mass. With no calibration standard available, the DMM is periodically checked against filter-based PM mass to verify performance.
Gravimetric PM mass is determined by collecting PM onto 47 mm diameter Teflon filters. The vehicle exhaust measurements maintain a 47 ± 5°C face temperature, as required by regulation, and the filters were weighed with a 0.1 μg balance in a temperature, humidity, and air flow-controlled weigh room. Filters for the laboratory flame measurements were collected at room temperature, loaded to ∼100 μg, and weighed with a 2 μg balance. Alternatively, the PM is collected onto quartz filters and thermally analyzed for EC and OC with a Horiba MEXA 1370PM. This instrument heats the quartz filters to 1000°C successively in nitrogen and air, assigning the evolved CO2 to OC and EC, respectively. It does not correct for pyrolysis, but the EC + OC total correlates well with gravimetric PM (Akard et al. Citation2004).
Thermal pretreatment of the flame soot is done by a thermo-denuder at 300°C and a thermodesorption tube at ∼600°C. The thermodenuder, a temperature-regulated heat pipe followed by an active charcoal denuder, is from Dekati. This has a maximum operating temperature of 300°C; thus, a simple thermodesorber was constructed to extend the temperature range. It consists of a 20 cm long, 0.95 cm diameter, stainless steel heat pipe employed downstream of the diluters to avoid condensation and re-nucleation of evaporated material (see the online supplemental information (SI), Figure S1). Residence times are ∼0.2 and 0.9 s in the thermodesorber at the nano and long DMA inlet flows, and 1 and 5 s for the thermodenuder.
Size-selected soot particles are collected onto lacy carbon grids for TEM imaging. The flame aerosol first passes through the neutralizer and DMA for size selection, and then is electrostatically precipitated onto the TEM grid. Particle size is chosen on the high side of the mean diameter to minimize the presence of multiply charged particles. The images are obtained with a JEOL 2010F TEM.
Aerosol mass spectra are obtained by laser ablation/ionization, as described previously (Maricq Citation2009). The sample aerosol is formed into a beam by an aerodynamic lens (Liu et al. Citation1995) and crosses the intersection of a 193 nm ArF excimer laser beam and the axis of a time-of-flight mass spectrometer (Comstock RTOF-210/LI). This instrument is a reflectron design and employs time lag focusing to improve mass resolution. The 193 laser beam is softly focused to retain molecular fragments; tight focusing produces only C1–C3 fragments.
RESULTS
BC Fraction of Flame Soot
Soot from the premixed ethylene–air and mini-CAST flames is examined as a function of height above the burner (HAB) and nitrogen fuel dilution, respectively. The results are presented in parallel to highlight the similarities in trends. Premixed flames are one-dimensional. HAB correlates linearly with time from start of combustion and, thereby, provides a time-line for soot evolution. The mini-CAST operates differently, namely, the diffusion flame is quenched at a fixed distance above the burner. Here, the increase in N2 fuel dilution flow reduces combustion time, while the concomitant decrease in O2 lowers reaction rates.
displays the changes in soot size distribution with HAB in the Φ = 2.05 ethylene premixed flame and with fuel dilution in the mini-CAST. With increased combustion time, the premixed flame soot particles grow in size and develop into a bimodal distribution. The smaller, nucleation, mode remains at a fixed mean diameter, and represents continued formation of nascent soot particles. The larger, accumulation, mode consists of soot agglomerates. This mode evolves to larger diameter and lower concentration as a consequence of particle coagulation. The CAST generally produces a single lognormal distribution of soot agglomerates, but consistent with previous work (Schnaiter et al. Citation2006), some conditions, such as CAST 3, include a small semivolatile nucleation mode.
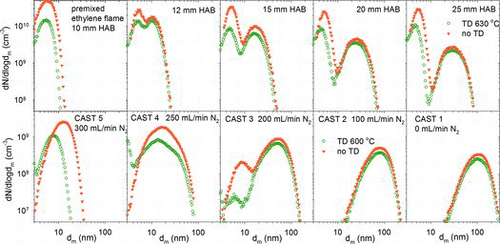
Also shown in are size distributions recorded after thermodesorption at ∼600°C. This has little impact on the premixed flame soot, other than losses in the thermodesorber and a small, ∼1 nm, decrease in nucleation mode diameter. The losses occur from thermophoresis, approximately size independent, and diffusion, which increases for small particles. Thermodesorber penetration is estimated from the ethylene flame data in to be about 75% above 20 nm and decreasing at lower size to ∼33% at 5 nm.
The situation is somewhat different for the mini-CAST. CAST 1, 2, and accumulation mode of 3 are unaffected by the thermodesorber. In contrast, the nucleation mode in CAST 3 is mostly removed, and the size distributions for CAST 4 and 5 shift to appreciably smaller size. Thus, the premixed flame soot appears to contain very little semivolatile material, possibly due to the immediate high dilution, whereas the mini-CAST soot contains both semivolatile and solid carbon depending on operating condition (Schnaiter et al. Citation2006; Mamakos et al. Citation2013).
(upper panel) shows that BC in the premixed flame measured by PASS significantly lags the soot mass recorded by quartz filter and calculated from SMPS size distributions. Filter collection and the SMPS data show soot formation to be complete by 20 mm HAB, whereas BC continues to grow. PM mass is derived from the SMPS accumulation mode using a previously reported soot effective density, , which assumed a material density ρ0 = 2.0 g/cm3, and provided primary particle diameters of d0 = 16, 23, and 26 nm, and mass-mobility exponents of Df = 2.2, 2.1, and 2.2 at 10, 15, and 20 mm above the burner (Maricq and Xu Citation2004). Values of d0 and Df for 12, 25, and 30 mm are extrapolated from these. These parameters are consistent with TEM images such as displayed by Figure S2, and the calculated masses agree well with quartz filter-based EC (and gravimetric PM in Figure S3).
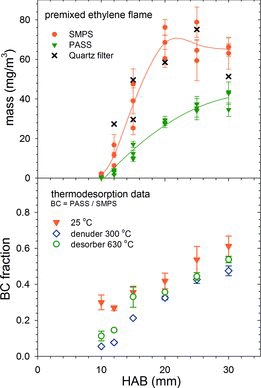
The BC fraction of accumulation mode mass displayed in the lower panel of increases with HAB, but remains well below unity, whether or not the soot is thermally treated. The small apparent decrease in BC upon thermal treatment could arise as a loss in the enhancement of optical absorption by lensing from an organic coating (Cappa et al. Citation2012); but, it might also represent measurement variability, such as exhibited in the top panel. These results suggest that low BC values do not originate from condensed organic material, but are characteristic of young soot. Increasing the equivalence ratio raises the rate of BC formation with HAB, as demonstrated in Figure S3.
The mini-CAST flames exhibit a similar BC trend with respect to propane fuel dilution. shows the BC fraction with and without thermal pretreatment to remove semivolatile material. Room temperature data are relative to gravimetric total PM mass. Between 300 and 1000 μg were collected to minimize gaseous filter adsorption artifacts. Two data sets are plotted to illustrate repeatability. BC fractions of thermally treated soot are based on SMPS-derived total PM mass assuming ρ0 = 2.0 g/cm3, Df = 2.3, and d0 = 18.3, 15.6, 12.7, 11, and 10 nm for CAST 1–5 flames (Figure S4). The BC fraction is high, >80%, for CAST flames 1 and 2, but regardless of evaporation of semivolatile material it falls steeply with increasing fuel dilution in flames 3–5. Removal of condensed semivolatile species substantially increases the BC fraction in the low BC flames, but it remains low, for example, the shift in CAST 5 size distribution in leads to a four-fold increase in BC fraction, but it remains well under 10%.
Particle ablation mass spectra of CAST 1 and 4 flames are compared in . CAST 1 and 2 spectra are nearly identical, as are those from CAST 3, 4, and 5. The positive fragment ions fall into three regions: a CxH+y series with x = 1 to >17 and y = 0 to 3, a region of PAHs, and a fullerene series from C34+ to C60+ (shown in insets). Under identical conditions of laser ablation, these spectra show significant differences. The PAH and fullerene fragment ions are absent from the C1 soot. And the fractions of hydrogenated CxH+y ions are significantly lower than for C4 soot. The latter is illustrated in , where the +'s display the fractions . This measure of “BC” exhibits qualitatively the same decrease with increasing fuel dilution as the PASS-derived data. Particle mass spectra of premixed ethylene flame soot are essentially the same as for CAST 4 (Maricq Citation2009). Flame conditions in that work correspond to those in the present work and thus have BC fractions below ∼60%, so mass spectra lacking PAH and fullerene series similar to CAST 1 soot were not observed.
TEM images reveal that low BC content is not limited to small nascent soot particles, but continues as the soot agglomerates. The images in are of particles size selected at 150, 100, 65, and 25 nm, respectively, from CAST flames 1–4 (the larger CAST 3 particle was collected as a result of being doubly charged). Whereas CAST 1 and 2 soots have BC fractions near unity, the BC content of CAST 3 falls to about 75%, even though the particles display typical soot agglomerate morphology. The mean diameter of CAST 4 soot is only about 25 nm, so these are multiples of only a few primary particles; however, their BC fraction is quite low, <20%. The BC fraction of premixed ethylene flame soot likewise remains low even as the particles grow into agglomerates. The 60 nm soot agglomerates in Figure S2 are sampled at 30 mm HAB from a Φ = 2.05 flame, where the BC fraction is ∼60%.
Engine Exhaust PM
BC and total PM mass emissions were measured over the federal test procedure (FTP) and supplemental (US06) drive cycles. They fall into the ranges of 0–1 mg/mi, 1–9 mg/mi, and 30–80 mg/mi for the PFI, GTDI, and diesel vehicles, respectively, depending on drive cycle (Figure S6). The BC fractions of total PM are displayed in . These are calculated in two ways: (1) PASS relative to gravimetric filter PM mass (thermal EC + OC for diesel tests) and (2) PASS relative to total mass recorded by DMM. Both methods reveal high BC content, 80–100%, for all three combustion processes, PFI, gasoline direct injection, and diesel. The >100% BC fractions for GTDI derived from DMM data are due to underestimation of total PM mass by the DMM. While this is indicative of uncertainty in instrumental measurement of PM mass, the filter data confirm the high, >80%, BC content. At low PM emission levels, filters have their own problems, namely, interference from gaseous adsorption. The filter measurements in are corrected for a 4 μg adsorption per filter. This has negligible impact on the diesel PM measurements where 200–900 μg of PM are collected, but is appreciable compared with the 2–20 μg collected for the PFI vehicle. In this case, measurement variability sometimes exceeds 100%, which explains the few missing filter-derived BC values in .
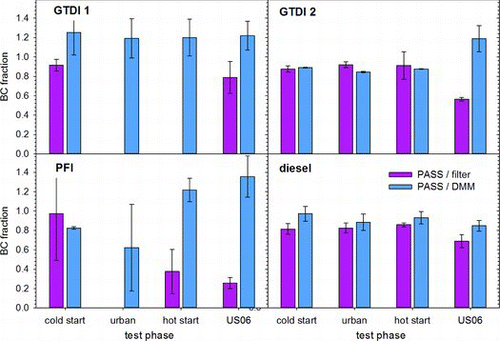
The high BC fraction in the PM from these vehicles suggests a combination of carbonized soot and low hydrocarbon/sulfate condensation. A low semivolatile content results from the three-way and oxidation catalysts present in the gasoline and diesel vehicle aftertreatment systems and low sulfur fuel; however, sampling conditions, for example, CVS system versus real world, can also play a role (Keskinen and Rönkkö Citation2010). The high BC content of the solid soot agglomerates from these engines distinguishes them from the soot produced by many of the flames above. Soot in the latter case was sampled relatively early in its life. This contrasts with the situation in engines, where soot experiences a longer time at near combustion temperature and high pressure. Soot produced in diesel engine cylinder, for example, remains in the hot exhaust gas after combustion stops and 90+% is oxidized as it mixes with the remaining oxygen. These conditions cause dehydrogenation and increase the graphitic nature of the soot and, thereby, its light absorption. With little or no oxygen left in gasoline exhaust, there is less soot oxidation, but carbonization can continue nonetheless to produce a high BC content.
DISCUSSION AND CONCLUSIONS
The present work confirms the earlier observation that BC content remains low relative to EC in mini-CAST flames even after removal of condensed organic material (Mamakos et al. Citation2013). Here, this is associated with nitrogen fuel dilution, where the lower oxygen concentration slows flame chemistry and the accompanying increased flow rate reduces residence time. However, other operating parameters may also lead to the formation of immature soot. The same phenomenon appears in premixed flames, where BC lags the growth in soot accumulation mode mass with HAB, and where the soot collected on filters correspondingly changes from brown to black. These are not due to condensed organic species, which thermodesorption measurements reveal to be negligible. Instead, the low BC content correlates with PAH and fullerene fragment ions, suggesting that these are solid agglomerate particles, but have lower light absorption because they have not yet fully carbonized (Dobbins Citation2009). In addition, the nucleation mode particles in these flames have been characterized as liquid-like nano-OC (Dobbins Citation2007, Citation2009; Sgro et al. Citation2008; D’Alessio et al. 2009), but these are not semivolatile in the conventional sense that they can be readily evaporated (as shown by ).
Carbonization has been reported to follow first-order Arrhenius kinetics, k = A exp(−Ea/kT), with an activation energy of Ea = 113 kJ/mole and pre-exponential factor of A = 1.8×106 s−1 that apply to a variety of fuels (Dobbins Citation2009, and references therein). This is thought to result from a common pathway via so-called stabilomer PAHs in the 200–300 amu range (). This kinetics is consistent with a longer time in the premixed ethylene flame promoting the observed increase in BC with HAB. It is also consistent with the typically higher temperatures of engine combustion relative to flames yielding a high BC content in vehicle exhaust solid particles.
As motor vehicle PM emissions have declined to meet increasingly stringent standards, there has been some expectation that the semivolatile fraction grows. The situation is complicated because catalytic aftertreatment has also improved and fuel sulfur levels have fallen, which likewise impact PM. The present work shows that current model vehicle PM is dominated by BC, about 80+%, for diesel, GTDI, and PFI gasoline technology, even at very low emissions (∼1 mg/mi) and for cold and hot operation. This is consistent with the recent study of eight light duty vehicles by Forestieri et al. (Citation2013) who found an average of 75% BC/total carbon. It suggests that differences in BC emission factors in the literature (Strawa et al. Citation2010; Liggio et al. Citation2012; Forestieri et al. Citation2013) may reflect regional differences in vehicle age/maintenance, or other factors, more than developments in engine/aftertreatment technology. The situation for PM from diesels equipped with properly functioning DPFs is somewhat different; results from the Advanced Collaborative Emissions Study (ACES) study of heavy duty engines show a mix of 53% sulfate, 30% OC, and 17% EC + elements (Khalek et al. Citation2011) because of the 99+% soot filtration. Such a high sulfur fraction is not expected for gasoline engines, where conversion of SO2 to sulfate is inefficient owing to the very low oxygen level in the exhaust. BC content is not only of interest with respect to motor vehicles, but also to aircraft. Recent work by Vander Wal et al. (Citation2014) reports a systematic variation in soot nanostructure from amorphous at low power to graphitic at high power, which likely means a concomitant variation from low to high BC.
Soot from CAST-type generators is also being used to help develop PM sensors for OBD (Malik et al. Citation2011). Present sensors rely on soot conductivity. Observations of anomalous sensor response have raised the question of whether conductivity, like optical absorption, might depend on soot generation conditions.
While there has been considerable progress in the development in instruments to measure PM emissions, there are very few good reference methods for their calibration. A major difficulty is the vast array of materials and properties that comprise PM. The European Union decision to adopt solid particle counting for vehicle emissions certification makes progress in this regard by narrowing the scope of PM to the non-volatile fraction, which is predominantly soot but may contain some ash, and by requiring calibration procedures. Flame soot is increasingly being looked to as a standard material (Giechaskiel et al. Citation2013), but as noted by Mamakos et al. (Citation2013), Schnaiter et al. (Citation2006), and the present work, care must be taken to match the flame-generated soot to the application. and demonstrate that soot from mini-CAST flames 1 and 2 matches engine exhaust soot sufficiently well for BC to serve as a useful surrogate for solid PM mass; in fact, AVL calibrates its Micro Soot Sensor with a CAST generator (Schindler et al. Citation2004). Use of a thermodenuder or catalytic stripper (Khalek and Bougher Citation2011) may be needed to remove semivolatile species, but is not sufficient. The generated soot must exhibit similar carbonization as the source soot being monitored. The present work suggests that this remains relatively constant for motor vehicle exhaust, but the sample size here is small. Further work is needed to see how broadly the present results extend over various engine-operating conditions and to other combustion sources.
SUPPLEMENTAL MATERIALS
Supplemental data for this article can be accessed on the publisher's website.
black_carbon_vs_soot_supplemental_info.zip
Download Zip (1.5 MB)ACKNOWLEDGEMENTS
The author is grateful to the help of Amy Harwell, Joseph Szente, Michael Loos, Adolfo Mauti, and Todd Wells with running the vehicle emissions tests.
REFERENCES
- Akard, M., Oestergaard, K., Chase, R.E., Richert, J.F. O., Fukushima, H., and Adachi, M. (2004). Comparison of an Alternative Particulate Mass Measurement with Advance Microbalance Analysis, SAE Technical Paper 2004-01-0589.
- Baumgardner, D., Popovicheva, O., Allan, J., Bernardoni, V., Cao, J., Cavalli, F., Cozic, J., Diapouli, E., Eleftheriadis, K., Genberg, P.J., Gonzalez, C., Gysel, M., John, A., Kirchstetter, T.W., Kuhlbusch, T.A. J., Laborde, M., Lack, D., Müller, T., Niessner, R., Petzold, A., Piazzalunga, A., Putaud, J.P., Schwarz, J., Sheridan, P., Subramanian, R., Swietlicki, E., Valli, G., Vecchi, R., and Viana, M. (2012). Soot Reference Materials for Instrument Calibration and Intercomparisons: A Workshop Summary with Recommendations. Atmos. Meas. Tech., 5:1869–1887.
- Bond, T.C., Doherty, S.J., Fahey, D.W., Forster, P.M., Berntsen, T., DeAngelo, B.J., Flanner, M.G., Ghan, S., Kärcher, B., Koch, D., Kinne, S., Kondo, Y., Quinn, P.K., Sarofim, M.C., Schultz, M.G., Schulz, M., Venkataraman, C., Zhang, H., Zhang, S., Bellouin, N., Guttikunda, S.K., Hopke, P.K., Jacobson, M.Z., Kaiser, J.W., Klimont, Z., Lohmann, U., Schwarz, J.P., Shindell, D., Storelvmo, T., Warren, S.G., and Zender, C.S. (2013). Bounding the Role of Black Carbon in the Climate System: A Scientific Assessment. J. Geophys. Res. Atmos., 118:5380–5552.
- Cappa, C.D., Onasch, T.B., Massoli, P., Worsnop, D.R., Bates, T.S., Cross, E.S., Davidovits, P., Hakala, J., Hayden, K.L., Jobson, B.T., Kolesar, K.R., Lack, D.A., Lerner, B.M., Li, S.-M., Mellon, D., Nuaaman, I., Olfert, J.S., Petäjä, T., Quinn, P.K., Song, C., Subramanian, R., Williams, E.J., and Zaveri, R.A. (2012). Radiative Absorption Enhancements Due to the Mixing State of Atmospheric Black Carbon. Science, 337:1078–1081.
- D'Alessio, A., D'Anna, A., Minutolo, P. and Sgro, L. A. (2009). Nanoparticles of Organic Carbon (NOC) Formed in Flames and their Effects in Urban Atmospheres, in Combustion Generated Fine Carbonaceous Particles, H. Bockhorn, A. D' Anna, A.F. Sarofim, H. Wang, eds., KIT Scientific Publishing, Karlsruhe, Germany, pp. 208–233.
- Dobbins, R.A. (2007). Hydrocarbon Nanoparticles Formed in Flames and Diesel Engines. Aerosol Sci. Technol., 41:485–496.
- Dobbins, R.A. (2009). Precursor Nanoparticles in Flames and Diesel Engines: A Review and Status Report, in Combustion Generated Fine Carbonaceous Particles, H. Bockhorn, A. D’Anna, A.F. Sarofim, and H. Wang, eds., KIT Scientific Publishing, Karlsruhe, Germany, pp. 191–205.
- Forestieri, S.D., Collier, S., Kuwayama, T., Zhang, Q., Kleeman, M.J., and Cappa, C.D. (2013). Real-Time Black Carbon Emission Factor Measurements From Light Duty Vehicles. Environ. Sci. Technol., 47:13104–13112.
- Ghazi, R., Tjong, H., Soewono, A., Rogak, S.R., and Olfert, J.S. (2013). Mass, Mobility, Volatility, and Morphology of Soot Particles Generated by a McKenna and Inverted Burner. Aerosol Sci. Technol., 47:395–405.
- Giechaskiel, B., Davok, R., Giovanella, K.M., Joergl, H., Diewald, R., and Schindler, W. (2013). Particle Generator (APG): A Soot Generator for the On-Site Checks of Particle Number (PN) Measurement Systems. JSAE Technical Paper 2013–5504.
- Giechaskiel, B., Maricq, M., Ntziachristos, L., Dardiotis, C., Wang, X., Axmann, H., Bergmann, A., and Schindler, W. (2014). Review of Motor Vehicle Particulate Emissions Sampling and Measurement: From Smoke and Filter Mass to Particle Number. J. Aerosol Sci., 67:48–86.
- Gothaniya, G., Lee, S.-Y., Menon, A.V., Iyer, S., Linevsky, M.J., Santoro, R.J., and Litzinger, T.A. (2009). A Study on the Effect of Experimental Setup Configuration on Soot Formation in a Laminar Premixed Ethylene-Air Flame, in Combustion Generated Fine Carbonaceous Particles, H. Bockhorn, A. D’Anna, A.F. Sarofim, and H. Wang, eds., KIT Scientific Publishing, Karlsruhe, Germany, pp. 353–372.
- Janssen, N.A. H., Gerlofs-Nijland, M.E., Lanki, T., Salonen, R.O., Cassee, F., Hoek, G., Fischer, P., Brunekreef, B., and Krzyzanowski, M. (2012). Health Effects of Black Carbon. World Health Organization.. http://www.euro.who.int/en/health-topics/environment-and-health/air-quality/publications/2012/health-effects-of-black-carbon
- Janssen, N.A. H., Hoek, G., Simic-Lawson, M., Fischer, P., van Bree, L., ten Brink, H., Keuken, M., Atkinson, R.W., Anderson, H.R., Brunekreef, B., and Cassee, F.R. (2011). Black Carbon as an Additional Indicator of the Adverse Health Effects of Airborne Particles Compared with PM10 and PM2.5. Environ. Health Perspect., 119:1691–1699.
- Keskinen, J., and Rönkkö, T. (2010). Can Real-World Diesel Exhaust Particle Size Distribution be Reproduced in the Laboratory? A Critical Review. J. Air Waste Manage. Assoc., 60:1245–1255.
- Khalek, I.A., and Bougher, T. (2011). Development of a Solid Exhaust Particle Number Measurement System Using a Catalytic Stripper Technology, SAE Technical Paper 2011-01-0635.
- Khalek, I.A., Bougher, T.L., Merritt, P.M., and Zielinska, B. (2011). Regulated and Unregulated Emissions from Highway Heavy-Duty Diesel Engines Complying with U.S. Environmental Protection Agency 2007 Emissions Standards. J. Air Waste Manage. Assoc., 61:427–442.
- Kittelson, D.B. (1998). Engines and Nanoparticles: A Review. J. Aerosol Sci., 29:575–588.
- Liggio, J., Gordon, M., Smallwood, G., Li, S.-M., Stroud, C., Staebler, R., Lu, G., Lee, P., Taylor, B., and Brook, J.R. (2012). Are Emissions of Black Carbon from Gasoline Vehicles Underestimated? Insights From Near and On-Road Measurements. Environ. Sci. Technol., 46:4819–4828.
- Liu, P., Ziemann, P.J., Kittelson, D.B., and McMurry, P.H. (1995). Generating Particle Beams of Controlled Dimensions and Divergence: I. Theory of Particle Motion in Aerodynamic Lenses and Nozzle Expansions. Aerosol Sci. Technol., 22:293–313.
- Malik, A., Abdulhamid, H., Pagels, J., Rissler, J., Lindskog, M., Nilsson, P., Bjorklund, R., Jozsa, P., Visser, J., Spetz, A., and Sanati, M. (2011). A Potential Soot Mass Determination Method from Resistivity Measurement of Thermophoretically Deposited Soot. Aerosol Sci. Technol., 45:284–294.
- Mamakos, A., Khalek, I., Giannelli, R., and Spears, M. (2013). Characterization of Combustion Aerosol Produced by a Mini-CAST and Treated in a Catalytic Stripper. Aerosol Sci. Technol., 47:927–936.
- Mamakos, A., Ntziachristos, L., and Samaras, Z. (2006). Evaluation of the Dekati Mass Monitor for the Measurement of Exhaust Particle Mass Emissions. Environ. Sci. Technol., 40:4739–4745.
- Maricq, M.M. (2004). Size and Charge of Soot Particles in Rich Premixed Ethylene Flames. Combust. Flame 137:340–350.
- Maricq, M.M. (2009). An Examination of Soot Composition in Premixed Hydrocarbon Flames via Laser Ablation Particle Mass Spectrometry. J. Aerosol Sci., 40:844–857.
- Maricq, M.M., and Xu, N. (2004). The Effective Density and Fractal Dimension of Soot Particles from Premixed Flames and Motor Vehicle Exhaust. J. Aerosol Sci., 35:1251–1274.
- Mikkanen, P., Moisio, M., Keskinen, J., Ristimäki, J., and Marjamäki, M. (2001). Sampling Method for Particle Measurements of Vehicle Exhaust, SAE Technical Paper 2001-01-0219.
- Ng, I.P., Ma, H., Kittelson, D., and Miller, A. (2007). Comparing Measurements of Carbon in Diesel Exhaust Aerosols using the Aethalometer, NIOSH method 5040, and SMPS, SAE Technical Paper 2007-01-0334.
- NIOSH (1999). Method 5040 Issue 3: Elemental Carbon (Diesel Exhaust). NIOSH Manual of Analytical Methods. National institute of Occupational Safety and health, Cincinnati, OH .
- Petzold, A., Ogren, J.A., Fiebig, M., Laj, P., Li, S.-M., Baltensperger, U., Holzer-Popp, T., Kinne, S., Pappalardo, G., Sugimoto, N., Wehrli, C., Wiedensohler, A., and Zhang, X.-Y. (2013). Recommendations for Reporting “Black Carbon” Measurements. Atmos. Chem. Phys., 13:8365–8379.
- Schindler, W., Haisch, C., Beck, H.A., Niessner, R., Jacob, E., and Rothe, D. (2004). A Photoacoustic Sensor System for Time Resolved Quantification of Diesel Soot Emissions, SAE Technical Paper 2004-01-0968.
- Schnaiter, M., Gimmler, M., Llamas, I., Linke, C., Jäger, C., and Mutschke, H. (2006). Strong Spectral Dependence of Light Absorption by Organic Carbon Particles Formed by Propane Combustion. Atmos. Chem. Phys., 6:2981–2990.
- Sgro, L.A., Borghese, A., Speranza, L., Barone, A.C., Minutolo, P., Bruno, A., D’anna, A., and D’alessio, A. (2008). Measurements of Nanoparticles of Organic Carbon and Soot in Flames and Vehicle Exhausts. Environ. Sci. Technol., 42:859–863.
- Snelling, D.R., Smallwood, G.J., Liu, F., Gulder, O.L., and Bachalo, W.D. (2005). A Calibration-Independent Laser-Induced Incandescence Technique for Soot Measurement by Detecting Absolute Light Intensity. Appl. Opt., 44:6773–6785.
- Stettler, M.E. J., Swanson, J.J., Barrett, S.R. H., and Boies, A.M. (2013). Updated Correlation Between Aircraft Smoke Number and Black Carbon Concentration. Aerosol Sci. Technol., 47:1205–1214.
- Strawa, A.W., Kirchstetter, T.W., Hallar, A.G., Ban-Weiss, G.A., McLaughlin, J.P., Harley, R.A., and Lunden, M.M. (2010). Optical and Physical Properties of Primary on-Road Vehicle Particle Emissions and Their Implications for Climate Change. J. Aerosol Sci., 41:36–50.
- US Environmental Protection Agency (2012). Air Quality Planning and Standards, Regional Haze Program. . http://www.epa.gov/visibility/program.html
- Vander Wal, R.L., Bryg, V.M., and Huang, C.-H. (2014). Aircraft Engine Particulate Matter: Macro- Micro- and Nanostructure by HRTEM and Chemistry by XPS. Combust. Flame, 161:602–611.
- Wang, H. (2011). Formation of Nascent Soot and Other Condensed-Phase Materials in Flames. Proc. Combust. Inst., 33:41–67.
- Watson, J.G. (2002). Visibility: Science and Regulation. J. Air Waste Manage. Assoc., 52:628–713.
- Xu, F., Sunderland, P.B., and Faeth, G.M. (1997). Soot Formation in Laminar Premixed Ethylene/Air Flames at Atmospheric Pressure. Combust. Flame, 108:471–493.