Abstract
The aerosol performance of engineered porous particles (PulmoSphere™) for inhalation as a function of powder properties (particle size and density) was assessed using an idealized replica of the adult human upper respiratory tract (URT) known as the Alberta mouth-throat model. Engineered placebo powders were prepared using the PulmoSphere™ technology, which is based on spray-drying an emulsion feedstock and produces porous particles with well-controlled size and density. These placebo powders are useful surrogates for a class of potent active formulations, and covered a range of particle sizes and densities, representing a particle design space relevant to dry powder inhalers. The Alberta idealized mouth-throat model was used for in vitro measurement of oropharyngeal (mouth and throat) losses and to estimate the total lung dose for different inhalation drug products. The in vitro lung doses measured with the mouth-throat model were compared to predictions of lung dose from semi-empirical numerical models of oropharyngeal deposition. Data from the mouth-throat model and numerical models were used to rank order oropharyngeal losses and flow-rate dependence in the 1–6 kPa pressure drop range. Aerosol performance of the PulmoSphere™ powders was favored by low-particle density and large geometric size, with the oropharyngeal deposition and flow rate dependence being lower for powders with median particle diameter ≥2.5 microns. In comparison, data from lactose-blend formulations showed significantly higher oropharyngeal deposition and flow rate dependence. The idealized mouth-throat model provides a reasonable in vitro estimate of dose delivered to the lungs, and is a useful tool for studying the effect of factors such as drug/device and inhalation airflow.
Copyright 2014 American Association for Aerosol Research
INTRODUCTION
Particle deposition in the mouth and throat pose a great challenge to the dose delivery performance of inhaled medications. The geometry of the human airway is complex and variable (DeHaan and Finlay Citation2001), deposition is influenced not only by physiological differences in size, but also time-dependent changes associated with patient breathing maneuver, and factors related to the inhaler, such as the flow resistance, and mouthpiece geometry and position (McRobbie et al. Citation2003; Pritchard and McRobbie Citation2004; McRobbie and Pritchard Citation2005). Other factors including aerosol concentration and polydispersity, and environmental factors such as humidity, can also affect the delivery of inhaled medication to the target airways (DeHaan and Finlay Citation2001). Understanding the effect of these factors on product performance and prediction of delivered lung doses, ahead of clinical trials, is of great utility to product designers and developers, and has therefore attracted considerable attention in the past decades.
Oropharyngeal deposition of inhaled aerosols has been studied in depth by Stahlhofen, and more recently by Finlay and others (Stahlhofen et al. Citation1989; DeHaan and Finlay Citation2001; Longest et al. Citation2008; Olsson et al. Citation2008; Delvadia et al. Citation2012; Golshahi et al. Citation2013). Stahlhofen et al. (Citation1989) compiled data from multiple studies showing that oropharyngeal deposition increases with increasing values of d2Q, where d is the aerodynamic particle size and Q the volumetric flow-rate. This relationship indicates that inertial impaction is the dominant factor in determining oropharyngeal deposition. Finlay and coworkers further showed that a better correlation of oropharyngeal losses is achieved for pharmaceutical aerosols when the characteristic length scales of the inhaler mouthpiece (Finlay et al. Citation2002) as well as the mouth-throat geometry (Grgic et al. Citation2004) are considered, for example, via a dimensionless parameter, the Stokes number. These studies provided justification for the use of predictive numerical models of oropharyngeal losses based on semiempirical correlations. Inputs to these models included inhalation airflow, inhaler mouthpiece geometry, airway geometry, as well as the aerodynamic particle size distribution of the delivered aerosol.
The aerodynamic particle size distributions (APSD) of inhalation aerosols are typically measured using an inertial impactor such as the Next Generation Pharmaceutical Impactor (NGI) with induction port and preseparator per USP <601>. Prediction of lung deposition based on stage groupings of APSD data, such as a fine particle dose fraction post induction port and preseparator, is complicated and often poses a challenge, and while identifying a suitable fine particle mass (FPM) marker could be justified via correlation with in vivo lung deposition, such data are often not available. Moreover, the size distribution of the aerosol delivered from the inhaler is often incompletely characterized, due a significant unsized fraction lost in the induction port. The incomplete size characterization of the delivered dose could potentially bias the estimation of in vitro lung dose. An alternative approach in estimating an in vitro lung dose would be to use a physical model of the idealized mouth-throat model. The benefits of this approach include (i) a simple and direct in vitro measurement of lung dose, that is expected to capture the physics of particle transport and deposition in the human mouth-throat and (ii) the ability to measure aerosols generated under clinically relevant conditions, via the use of realistic patient flow profiles during actuation of an inhaler. Olsson et al. (Citation2013) recently showed good in vitro in vivo correlation with the use of an anatomic throat model to assess nine different inhaler products.
To date, most publications on dry powder inhalers (DPI) have been focused on the delivery performance of blend formulations where small drug crystals (<2 μm) are attached to large lactose carrier particles with size >50 μm (Podczeck Citation1998). There are relatively few published studies on engineered powders intended for inhalation. Such powders are typically characterized by low bulk-powder density (<0.2 g·cm−3) and primary particles having geometric diameter ≅2 μm, respectively (Weers et al. Citation2007). It is of interest to investigate the dose delivery performance of engineered powders, as exemplified by the low density porous particles produced using the PulmoSphere™ technology. Low density engineered powders have enabled the development of DPI products capable of delivering large lung doses via improvement in powder fluidization and dispersibililty. Studies with PulmoSphere™ powder formulations have demonstrated that it is possible to achieve high lung deposition (>50% of dose) that is largely independent of inspiratory flow-rate (Duddu et al. Citation2002; Hirst et al. Citation2002; Newhouse et al. Citation2003; Weers et al. 2007).
The aim of this study was to use the idealized mouth-throat model to characterize the dose delivery performance of DPI products based on engineered powder and lactose-blend formulations. The idealized mouth-throat model provides an in vitro estimate of the lung dose, which is compared to in vitro markers based on semiempirical models of oropharyngeal deposition.
MATERIALS AND METHODS
Powder Formulations and Inhaler Systems
summarizes different devices and formulations investigated in this study. PulmoSphere™ placebo powder formulations were prepared by spray-drying an emulsion of perflubron (PFOB; Allied, lot #101800, USA) in water, stabilized by distearoylphosphatidylcholine (DSPC; Lipoid, lot #103605, Germany) and calcium chloride (CaCl2; Sigma Alrich, lot #103936, USA). The spray-drying process eliminates water and PFOB, and produces porous particles of DSPC/CaCl2, representing the key excipients in PulmoSphere™ formulations. The goal was to produce a panel of low-mass density porous particles having median geometric diameters in the range of 1.5–5.0 μm and tap densities of 0.03–0.15 g·cm−3. Particle sizes and densities were modulated by varying feedstock parameters such as the ratio of PFOB to water (0.04–0.87 w/w) and total solids (1.0–6.0% w/v), and process parameters such as air-to-liquid ratio (5–10 w/w) and drying kinetics (total gas flow 90–160 scfm, dryer outlet temperature maintained at 70°C). The rationale for studying PulmoSphere™ placebo powders is that they are useful surrogates for a class of potent active formulations with low drug loading (<10% w/w). The incorporation of such small amounts of drug does not greatly affect the bulk powder properties, and consequently the aerosolization behavior. The PulmoSphere placebo powders were all filled into foil-foil blisters (fill mass of 2 mg), designed for actuation with the Simoon™ inhaler (Device A).
TABLE 1 Dry powder inhaler and formulations used in the present study
Two marketed DPI products, Spiriva® and Asmanex®, were also evaluated in this study as products representative of lactose-based formulations. Asmanex is an inhaled corticosteroid for asthma treatment, in which the micronized drug (mometasone furoate) is combined with micronized excipient (lactose) and formulated into large agglomerates to facilitate handling in the bulk state (Yang et al. Citation2001). The drug is delivered using a Twisthaler multidose reservoir device, and is available in two strengths, 220 μg and 110 μg of mometasone furoate (Package Insert). The therapeutic agent in Spiriva is tiotropium bromide, which is a long-acting (24-h) muscarinic receptor antagonist also known as an antimuscarinic or anticholinergic agent for treatment of reversible airways obstruction or chronic obstructive pulmonary disease (COPD). The inhalable formulation consists of tiotropium bromide blended with lactose monohydrate carrier particles (Package Insert; Chodosh et al. Citation2001). Each Spiriva capsule contains 18 μg tiotropium (22.5 μg of inhalable tiotropium bromide monohydrate).
Determination of Geometric Primary Particle Size of Bulk Powder
The geometric primary particle size distribution of PulmoSphere™ placebo powder was measured with a Sympatec HELOS Type BF Model Laser Light Diffraction Analyzer (Sympatec GmbH, Germany) with the RODOS-M (OASIS) dry disperser and the ASPIROS powder dosing unit. The instrument evaluation mode was set to high resolution laser diffraction (HRLD), which returns size distributions based on Fraunhofer diffraction theory. Powder samples of 5–15 mg of powder was placed into a 1-mL vial and loaded into the ASPIROS dosing unit set at a speed of 25 mm·s−1. The injector and primary pressure settings for the RODOS dry disperser were 4 mm and 4 bar, respectively. Measurements were performed using the R2 lens (particle size range of 0.45–87.5 μm). The RODOS settings were selected after verifying that they achieved essentially complete dispersion of the PulmoSphere powder down to the primary particles formed during the spray-drying process. Results are reported here in terms of the mean volume median diameter, X50. Repeatability tests of this method with representative powders (total 9 lots) have demonstrated relative standard deviation <3% with triplicate samples.
Tapped Density Analysis of Bulk Powder
The tapped density of the PulmoSphere™ placebo formulations was measured by dispensing bulk powder into a 0.593 cubic centimeter cylindrical cavity, doctoring off excess powder, and manually tapping 3–5 times on a hard surface, while adding powder to compensate for powder settling. The cavity is placed onto a weighing pan for gravimetric analysis (Mettler Toledo AX206, US). Assessment of method precision was performed for low (0.041 g·cm−3), medium (0.065 g·cm−3), and high (0.122 g·cm−3) density powders with six replicates for each lot and the relative standard deviations were 2.8%, 6.7%, and 4.5%, respectively. Other powder lots were based on duplicate samples. Results are reported as mean tapped density.
Scanning Electron Microscopy
Samples of the powder formulations were viewed under a scanning electron microscope using a Philips XL 30 Environmental Scanning Electron Microscope (ESEM; Philips Electron Optics, USA). A thin layer of powder was placed on a 1 cm × 1 cm of silicon wafer disk (Omnisil, VWR IBSN3961559, USA) for engineered particles or on a 12-mm diameter carbon adhesive tape for lactose-based powders (Electron Microscopy Sciences, Cat. #77825-12, USA), and the sample was prepared for electron microscopy by sputter-coating a thin gold and palladium film (Denton, 21261 Cold Sputter/Etch and DTM-100, operated at <100 mTorr and 30–42 mA for 100–150 s). Samples were then loaded into the ESEM chamber and the filament current and accelerating voltage set to 1.6 A and 20 kV, respectively.
In Vitro Mouth Throat Model
The mouth-throat model was used for in vitro measurement of estimate total lung dose for devices and formulations listed in . The mouth-throat model used in this study was developed by Finlay and coworkers at the University of Alberta, Canada. They combined medical imaging technology, e.g., CT/MRI scans, and particle aerodynamics data to construct an idealized upper airway model whose aerosol deposition characteristics matched the average in vivo deposition data (Stapleton et al. Citation2000; DeHaan and Finlay Citation2001). In the present study, the anatomical throat model was fabricated out of aluminum using CAD geometry data provided by Professor Finlay. For ease of use, the model was fabricated in two parts, corresponding roughly to the “mouth” and “throat” sections of the airway. This two-staged construction of the throat allows some qualitative assessment of regional deposition in the upper airway. Suitable mouthpiece adapters were designed and customized for each inhaler for attachment to the mouth-throat model, so that the inhalers were oriented approximately horizontally with respect to the mouth-throat model.
Semi-Empirical Correlations for Estimating Lung Dose
Experimental data from the idealized mouth-throat model were compared to two proposed in vitro markers derived from cascade impactor measurements of aerosol size. The first proposed in vitro marker for lung deposition is based on a fine particle mass (FPM) corresponding to a selected cutoff value of the inertial impaction parameter, d2Q, where d is the aerodynamic diameter (μm) and Q is volumetric airflow (L·min−1).
Thus, an in vitro estimate of lung dose can be obtained from standard cascade impactor measurements as , where C is a suitable cutoff value. Note that this in vitro FPM marker factors in both particle size and inhaler flow-rate, and can be used to compare the performance of inhalers having different flow resistances (hence tested at different flow-rate), or of the same inhaler over a range of flow-rates. A cutoff value of C ∼ 1450 μm2 L·min−1 corresponds to a mean oropharyngeal deposition of ∼50% according to the model of Stahlhofen et al. (Citation1989) and also represents ∼50% deposition in the Alberta mouth-throat model for monodisperse aerosols introduced through a straight tube (DeHaan and Finlay Citation2001). For convenience, cutoff values in this study are selected based on NGI stage groupings, e.g., the mass fraction accumulated on stages 4 to multiorifice collector (MOC) corresponding approximately to
, and mass on stages 3 to MOC, corresponding approximately to
. Flow-rate effects on inhaler dose delivery performance are thus assessed on the basis of fixed impactor stage groupings (essentially fixed d2Q), rather than a fixed diameter cutoff alone as is customarily and incorrectly done, Clark and Hollingworth (Citation1993).
A second measure of lung dose is derived from a semiempirical correlation for estimating mouth-throat losses proposed by DeHaan and Finlay (Citation2004). It is based on a Stokes number that incorporates the inhaler mouthpiece geometry as a length scale. The fraction of the delivered dose deposited in the mouth and throat is estimated from the following:[1] where St is the Stokes number given by
[2] ρp is particle density, g·cm−3, U0 is fluid velocity, cm·s−1, dp is particle diameter, μm, μ is fluid dynamic/kinematic viscosity, kg·cm−1·s−1, d is aerodynamic diameter with the density of water, μm, Q is volumetric airflow, L·min−1, and dm is the inhaler mouthpiece diameter, cm.
In EquationEquation (1)[1] , the first term represents losses in the mouth and is significant for inhalers having small mouthpiece diameters. The second term represents laryngeal losses according to Stahlhofen et al. (Citation1989). The above semiempirical correlation, EquationEquation (2)
[2] , has been implemented in the form of an online calculator, ARLA Respiratory Deposition Calculator (CitationMartin and Finlay 2008). Note that DeHaan and Finlay (DeHaan and Finlay Citation2001, 2004; Finlay et al. Citation2002) developed their correlation using experimental data with the Alberta mouth-throat model, so it is reasonable to compare semiempirical model predictions with results from the Alberta throat for the DPI products tested in this study.
The fraction of delivered dose deposited in the lung may be evaluated as the fraction penetrating through the mouth-throat:[3] where the mouth-throat loss fraction is obtained from EquationEquation (1)
[1] . Inputs to the ARLA calculator include the inhaler mouthpiece dimensions, the inhaler flow rate, and the corresponding APSD of the delivered aerosol, which is modeled as having a log-normal distribution, and specified in terms of the mass median aerodynamic diameter (MMAD) and geometric standard deviation (GSD). For each of the inhalation products investigated, these APSD inputs were obtained by in vitro measurement of the delivered aerosol dose using the Next Generation Impactor (MSP Corp., USA). Aerosol characterization methods are described below.
In Vitro Characterization of Aerosol Dose Delivery Performance
In vitro dose delivery performance was investigated over a range of pressure drops (1–6 kPa) considered representative of the range achievable by asthma and COPD patient populations (Al-Ahowair et al. Citation2007). Aerosol dose delivery tests were performed using a square-wave flow-rate profile generated using a timer-controlled vacuum source. A single inhalation actuation was conducted for each inhaler type. Test attributes included the (i) emitted powder mass (EPM), (ii) in vitro lung dose (LD), and (iii) aerodynamic particle size distribution (APSD) using the NGI cascade impactor. Note that tests (i) and (iii) are standard in vitro tests per USP <601>. A 2-L sampling volume was maintained for each dose actuation for EPM and LD analyses. However, for NGI analysis, a 4-L volume was used to account for the large internal dead volume (2025 mL) of the NGI apparatus (Copley et al. Citation2005), including the induction port, preseparator and NGI body.
The test fixture used for EPM measurement is shown schematically in . When actuated with flow, the aerosolized dose leaving the inhaler mouthpiece is collected onto a 47-mm diameter filter (A/E type, Pall Corp., USA). A custom filter holder designed for gravimetric analysis of engineered powder was used for testing Device A. Standard DUSA tubes were used for collecting doses from Devices B and C. It should be noted that the gravimetric analysis approach is not commonly used in aerosol dose delivery testing for lactose blend formulations, but is entirely appropriate for engineered powder formulations. Engineered powders are reasonably homogeneous in composition, and the distribution of drug (when present) closely follows the distribution of powder mass. The results for EPM are reported in terms of % of label claim (Devices B and C) or fill mass (Device A).
FIG. 1. Emitted powder mass measurement set-up with a customized emitted dose powder collector for Device A and using DUSA tube for Devices B and C.
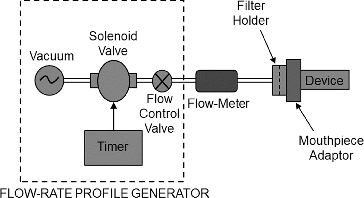
For determination of in vitro lung dose fraction analysis, the test inhaler was coupled to the inlet of the idealized mouth-throat model, and the dose penetrating through the model was collected downstream on a 76-mm diameter filter (A/E type, Pall Corp., USA), as shown in . A polysorbate (EMD Chemicals, Cat. #8170072, USA) wetting agent (equal parts of Tween 20 and methanol, v/v) was used for coating the interior walls of the cast model to prevent particle reentrainment. The procedure for applying coating solution to the mouth-throat model was as follows: (a) ∼15 mL of the coating solution was dispensed into the mouth-throat model, which was then capped at both ends; (b) the solution was allowed to wet the internal walls of the mouth-throat model using a rocking or rotary motion to tilt the mouth-throat model from side to side; (c) excess solution was allowed to drain for 5 min before use. After five dose actuations, the mouth-throat model was rinsed with lukewarm water, air dried, and a fresh coating applied before the next use.
FIG. 2. Cross-sectional view of the idealized mouth-throat model with an inhaler and filter housing attached upstream and downstream, respectively.
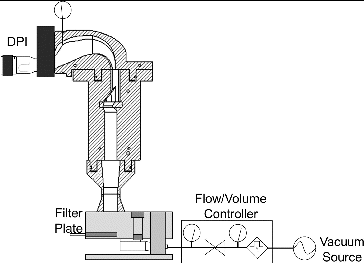
For determining the APSD, the NGI (Apparatus 5 in USP <601>), was used with the Induction Port + Pre-Separator + NGI Body. As noted earlier, due to their homogeneous composition, the PulmoSphere™ placebo powders may be assayed using a gravimetric approach. To enable gravimetric analysis, the gravimetric NGI cups were fitted with 55-mm diameter glass fiber filters (A/E type, Pall Corp, USA) and the preseparator upper and lower compartments were coated 1 and 2 mL, respectively, with a polysorbate wetting agent (equal parts of Tween 20 and methanol, v/v).
For the lactose-based formulations, drug-specific HPLC assays were used to quantitate Mometasone (with Device C) and Tiotropium (with Device B). The collection substrates of the NGI along with the preseparator were coated with polysorbate solution: 1 mL for small cups and 2 mL for preseparator and large cups.
Mometasone content was determined using a reverse phase-high performance liquid chromatograph (RP-HPLC) method on a Dionex Ultimate 3000 system (Thermo Scientific Dionex, USA) equipped with a photodiode array (PDA) detector set to 238 nm. Tiotropium content was determined on the same system using PDA detector set to 254 nm. Chromatographic separation was attained using an YMC-Pack ODS-AQ (50 × 4.6 mm, 3 μm) column at a flow-rate of 0.6 and 1.0 mL·min−1 (50 and 30 μL injection volume) for tiotropium and mometasone, respectively. Mobile Phase A for tiotropium method was composed of 75% v/v water mixed with 25% acetonitrile and 0.1% phosphoric acid, and water with 0.1% trifluoroacetic acid for mometasone. The diluent used to dissolve drug samples before HPLC analysis for tiotropium and mometasone were 25% acetonitrile mixed with 75% water and 42% acetonitrile mixed with 58% water, respectively. Drug recoveries for both compounds were ≥97%.
NGI Data Analysis
Aerodynamic particle size distribution results for all drug products were expressed in the form of a fine particle mass (FPM,% of label claim, or fill mass) with cutoff d2Q < 500 μm2·L·min−1, which represents the cumulative mass deposited on stages 4 to MOC (or filter) of the NGI. The corresponding airflows and effective cutoff diameters (ECD) are summarized in . Note that APSD results were also used as inputs to the ARLA calculator for estimating total lung dose, as described later.
TABLE 2 Stage cut-off diameters for NGI stage 3 at test conditions
RESULTS AND DISCUSSION
presents the bulk powder properties of spray-dried PulmoSphere™ powders. The results show the powder properties fall within the target values of 0.03–0.15 g·cm−3 for tapped density and 1.5–5.0 μm for primary particle size, thus allowing a range sufficiently wide to study the effects of these parameters. As noted earlier, the placebo powders (A–F) are useful surrogates for a class of potent active formulations with low drug loading, since incorporation of small amounts of drug (<10%) does not greatly affect the bulk powder properties or consequently the aerosolization behavior. An example of this is shown in , where active PulmoSphere formulations (G, H) with 2% and 6% Indacaterol (Novartis AG, Switzerland) had powder properties comparable to that of placebo formulations (A–C), which were processed within a similar range of conditions. It should be cautioned that the results for the Placebo powders studied here may not necessarily be representative of active formulations with significantly higher drug loading, or of performance in other inhalers.
TABLE 3 Bulk powder properties of spray-dried PulmoSphere™ formulations
Representative photomicrographs from SEM analyses are presented in for the three formulation types investigated in this study. SEM images show three distinct particle morphologies for the formulation types. The PulmoSphere™ powder (A) has porous, roughly spherical particles, in the respirable size range (1–5 μm). The tiotropium formulation (B) has large angular carrier particles (>20 μm) onto which are adhered small micron-sized particles, presumably of drug.
FIG. 3. Three particle morphologies analyzed by scanning electron microscopy: (a) spray-dried PulmoSphere™ (Batch A), (b) traditional lactose-blend with tiotropium bromide, and (c) spheronized mometasone furoate.
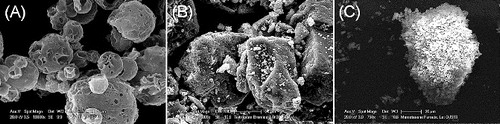
presents a correlation between bulk powder properties and in vitro aerosol performance from EPM and NGI data for the six PulmoSphere™ placebo powders (A–F) when tested with Device A at a pressure drop of 4 kPa. indicates a strong effect of tap density, with smaller tap density resulted in higher emitted powder mass fraction.
FIG. 4. Effect of tapped density and primary particle size on in vitro aerosol performance: (a) tapped density as function of emitted powder mass (N = 10) and (b) primary particle size, X50, as function of fine particle mass (N = 3) accumulated mass from stage 3 to MOC.
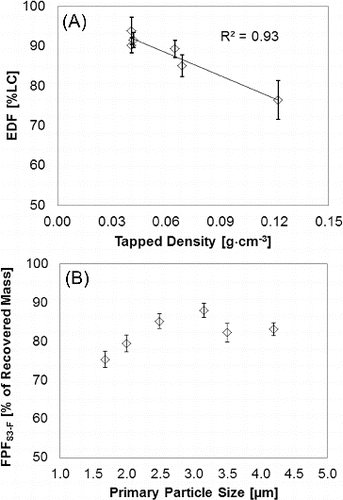
A one-way ANOVA analysis of the six PulmoSphere™ powders showed a significant difference in EPM among the lots (p < 0.0001). A post-hoc means comparison test (Tukey-Kramer HSD) showed that the three low density powders (A, B, and C) were similar, but significantly different from the two medium density powders (D and E), and the high density (F) powder batch.
The effect of primary particle size on the FPM (% of mass recovered from cups) of the dispersed aerosol is shown in . The results show that the FPM initially increases with X50 and then appears to reach a plateau for X50 ≥ 2.5 μm, and therefore suggesting greater cohesive forces with smaller particles. At first glance, it may appear counter-intuitive for the FPM to show an increasing trend with X50. It should be kept in mind, however, that X50 represents a size metric for bulk powder, which is essentially completely dispersed to primary particles using compressed air at pressures up to 4 bar. The FPM, on the other hand is a measure of the incompletely deagglomerated aerosol delivered by the Device A, where the powder is dispersed under relatively gentle conditions. The formation of aerosol agglomerates from the bulk powder is determined by the balance between “cohesive” inter-particle forces and the “dispersive” fluid hydrodynamic forces, both of which vary with primary particle size in a complex manner, resulting in the relationship plotted in .
summarizes the mass median aerodynamic diameter (MMAD) and geometric standard deviation (GSD) derived from the NGI measurement for each inhalation product. The MMAD & GSD values were estimated based on the aerosol fraction deposited in the stages downstream of the throat and preseparator. The drug mass distributed on the stages (often termed the impactor sized mass, ISM) were transformed to log probability coordinates, and best fit estimates of MMAD and GSD obtained, per USP <601>. The MMAD and GSD values so obtained were used as inputs to the ARLA Respiratory Deposition Calculator, along with input parameters identified in . The output from the ARLA calculator represents the calculated lung dose, reported here as a fraction of the ISM, and also as a fraction of the label claim/fill mass.
TABLE 4 NGI analysis data and mean
TABLE 5 ARLA calculator selection input parameters, all others set to default mode
In using these MMAD/GSD estimates to represent the aerosol delivery from the inhaler, it is implicitly assumed that the coarse aerosol fraction prefiltered by the USP induction port + Preseparator is deposited in the mouth-throat, and therefore does not contribute to lung dose. This assumption is justified based on experimental data on particle capture efficiency of mouth-throat model versus USP induction port (Zhou et al. Citation2011), and has been verified in a sensitivity analysis (not reported here).
In addition to the aerosol size distribution, the ARLA calculator also requires the diameter of the inhaler mouthpiece to be specified as an input. As seen in , the inhaler mouthpiece exits have a complex shape, and based on the internal geometry, an “effective” diameter was estimated for each inhaler and used as an input to the ARLA online calculator. For example, the effective diameter of Device A was based on the diameter of the internal flow passage issuing into the mouthpiece.
Idealized Mouth-Throat Model Data Analysis
The in vitro lung dose fraction was measured experimentally using the idealized mouth-throat model at pressure drops ranging from 1–6 kPa. presents the measured in vitro lung dose fraction as a function of inhaler pressure drop for the six PulmoSphere™ placebo powder formulations tested using Device A. For median primary particle size ≥2.5 μm, the data show consistently a low oropharyngeal deposition (hence higher lung deposition) that is independent of primary particle size and flow-rate. However, for particle sizes <2.5 μm, lung deposition is significantly decreased, with greater variability and flow-rate dependence of primary particle size. These results suggest that the optimal geometric size range for PulmoSphere™ placebo powders delivered using Device A is ≥2.5 μm. Below this size, inter-particle attractive forces are greater than drag and lift forces generated in the inhaler. This negatively impacts powder fluidization and dispersion. The data in represent the same device with six different variants of the PulmoSphere™ placebo powder. The data clearly demonstrate that the properties of the PulmoSphere™ powder can be tailored to achieve significant improvements in dose delivery for a given device.
FIG. 6. In vitro lung dose performance as measured from the mouth-throat model for PulmoSpheres™ delivered by Device A as a function of mean primary particle size, tested at 1–6 kPa inhaler pressure drops.
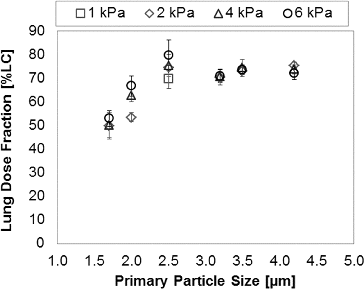
TABLE 6 Mean and standard deviation of in vitro aerosol delivered dose for different formulation platforms
summarizes in vitro aerosol performance data from the idealized mouth-throat model for the three inhalation powder types studied. One of the six PulmoSphere™ powders (primary particle size of 2.49 μm and tapped density 0.041 g·cm−3) was used to generate results with Device A. The results show that the in vitro lung dose for this PulmoSphere™ powder when delivered with Device A is greater than that of the other two products. The PulmoSphere™ data presented in and are consistent with recent studies that suggest that a drug/device combination comprising of PulmoSphere™ powder and blister-based inhaler enable consistent and high delivery efficiency performance over a range of patient inspiratory flow-rates (Weers et al. Citation2012, Citation2013).
Comparing Mouth-Throat Model and Numerical Models
is a summary of in vitro markers of lung dose from the idealized mouth-throat model and semiempirical models of mouth-throat deposition accounting for inertial impaction due to the effects of airflow (Q), aerodynamic particle size of the dispersed powder, and inhaler mouthpiece dimension. Data are presented as estimated in vitro aerosol delivered dose as percent of label claim or fill mass, along with airflows and corresponding pressure drops for each formulation and inhaler platform. The PulmoSphere™ powder shows a slight increase in lung dose with increasing airflow, but EquationEquation (3)[3] predicts a small drop in lung dose across the range of airflow tested. In contrast, Device B shows strong flow-rate dependence across the range of airflows tested. Measured lung doses using the mouth-throat model are significantly higher than the values predicted from the semiempirical model. It should be noted that the internal flow path geometry within the mouthpiece of many inhalers and assumptions regarding the “effective” exit diameter of the inhaler mouthpiece could bias prediction of inhaler exit flow velocities and the associated oropharyngeal deposition (Cardwell et al. Citation2014). Despite this, the in vitro mouth-throat model and oropharyngeal numerical model predict a similar trend and performance ranking for the different aerosol delivery platforms. The experimentally measured in vitro lung dose (using the idealized mouth-throat model) also correlates with the values predicted using the inertial impaction parameter, d2Q (). Interestingly, the correlation is better using the prediction at d2Q < 1300 μm2·L·min−1 (i.e., NGI stages 3 to MOC) as compared with d2Q < 500 μm2·L·min−1 (i.e., NGI stages 4 to MOC). This observation is consistent with the cutoff value of ∼1450 μm2·L·min−1 reported for the mouth-throat model for monodisperse aerosols (DeHaan and Finlay Citation2001).
CONCLUSIONS
The idealized mouth-throat model is an effective tool for assessing the design space for engineered particles and can be used to tailor engineered powders for a specific DPI system. In this study, application of this tool showed that for porous PulmoSphere™ powders delivered with Device A, enhanced delivery to the lung is favored by low particle density and large geometric size. In addition, the idealized mouth-throat model provides a simple and direct method to compare the dose delivery performance among different types of inhalation products, covering a broad range of device and powder technologies, and operating over a range of inhalation flow-rates. In this study, the idealized mouth-throat model was used to compare the performance of engineered powders with that of lactose-based formulations over a clinically relevant range of inhaler pressure drops (1–6 kPa). The results showed that the engineered powders ranked higher in in vitro measures of lung dose compared to the lactose-based products. These findings are consistent with predictions based on cascade impactor data for the same products. Although the in vitro lung dose derived from the idealized mouth-throat model are higher than that predicted from the semiempirical correlations, the results from all the approaches rank-order the products in a similar manner. Potential advantages of the idealized mouth-throat model over standard cascade impactor sizing tools (e.g., NGI, ACI) are: (i) direct measurement of in vitro lung dose, (ii) less analytical burden, and (iii) allowing the use of realistic breath profiles, which have not been considered in this study.
ACKNOWLEDGMENTS
The authors thank Daniel Huang, John Le, and Esther Hui for their assistance in facilitating this research.
The authors also thank Professor Warren Finlay, from the University of Alberta, Canada, for providing CAD data for the Alberta throat geometry in 2003.
REFERENCES
- Al-Ahowair, R. A. M., Tarsin, W. Y., Assi, K. H., Pearson, S. B., and Chrystyn, H. (2007). Can All Patients with COPD Use the Correct Inhalation Flow with all Inhalers and Does Training Help? Resp. Med., 101:2395–2401.
- Asmanex® Twisthaler®, Package Insert for Use.
- Cardwell, N. D., Zee, J., Kadrichu, N. P., Rao, N. P., and Clark, A. R. (2014). Flow Field Characterization of Three Dry Powder Inhalers. Respir. Drug Deliv., 501–505.
- Chodosh, S., Flanders, J. S., Kesten, S., Serby, C. W., Hochrainer, D., and Witek, T. J. (2001). Effective Delivery of Particles with the HandiHaler Dry Powder Inhalation System over a Range of Chronic Obstructive Pulmonary Disease Severity. J. Aerosol Med., 14(3):309–315.
- Clark, A. R., and Hollingworth, A. M. (1993). The Relationship Between Powder Inhaler Resistance and Peak Inspiratory Conditions in Healthy Volunteers—Implications for In Vitro Testing. J. Aerosol Med., 6(2):99–110.
- Copley, M., Smurthwaite, M., Roberts, D. L., and Mitchell, J. P. (2005). Revised Internal Volumes of Cascade Impactors for Those Provided by Mitchell and Nagel. J. Aerosol Med., 18(3):364–366.
- DeHaan, W. H., and Finlay, W. H. (2001). In Vitro Monodisperse Aerosol Deposition in a Mouth and Throat with Six Different Inhalation Devices. J. Aerosol Med., 14:361–367.
- DeHaan, W. H., and Finlay, W. H. (2004). Predicting Extrathoracic Deposition from Dry Powder Inhalers. Aerosol Sci., 35:309–331.
- Delvadia, R. R., Longest, P. W., and Byron, P. R. (2012). In Vitro Tests for Aerosol Deposition. I: Scaling a Physical Model of the Upper Airways to Predict Drug Deposition Validation in Normal Humans. J Aerosol Med., 25(1):32–40.
- Duddu, S. P., Sisk, S. A., Walter, Y. H., Tarara, T. E., Trimble, K. R., Clark, A. R., et al. (2002). Improved Lung Delivery from a Passive Dry Powder Inhaler Using an Engineered PulmoSphere® Powder. Pharmaceut. Res., 19:689–695.
- Finlay, W. H., DeHaan, W., Grgic, B., Heenan, A., Matida, E. A., Hoskinson, M., et al. (2002). Fluid Mechanicals and Particle Deposition in the Oropharynx: The Factors that Really Matter. Respir. Drug Deliv. VIII, 171–177.
- Golshahi, L., Noga, M. L., Vehring, R., and Finlay, W. H. (2013). An In Vitro Study on the Deposition of Micrometer-Sized Particles in the Extrathoracic Airways of Adults During Tidal Oral Breathing. Ann. Biomed. Eng., 41(5):979–989.
- Grgic, B., Finlay, W. H., and Heenan, A. F. (2004). Regional Aerosol Deposition and Flow Measurements in an Idealized Mouth and Throat. J. Aerosol Sci., 35:21–32.
- Hirst, P. H., Pitcairn, G. R., Weers, J. G., Tarara, T. E., Clark, A. R., Dellamary, L. A., et al. (2002). In Vitro Lung Deposition of Hollow Porous Particles from a Pressurized Metered Dose Inhaler. Pharmaceut. Res., 19:258–264.
- Longest, P. W., Hindle, M., Choudhuri, S. D., and Xi, J. (2008). Comparison of Ambient and Spray Aerosol Deposition in a Standard Induction Port and More Realistic Mouth—Throat Geometry. J. Aerosol Sci., 39(7):572–591.
- Marple, V. A., Olson, B. A., Santhanakrishnan, K., Mitchell, J. P., Murray, S. C., and Hudson-Curtis, B. L. (2003). Next Generation Pharmaceutical Impactor (A New Impactor for Pharmaceutical Inhaler Testing). Part II: Archival Calibration. J. Aerosol Med., 16(3):301–324.
- Marple, V. A., Olson, B. A., Santhanakrishnan, K., Roberts, D. L., Mitchell, J. P., and Hudson-Curtis, B. L. (2004). Next Generation Pharmaceutical Impactor: A New Impactor for Pharmaceutical Inhaler Testing. Part III. Extension of Archival Calibration to 15 L/min. J. Aerosol Med., 17(4): 335–343.
- Martin, A. R., and Warran, F. H. (2008). An Online Calculator for Predicting Respiratory Deposition of Inhaled Aerosols. Respir. Drug Deliv., Book 2, 801–805. www.mece.ualberta.ca/arla/aerosoldepositioncalculator_adult.html
- McRobbie, D. W., and Pritchard, S. (2005). Studies of Human Oropharygneal Airspaces Using Magnetic Resonance Imaging. II. The Effects of Device Resistance with Forced Maneuver and Tidal Breathing on Upper Airway Geometry. J. Aerosol Med., 18:325–336.
- McRobbie, D. W., Pritchard, S., and Quest, R. A. (2003). Studies of Human Oropharyngeal Airspaces Using Magnetic Resonance Imaging. I. Validation of a Three-Dimensional MRI Method for Producing Ex Vivo Virtual and Physical Casts of the Oropharyngeal Airways During Inspiration. J. Aerosol Med., 16:401–415.
- Newhouse, M. T., Hirst, P. H., Duddu, S. P., Walter, Y. H., Tarara, T. E., Clark, A. R., et al. (2003). Inhalation of a Dry Powder Tobramycin PulmoSphere Formulation in Healthy Volunteers. Chest., 124:360–366.
- Olsson, B., Borgström, L., Lundbäck, H., and Svensson, M. (2013). Validation of a General In Vitro Approach for Prediction of Total Lung Deposition in Healthy Adults for Pharmaceutical Inhalation Products. J. Aerosol Med., 26:1–15.
- Olsson, B., Borgstrom, L., Svensson, M., and Lundback, H. (2008). Modeling Oropharyngeal Cast Deposition to Predict Lung Delivery from Powder Inhalers. Respir. Drug Deliv., 197–205.
- Podczeck, F. (1998). The Relationship Between Physical Properties of Lactose Monohydrate and the Aerodynamic Behavior of Adhered Drug Particles. Int. J. Pharmaceut., 160:119–130.
- Pritchard, S., and McRobbie, D. W. (2004). Studies of Human Oropharyngeal Airspaces using Magnetic Resonance Imaging. II. The Use of Three-Dimensional Gated MRI to Determine the Influence of Mouthpiece Diameter and Resistance of Inhalation Devices on the Oropharyngeal Airways Geometry. J. Aerosol Med., 17:310–324.
- Spiriva® HandiHaler®, Package Insert for Use.
- Stahlhofen, W., Rudof, G., and James, A. C. (1989). Intercomparison of Experimental Regional Aerosol Deposition Data. J. Aerosol Med., 2:285–308.
- Stapleton, K. W., Guentsch, E. Hoskinson, M. K., and Finlay, W. H. (2000). On The Suitability of k-e Turbulence Modeling for Aerosol Deposition in the Mouth and Throat: A Comparison with Experiment. J. Aerosol Sci., 31:739–749.
- Weers, J. G. (2001). Dispersible Powders for Inhalation Applications. Innov. Pharmaceut. Technol., 111–116.
- Weers, J. G., Maltz, D. S., Ung, K., Chan, L., Glusker, M., Ament, B., et al.. (2012). Minimizing Human Factors Effects through Inhaler Design. Respir. Drug Deliv., 217–226.
- Weers, J. G., Tarara, T. E., and Clark, A. R. (2007). Design of Fine Particles for Pulmonary Drug Delivery. Expert Opin. Drug Deliv., 4:297–313.
- Weers, J. G., Ung, K., Le, J., Rao, N., Ament, B., Axford, G., et al. (2013). Dose Emission Characteristics of Placebo PulmoSphere® Particles Are Unaffected by a Subject's Inhalation Maneuver. J. Aerosol Med., 26(1):56–68.
- Yang, T. T., Li, S., Wyka, B., and Kenyon, D. (2001). Drug Delivery Performance of the Mometasone Furoate Dry Powder Inhaler. J. Aerosol Med., 14:487–494.
- Zhou, Y., Sun, J., and Cheng, Y. (2011). Comparison of Deposition in the USP and Physical Mouth-Throat Models with Solid and Liquid Particles. J, Aerosol Med Pulm Drug Deliv., 24(3):277–284.