Abstract
Bipolar diffusion charging is used routinely in aerosol electrical mobility size distribution measurements. In this study, aerosol charge fractions produced by six bipolar chargers (neutralizers) were measured using a tandem differential mobility analyzer system. Factors that were studied include the type of ion source (210Po, 85Kr, 241Am, and soft X-ray), source activity, charger design, and aerosol flowrate. It was found that all six types of neutralizers achieve stationary state charge distributions when the source activity is sufficiently high. For 210Po neutralizers with an initial radioactivity of 18.5 MBq (0.5 mCi), stationary state charge distributions are achieved when the source is less than 3.25 years old (residual activity no less than 0.0527 MBq). Stationary state was achieved for 85Kr neutralizers having residual radioactivity greater than 70 MBq. Source activities of 241Am and soft X-ray neutralizers are discussed. Aerosol charge fractions for six neutralizers remain reasonably invariant over a wide range of flowrates. The positive charge fractions achieved by the soft X-ray neutralizer are higher than those by the other five neutralizers using radioactive sources while negative charge fractions for all neutralizers studied are all in a similar range. This study also raises questions about bipolar charging fractions used for data inversion in some scanning mobility particle spectrometer (SMPS) systems, and underscores the need to better understand bipolar charging to achieve more accurate measurements of particle size distributions.
Copyright 2014 American Association for Aerosol Research
1. INTRODUCTION
Bipolar diffusion charging (exposing aerosol particles to both positive and negative ions) is used to bring aerosols to stationary state charge distributions independent of their initial charge state (Cooper and Reist Citation1973; Liu and Pui Citation1974a; Adachi et al. Citation1985; Reischl et al. Citation1996). Knowledge of these stationary state charge distributions is especially important for instruments such as the scanning mobility particle spectrometer (SMPS), which rely on accurate knowledge of size-dependent charge fractions for data inversion (Wang and Flagan Citation1990; Stolzenburg and McMurry Citation2008).
Fuchs’ limiting sphere diffusion charging theory with corrections by Hoppel and Frick is widely used to predict the stationary state charge distributions achieved by bipolar chargers (Fuchs Citation1963; Hoppel and Frick Citation1986). This aerosol charging model was tested using measured aerosol charge fractions (Adachi et al. Citation1985; Wiedensohler et al. Citation1986; Reischl et al. Citation1996), though different ion properties in these studies were often used to achieve good agreement between experimental results and theoretical predictions. For aerosol exposed to soft x-rays and/or high temperature, this model was extended to account for simultaneous diffusion charging, direct photoionization, and thermionization (Jiang et al. Citation2007a,Citationb). Fuchs’ diffusion charging model needs to be solved numerically. Through fitting predicted charge fractions by Fuchs diffusion charging theory for particles up to two charges, an approximation formula for a fixed stationary state charge distribution was proposed (Wiedensohler Citation1988). Together with an analytical expression for estimating fractions of particles with three charges or more (Gunn and Woessner Citation1956), it allows rapid calculation of aerosol bipolar charge distribution. Though definite assumptions about ion properties (mass, mobility and concentration) were made when deriving this formula, it has been widely used in different electrical mobility particle spectrometers for data inversion (Wiedensohler et al. Citation2012). However, Gopalakrishnan et al. (Citation2013) reported that this formula noticeably underestimates the fractions of charged particles when compared to results from a Brownian Dynamics analysis. In addition, López-Yglesias and Flagan (Citation2013) found that assumptions made by Hoppel and Frick in estimating ion flux to particles limits the accuracy of Fuchs’ theory. They proposed corrections to the current diffusion charging model and approximation formulas to estimate different aerosol stationary state charge distributions for two types of ions. These updates remain to be experimentally evaluated.
Different ionization methods have been used to generate bipolar ions for aerosol charging, e.g., corona ionization, radioactive sources, and soft X-ray. Corona discharge is a conventional technique used to generate ions for aerosol charge neutralization (Whitby and Peterson Citation1965; Romay et al. Citation1994; Stommel and Riebel Citation2005; Kimoto et al. Citation2009). However, formation of contaminant particles and ozone was reported for corona neutralizers (Liu et al. Citation1987; Romay et al. Citation1994). In addition, bipolar corona neutralizers often suffer from the difficulty of maintaining a balance of positive and negative ions (Romay et al. Citation1994; Stommel and Riebel 2004). Radioactive sources (e.g., 210Po, 85Kr, and 241Am) are most commonly used to produce bipolar ions because of their simplicity and effectiveness (Cooper and Reist Citation1973; Liu and Pui Citation1974a; Reischl et al. Citation1996). In recent years, however, the tight safety regulations on using and transporting radioactive sources promoted the search for alternative ionization methods, such as soft X-ray (Shimada et al. Citation2002), micro-plasma (Kwon et al. Citation2006), and dual electrode corona (Han et al. Citation2009; Qi and Kulkarni Citation2013). Radioactive sources, soft X-ray, and corona discharge are all currently used in different commercial aerosol neutralizers.
Aerosol charge fractions have been measured using bipolar diffusion chargers with different radioactive sources such as 241Am (Hussin et al. Citation1983; Adachi et al. Citation1985; Reischl et al. Citation1996; Vivas et al. Citation2008), 210Po (Wiedensohler et al. Citation1986; Maricq Citation2008), and 85Kr (Liu and Pui Citation1974b; Covert et al. Citation1997). However, the effect of radioactive source activity on charge fractions has not been systematically examined. In the last few years, a soft X-ray neutralizer became commercially available (Model 3087, TSI Inc., USA). Charging ratios and particle generation were evaluated in a prototype soft X-ray neutralizer which used the opposite flow direction and different housing designs from the commercial unit (Shimada et al. Citation2002; Yun et al. Citation2009). In addition, the commercial unit has an aluminum foil installed to attenuate soft X-ray intensity. Though the ion properties of the TSI 3087 neutralizer (Kallinger et al. Citation2012) and its performance in neutralizing electrosprayed bioaerosol (Modesto-Lopez et al. Citation2011) were studied, the aerosol charge fractions downstream of this soft X-ray neutralizer have not been reported or compared with other neutralizers. In addition, Hoppel and Frick (Citation1990) showed that the charge ratio was affected by neutralizer design and aerosol flowrate.
In this study, we aim to examine the effects of neutralizer design, ionization method, source activity, and flowrate on aerosol charge fractions. Six neutralizers with different designs and/or different ionization methods were investigated. We used the tandem differential mobility analyzer (TDMA) technique to measure the bipolar fractions of singly- and doubly-charged monodisperse particles. Different source activities and flowrates were tested. Measured charge fractions were compared with those reported by other studies and values predicted by Wiedensohler's approximation formula.
2. METHODOLOGY
The TDMA system for measuring the single and double charge fractions of initially neutral particles is shown in . Polydisperse dioctyl sebacate acid (DOS) particles were generated by atomizing 0.01% DOS solution with isopropyl alcohol (IPA) as the solvent. Diffusion dryers with activated carbon were used to remove IPA. A dilution bridge was used to adjust aerosol concentration. Polydisperse DOS aerosol was neutralized by a fresh 210Po neutralizer. The peak diameter of the DOS size distribution downstream of the atomizer at the above solution concentration was below 70 nm. Most measurements in this study were done with 70 nm DOS particles, helping to reduce the effect of multiply charged particles during mobility classification in DMA1. Particles selected by DMA1 were sent via either the test route or a bypass route to DMA2 for a TDMA scan. A sheath flowrate to aerosol flowrate ratio of 10:1 was used for both DMAs (Model 3071, TSI Inc., USA) with open loops for the sheath and excess flows of each, i.e., filtered compressed air was provided as the sheath air (Rader and Mcmurry, Citation1986). With an open loop for DMA1, the carrying gas through test neutralizers is primarily compressed air and less influenced by the solvent used in generating aerosols through atomization. The DMA2 input and output aerosol concentrations were measured by upstream and downstream CPCs (Model 3772, TSI Inc., USA).
FIG. 1. Experimental setup for measuring the charge distribution of initially neutral monodisperse particles. The aerosol and sheath flowrates for both DMAs were maintained at 1 and 10 lpm, respectively. A TSI 3080 platform was used to control test flowrates through neutralizers (0.3 to 15 lpm): for flowrates >1 lpm, the flow direction is as shown in the figure; for 1 lpm, the blower is turned off; for flowrates <1 lpm, the flow extraction and injection points are switched from what is shown in the figure.
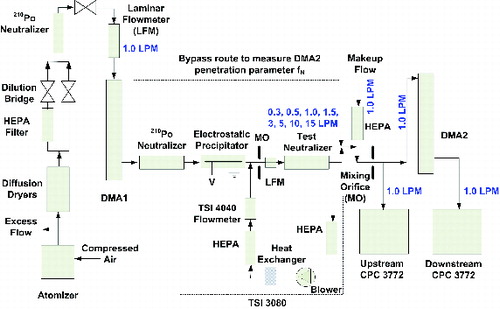
To measure the fraction of singly and doubly charged particles from a test neutralizer, 70 nm particles selected by DMA1 were first neutralized by a fresh 210Po neutralizer. The remaining charged particles were then removed by an electrostatic precipitator (ESP) which can completely remove charged particles below ∼150 nm when 1 kV is applied at 1 lpm (liter per minute). The remaining neutral particles were then charged by the test neutralizer. A TSI 3080 platform was used to control the flowrate through the test neutralizer ranging from 0.3 to 15 lpm. For flowrates greater than 1 lpm, the flow direction was as shown in ; for 1 lpm, the 3080 blower was turned off; and for lower flowrates, the flow extraction and injection points were switched from what is shown in the figure. Different from Adachi et al. (Citation1985), an ion trap was not used downstream of test neutralizers to represent the installations typical for SMPS systems. Prior to the upstream CPC, 1 lpm makeup flow was added. Orifices were used when makeup flows were added to ensure complete mixing.
For a TDMA scan, the DMA2 voltage was stepped to measure the singly and doubly charged particle distributions. The DMA2 output aerosol concentration versus voltage was measured by the downstream CPC 3772. With the TDMAFIT software (Stolzenburg and McMurry Citation1988), the fraction of singly and doubly charged particles can then be determined. This program fits the forward calculation of the TDMA response to the measured downstream concentrations (normalized by the upstream concentration) using nondiffusing transfer function theory and adjustable parameters fN, fβ, and fV. The parameter fN accounts for aerosol depositional losses in DMA2. The parameter fβ accounts for the ratio of the measured to expected width of the mobility distribution exiting DMA1, assuming nondiffusing transfer functions. The parameter fV is the ratio of the DMA1 centroid mobility set by its voltage to that measured by DMA2. At the beginning of the experiment, TDMA measurements using the bypass route provided values of DMA2 performance parameters fN0, fβ0, and fV0 equal to 1.01 ± 0.02, 1.26 ± 0.01, and 0.98 ± 0.01, respectively. The DMA2 penetration parameter fN0 was used to correct the raw measured fN values to give the true measured charge fractions. Diffusional broadening of the DMA transfer functions only accounts for about 20% of the extra width represented by fβ0 = 1.26 but this degradation of resolution should not affect the other results.
Figure S1 (in the online supplementary information) shows the dimensions of the six neutralizer housings tested in this study, i.e., the 210Po housing of Particle Technology Lab (PTL) at University of Minnesota; the 210Po housing of Aerosol Dynamics Inc. (ADI); the TSI 3077/3077A 85Kr housing, the TSI 3012/3012A 85Kr housing, the 241Am housing of Tokyo Dylec Corporation, and the TSI 3087 soft X-ray housing. Test flowrates for the TSI 3012/3012A ranged from 3 to 15 lpm. For the other five neutralizers, test flowrates ranged from 0.3 to 5 lpm. Table S1 summarizes radioactive sources tested in this study. The half-lives of 210Po, 85Kr, and 241Am are 4.6 months, 128.6 months, and 432.2 years, respectively. 210Po and 85Kr radioactive sources with different activities were examined. The TSI 3077 and 3077A neutralizers have the same housing. The difference is that the 85Kr radioactive source in the 3077A neutralizer has higher initial activity than that in the 3077 neutralizer, i.e., 370 MBq vs. 74 MBq. The same is true for the TSI 3012 and 3012A neutralizers. For the TSI 3087 neutralizer, a fresh soft X-ray ionizer (Photoionizer L9491, Hamamatsu Photonics, Japan) was tested. To prevent potential particle generation inside the neutralizer due to soft X-ray photoionization (Yun et al. Citation2009), the TSI 3087 has a 0.4 mm aluminum foil installed to attenuate soft X-ray intensity. The particle concentration through the test neutralizer was maintained at 3000 particles/cm3 to avoid ion depletion in the test neutralizer. Experiments using various aerosol concentrations (100, 300, 500, 1000, 3000, and 5000 particles/cm3) were carried out for the PTL housing with a 64-month old 210Po source to evaluate the effect of aerosol concentration on charge fractions. No significant differences in charge fraction were observed over this concentration range.
3. RESULTS AND DISCUSSIONS
3.1. Effect of Ion Source Activity on Aerosol Charge Fractions
shows the effect of 210Po activity on singly charged fractions of 70 nm DOS particles for the PTL and ADI housings. Measurements were done for flowrates ranging from 0.3 to 5.0 lpm. While some dependence on flowrate is observed, especially for the ADI housing, source activities of 0.0527 MBq or greater (i.e., ages of 39 months or less) appear to be adequate to achieve stationary state charge distributions. Charged fractions are significantly below stationary state values for source activities less than 0.0075 MBq (>52 months) and approach zero for the 91-month-old source (∼20 half-lives). For sources with insufficient activity, the fractions also show a stronger dependence on the aerosol flowrate. Despite a few noticeable exceptions, the charge fractions at lower flowrates (longer residence times in the neutralizer) tend to be higher than those at higher flowrates (shorter residence time) when the source activity is less than 0.0527 MBq. At insufficient 210Po activities, ion concentrations generated by 210Po alpha radiation are not high enough to bring all particles to a stationary state charge distribution. Longer residence time will help more particles to get charged. The ADI 210Po neutralizer shows stronger fluctuations of the charge fractions at different flowrates which may be attributed to the housing design. Large deviations of the charge fractions at 1.5 lpm from those at other flowrates will be discussed in the next section.
FIG. 2. Singly charged fractions of 70 nm particles with the PTL ((a) negative; (b) positive) and ADI ((c) negative; (d) positive) neutralizer housings as a function of 210Po ion source activity and flowrate (0.3–5 lpm). The second x-axis illustrates the corresponding age (in months) of 210Po sources.
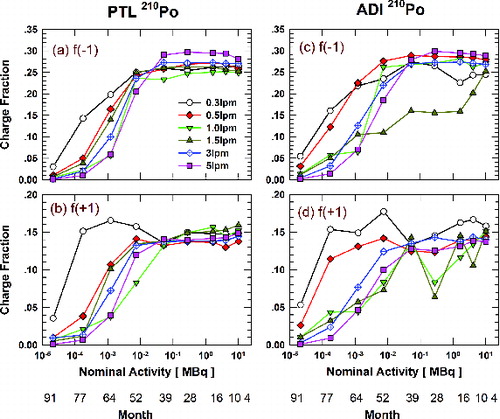
shows the effect of 85Kr activity on singly charged fractions for the TSI housings for a range of flowrates, again for 70 nm DOS particles. Since the age of the oldest 85Kr source tested in this study was only approximately one half-life (versus twenty half-lives for the oldest 210Po source tested, Table S1), the aging effect of the 85Kr source on the charge fractions is not as apparent as for the 210Po source. Again, the TSI 3077 and 3077A have the same housing, but different initial 85Kr radioactivity. Therefore, their results were combined in one plot (). The same is true for the TSI 3012 and 3012A (). Despite the variations of the charge fractions at different aerosol flowrates, the fractions of singly charged particles at the 85Kr activity of 37.3 MBq for the 3077/3077A neutralizers can be seen to deviate from those for 85Kr activities exceeding 70 MBq. As for the 3012/3012A, the fractions at the activity of 55.5 MBq also deviate from those for the activity higher than 70 MBq. These deviations are not as significant as those for 210Po results (). The age of the oldest 85Kr tested in this study was only approximately one half-life (∼10.6 year).
FIG. 3. Singly charged fractions of 70 nm particles with TSI 3077/3077A ((a) negative; (b) positive) and 3012/3012A ((c) negative; (d) positive) neutralizers as a function of 85Kr ion source activity and aerosol flowrate.
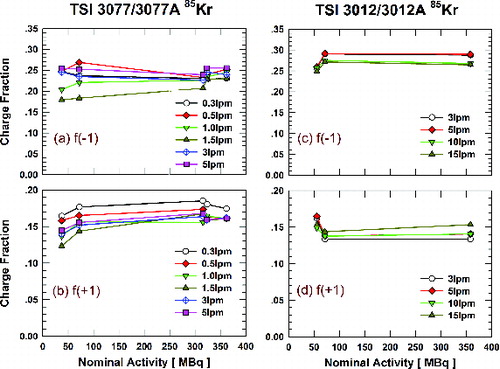
Nevertheless, shows that stationary state charge distributions are achieved by the 3077/3077A and 3012/3012A neutralizers when the 85Kr radioactivity is greater than about 70 MBq. Please note that this “threshold” 85Kr radioactivity is much higher than the “threshold” 210Po radioactivity, 0.0527 MBq. In addition to different neutralizer designs (e.g., different volumes of the charging chamber), this difference is also related to the difference in radioactive decay of 85Kr and 210Po. 85Kr emits beta particles (electrons) with continuous energy distribution up to 0.69 MeV, whereas 210Po and 241Am emit monoenergetic alpha particles (helium nuclei) with energy around 5 MeV (Knoll, Citation2010). Besides this difference in the energy available to create ions, the energy loss per distance traveled in air is far higher for alphas than for betas, so that the range is 1–3 meters for the betas and only a few centimeters for the alphas. This means the betas are stopped by the chamber walls before they can use all of their energy to create ions, resulting in approximately 500 times as many ions produced from a single alpha particle as from a single beta particle.
Given the long half-life of 241Am radioactive source, we did not test its aging effect on aerosol charge fractions. The initial radioactivity of the 241Am used in the test neutralizer is 3 MBq. For a 100-year old 241Am source, its radioactivity would be 2.55 MBq which is still significantly higher than the 39-month-old 210Po with an initial activity of 18.5 MBq. The soft X-ray source manufacturer (Hamamatsu Photonics, Japan) indicates that the guaranteed life for the soft X-ray source is 8000 hrs. Hamamatsu did not provide information how the soft X-ray intensity varies during its lifetime. Our test indicates that its intensity is reproducible within 4% during the course of 15-month continuous operation. Considering the increasing usage of the soft X-ray neutralizer in many countries, a longer aging effect on its performance needs to be addressed in future studies.
3.2. Effect of Aerosol Flowrate on Aerosol Charge Fractions
shows the dependence of singly charged fractions of 70 nm DOS particles on flowrate for the PTL and ADI housings averaged over measurements for all tested 210Po sources with the residual activity no less than 0.0527 MBq. These results reinforce the conclusion from that charged fractions are only weakly dependent on flowrate over the range of conditions tested, except for the anomalous result for the ADI housing at 1.5 lpmFootnote1 . Figure S2 indicates that unstable flow occurs inside this neutralizer at flowrates around 1.5 lpm (Wang et al. Citation2007). Figure S3 shows the flowrate dependence of singly charged fractions for the other sources, i.e., the TSI 85Kr sources, the TSI soft x-ray source, and the Dylec 241Am source. Again, as was observed for 210Po, charged fractions depend only weakly on flowrate, although a small decrease in charged fractions was observed for the TSI 3077/3077A neutralizer at the flowrate of 1.5 lpm (Figure S3a). Figure S4 shows the dependence of doubly charged fractions on flowrate for all sources studied. For most neutralizers, the relative standard deviation of the singly charged fraction at different flowrates is within 6%. For the doubly charged fraction, the relative standard deviation is larger but still within 13% for most neutralizers. Averaged values of charged fractions and uncertainties at 1 lpm are shown in .
TABLE 1 Charge fractions of 70 nm DOS particles achieved using different ion sources (210Po, 85Kr, 241Am, and soft X-ray) at the flowrate of 1 lpm
FIG. 4. Singly charged fractions of 70 nm particles with different neutralizers as a function of the aerosol flowrate: (a) PTL 210Po neutralizer; (b) ADI 210Po neutralizer. Charge fractions are averaged for all tested 210Po sources with the activity of 0.0527 MBq or greater. The error bars are the standard deviations. Numbers given on the plots are the mean charge fraction for all flowrates and the standard deviation (in parenthesis), respectively.
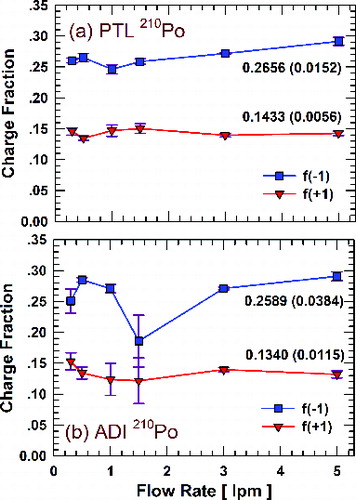
Although charged fractions are only weakly dependent on flowrate, the differences between negative and positive charge fractions tend to increase with increasing aerosol flowrate above a certain value except for the TSI 3012/3012A. In addition, negative charge fractions tend to increase with increasing flowrate while positive charge fractions show the opposite trend. These trends are observed for both singly and doubly charged fractions, though they are more apparent for doubly charge fractions. No clear correlation between the fraction of neutral particles and the aerosol flowrate was observed for any of the neutralizers. A similar effect of singly charged fractions on flowrate was observed previously when neutralizing 45 nm oil particles using an ADI 210Po neutralizer (Maricq, Citation2008), though the flowrates used in that study were lower.
There are differences between the mean charge fractions achieved by different neutralizers. For instance, the positive charge fractions by the TSI 3087 soft x-ray neutralizer are higher than those by the other five neutralizers while their negative charge fractions are all in a similar range. In addition, positive charge fractions (both singly and doubly) by the 3087 soft X-ray neutralizer are very close to negative charge fractions at low aerosol flowrates, i.e., 0.3 and 0.5 lpm. The difference between them increases significantly with increasing aerosol flowrate. Jiang et al. (Citation2007a) reported that direct photoionization charging can occur for soft X-ray chargers given that the photon energy of soft X-ray is ∼9.5 keV, which is approximately three orders of magnitude higher than the particle ionization work function (the energy barrier that must be overcome by an electron to escape from the particle surface). Direct photoionization would increase the fractions of positively charged particles. This effect would be stronger when the flowrate is lower, leading to longer particle residence time in the ionization zone. Kallinger et al. (Citation2012) reported that the mobility of negative ions produced by soft X-ray ionization is lower than those produced by radioactive source and corona discharge while the mobility of positive ions is similar among three methods. This can also contribute to the observed higher fractions of particles with positive charges.
Bipolar charging is a rather dynamic process. Though aerosol stationary state charge distributions can be achieved when the product of ion concentration and residence time is higher than a certain value (Liu and Pui Citation1974a), these stationary distributions are affected by positive and negative ion properties and the ratio of positive to negative ion concentrations. The unequal mobilities of positive and negative ions can lead to a changing balance in ion concentrations downstream of the ion generation zone (Hoppel and Frick Citation1990). Although negative and positive ions are treated as two discrete species in current aerosol charging models, their masses and mobilities have distributions which can change inside and downstream of the neutralizer. For instance, Luts et al. (2011) reported that the mobility distribution and composition of negative air ions produced by 241Am sources changes as they age from a few tenths of a second to ∼20 s. This will result in variations in stationary state charge distributions. Therefore, understanding the observed variations and differences in charge fractions requires detailed analysis of the flow patterns inside and downstream of neutralizers, ion production, loss, concentrations, and properties (mobility and mass distributions), and particle concentration which is beyond the scope of this study. Nevertheless, the empirical observations made in this study provide uncertainty ranges when using these six neutralizers at different flowrates.
3.3. Charge Fraction Comparison
Charge fractions measured in this study were compared to the widely used approximation formula of Wiedensohler (Citation1988). As shown in , measured f(−1) values for four sources are in reasonable agreement with the predicted values, i.e., the difference ranges from 4.6% for 210Po to 11.0% for 241Am. For positively charged particles, measured values of f(+1) for the soft X-ray source are in good agreement with the approximation formula, while they are significantly lower than predicted for the other three sources, i.e., the difference range is from 18.4% for 85Kr to 25.2% for 210Po. This discrepancy remains if charged fractions are found by averaging over the range of values measured for different flowrates (as shown in Figures 4 and S3). Additional experiments were done for 210Po, 85Kr, and 241Am neutralizers using 70 nm sodium chloride particles (replacing DOS/IPA solution in the atomizer with NaCl/H2O solution and the activated carbon in diffusion dryers with silica gel). Measured charge fractions are similar to those using DOS particles and the discrepancy in f(+1) values remains.
To further examine the difference in positive charged fractions, charge fractions measured by different studies along with those predicted by the approximation formula (Hussin et al. Citation1983; Adachi et al., Citation1985; Wiedensohler et al. Citation1986; Wiedensohler Citation1988; Reischl et al. Citation1996) are shown in . In order to evaluate the effect of different particle sizes for charge fractions, additional experiments using the PTL housing and a 4-month-old 210Po source were carried out with classified particles of 50, 100, and 130 nm at 1 lpm. The data in cover particle sizes ranging from 3 to 130 nm. Again, f(−1) values measured in this study are in reasonable agreement with predicted f(−1) values and those measured by Wiedensohler et al. (Citation1986), whereas f(+1) values for three radioactive sources measured in this study are noticeably lower (). As particle size decreases, the charge fraction drops significantly which makes the absolute difference between measured and predicted values difficult to distinguish on these plots. A logarithmic scale has often been used when comparing measured and predicted charged fractions in the literature, which leads to an impression of relatively good agreement between them (the same would be true for if a logarithmic scale was used for the y-axis). However, c shows that for the large size range of 3 to 130 nm, the approximation formula overestimates the ratio of positive to negative charged fractions relative to most measurement results. Ratios for 210Po, 85Kr, and 241Am measured in this study are consistent with those reported by previous measurements for smaller-sized particles (Hussin et al. Citation1983; Adachi et al. Citation1985; Wiedensohler et al. Citation1986; Reischl et al. Citation1996). The overestimation of positive charged fractions can lead to biases for SMPS data inversion since commercial SMPS systems (e.g., the TSI 3936) conventionally measure positively charged particles. More recently, we have used negatively charged particles when measuring sub-10 nm number distributions because their higher charged fractions and CPC counting efficiencies improve counting statistics (Jiang et al. Citation2011). Because it was found that the use of positively charged particles leads to higher uncertainties, Wiedensohler and colleagues at the World Calibration Center for Aerosol Physics also chose to use negatively charged particles for SMPS inter-comparison studies.
FIG. 5. Comparison of singly charged particle fractions measured by different studies and predicted by the approximation formula: (a) negative; (b) positive; (c) the ratio of positive fraction to negative fraction. Corrected values for coefficients a4,1 and a5,2 in Wiedensohler's formula were used.
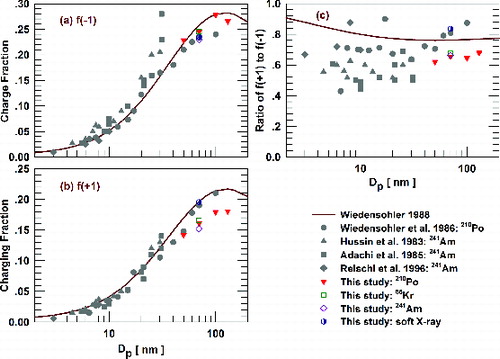
Stationary state charge distributions achieved by bipolar diffusion charging are largely influenced by the relative differences in concentration, mobility, and mass of positive and negative ions. In addition to the absolute values of charge fractions, the relative ratio of f(+1) to f(−1) indicates whether proper ion concentrations and properties (mobility and mass) are used in aerosol charging models. also illustrates the wide variation range of f(+1) to f(−1) ratios reported by different studies. It is possibly related to the fact that ion mass and mobility are distributions (rather than discrete values) which are influenced by species in the carrying gas passing through the neutralizer (Lee et al. Citation2005; Luts et al. Citation2011; Steiner and Reischl Citation2012; Steiner et al. Citation2014). Future studies on bipolar charging theory may incorporate these findings to develop an average stationary state charge distribution. However, the alternative of using different stationary state charge distributions depending on the applications (experimental conditions) might be more scientifically sound, but practically tedious, for electrical mobility size distribution measurement.
4. SUMMARY
Aerosol charge fractions downstream of neutralizers are affected by the ionization method, ion source activity, charger design, and aerosol flowrate. All six neutralizers (PTL 210Po, ADI 210Po, TSI 3077/3077A 85Kr, 3012/3012A 85Kr, 3087 soft X-ray, and Tokyo Dylec 241Am) tested in this study achieve stationary state charge distributions when the source activity is sufficiently high. To achieve stationary charge fractions, a source radioactivity no less than 0.0527 MBq is recommended for 210Po neutralizers. The minimum required activity for 85Kr neturalizers was not definitively established, although our measurements suggest that neutralizing effectiveness may diminish below 70 MBq. The threshold radioactivity for 210Po neutralizers provides some guidance for 241Am neutralizers as well since 210Po and 241Am emit alpha particles with similar energy. These threshold radioactivities were determined using specific neutralizer housings; caution should be exercised when extrapolating them to other neutralizer housing with different designs. Special designs of neutralizer housing can lead to successful achievement of stationary charge distributions at lower level of radioactivity (Hoppel and Frick Citation1990; Vivas et al. Citation2008). A 15-month continuous test indicates that soft X-ray intensity is reproducible within 4%. A longer aging effect on the performance of soft X-ray neutralizer is needed.
Aerosol charge fractions for six neutralizers remain reasonably invariant over a wide range of flowrates, i.e., 3–15 lpm for the 3012/3012A neutralizer and 0.3–5 lpm for the other five neutralizers. For instance, the relative deviation of the singly charged fractions for most neutralizers is within 6%.
The positive charge fractions achieved by the 3087 soft x-ray neutralizer are higher than those by the other five neutralizers using radioactive sources while their negative charge fractions are all in a similar range. A widely used approximation formula in SMPS data inversion appears to overestimate the ratio of positive charged fractions to negative charged fractions. Measured single negatively-charged fractions for the four ionization methods are in reasonable agreement with predictions of Wiedensohler's formula. Charged fractions for singly positively-charged fractions also agree well for the soft x-ray neutralizer, but are noticeably lower than the predicted value for the other three sources, with differences ranging from 18.4% for 85Kr to 25.2% for 210Po for 70 nm particles. These observations demonstrate the needs to better understand ion properties (mobility and mass) and concentrations in order to unambiguously predict positive and negative charge fractions and their ratios downstream of neutralizers and to apply them in aerosol size distribution measurements.
FUNDING
Funding for this research was provided by the US Department of Energy Grant Number DE-SC0006861. Jingkun Jiang acknowledges partial support from National Natural Science Foundation of China (41227805 and 21190054). We acknowledge Dr. Susanne Hering for the loan of the ADI neutralizer housing.
SUPPLEMENTAL MATERIAL
Supplemental data for this article can be accessed on the publisher's website.
SI_141007.zip
Download Zip (715.7 KB)Notes
1 Single and double charged fractions were measured consecutively without disturbing flows while those of opposite polarity were measured in a later run for which the flows were established independent of the first run.
REFERENCES
- Adachi, M., Kousaka, Y., and Okuyama, K. (1985). Unipolar and Bipolar Diffusion Charging of Ultrafine Aerosol-Particles. J. Aerosol Sci., 16(2):109–123.
- Cooper, D. W. and Reist, P. C. (1973). Neutralizing Charged Aerosols with Radioactive Sources. J. Colloid Interface Sci., 45(1):17–26.
- Covert, D., Wiedensohler, A., and Russell, L. (1997). Particle Charging and Transmission Efficiencies of Aerosol Charge Neutralizers. Aerosol Sci. Technol., 27(2):206–214.
- Fuchs, N. A. (1963). On the Stationary Charge Distribution on Aerosol Particles in a Bipolar Ionic Atmosphere. Geofis. Pura. Appl., 56:185–193.
- Gopalakrishnan, R., Meredith, M. J., Larriba-Andaluz, C., and Hogan, C. J. (2013). Brownian Dynamics Determination of the Bipolar Steady State Charge Distribution on Spheres and Non-Spheres in the Transition Regime. J. Aerosol Sci., 63:126–145.
- Gunn, R., and Woessner, R. H. (1956). Measurements of the Systematic Electrification of Aerosols. J. Colloid Sci., 11(3):254–259.
- Han, B., Hudda, N., Ning, Z., Kim, H. J., Kim, Y. J., and Sioutas, C. (2009). A Novel Bipolar Charger for Submicron Aerosol Particles Using Carbon Fiber Ionizers. J. Aerosol Sci., 40(4):285–294.
- Hoppel, W. A., and Frick, G. M. (1986). Ion-Aerosol Attachment Coefficients and the Steady-State Charge Distribution on Aerosols in a Bipolar Ion Environment. Aerosol Sci. Technol., 5(1):1–21.
- Hoppel, W. A., and Frick, G. M. (1990). The Nonequilibrium Character of the Aerosol Charge-Distributions Produced by Neutralizers. Aerosol Sci. Technol., 12(3):471–496.
- Hussin, A., Scheibel, H. G., Becker, K. H., and Porstendorfer, J. (1983). Bipolar Diffusion Charging of Aerosol-Particles .1. Experimental Results Within the Diameter Range 4-30-Nm. J. Aerosol Sci., 14(5):671–677.
- Jiang, J., Chen, M., Kuang, C., Attoui, M., and McMurry, P. H. (2011). Electrical Mobility Spectrometer Using a Diethylene Glycol Condensation Particle Counter for Measurement of Aerosol Size Distributions Down to 1 nm. Aerosol Sci. Technol., 45(4):510–521.
- Jiang, J., Lee, M. H., and Biswas, P. (2007a). Model for Nanoparticle Charging by Diffusion, Direct Photoionization, and Thermionization Mechanisms. J. Electrost., 65(4):209–220.
- Jiang, J. K., Hogan, C. J., Chen, D. R., and Biswas, P. (2007b). Aerosol Charging and Capture in the Nanoparticle Size Range (6–15 nm) by Direct Photoionization and Diffusion Mechanisms. J. Appl. Phys., 102(3):034904.
- Kallinger, P., Steiner, G., and Szymanski, W. W. (2012). Characterization of Four Different Bipolar Charging Devices for Nanoparticle Charge Conditioning. J. Nanopart. Res., 14(6).
- Kimoto, S., Mizota, K., Kanamaru, M., Okuda, H., Okuda, D., and Adachi, M. (2009). Aerosol Charge Neutralization by a Mixing-Type Bipolar Charger Using Corona Discharge at High Pressure. Aerosol Sci. Technol., 43(9):872–880.
- Knoll, G. F. (2010). Radiation Detection and Measurement. John Wiley & Sons, Hoboken, NJ.
- Kwon, S. B., Sakurai, H., Seto, T., and Kim, Y. J. (2006). Charge Neutralization of Submicron Aerosols Using Surface-Discharge Microplasma. J. Aerosol Sci., 37(4):483–499.
- López-Yglesias, X., and Flagan, R. C. (2013). Ion–Aerosol Flux Coefficients and the Steady-State Charge Distribution of Aerosols in a Bipolar Ion Environment. Aerosol Sci. Technol., 47(6):688–704.
- Lee, H. M., Kim, C. S., Shimada, M., and Okuyama, K. (2005). Effects of Mobility Changes and Distribution of Bipolar Ions on Aerosol Nanoparticle Diffusion Charging. J. Chem. Eng. Jpn., 38(7):486–496.
- Liu, B. Y., Pui, D. Y., Kinstley, W. O., and Fisher, W. G. (1987). Aerosol Charging and Neutralization and Electrostatic Discharge in Clean Rooms. J. Environ. Sci., 30(2):42–46.
- Liu, B. Y. H., and Pui, D. Y. H. (1974a). Electrical Neutralization of Aerosols. J. Aerosol Sci., 5(5):465–472.
- Liu, B. Y. H., and Pui, D. Y. H. (1974b). Equilibrium Bipolar Charge Distribution of Aerosols. J. Colloid Interface Sci., 49(2):305–312.
- Luts, A., Parts, T. E., Horrak, U., Junninen, H., and Kulmala, M. (2011). Composition of Negative Air Ions as a Function of Ion Age and Selected Trace Gases: Mass- and Mobility Distribution. J. Aerosol Sci., 42(11):820–838.
- Maricq, M. M. (2008). Bipolar Diffusion Charging of Soot Aggregates. Aerosol Sci. Technol., 42(4):247–254.
- Modesto-Lopez, L. B., Kettleson, E. M., and Biswas, P. (2011). Soft X-Ray Charger (SXC) System for use with Electrospray for Mobility Measurement of Bioaerosols. J. Electrost., 69(4):357–364.
- Qi, C. L., and Kulkarni, P. (2013). Miniature Dual-Corona Ionizer for Bipolar Charging of Aerosol. Aerosol Sci. Technol., 47(1):81–92.
- Rader, D. J., and Mcmurry, P. H. (1986). Application of the Tandem Differential Mobility Analyzer to Studies of Droplet Growth or Evaporation. J. Aerosol Sci., 17(5):771–787.
- Reischl, G. P., Makela, J. M., Karch, R., and Necid, J. (1996). Bipolar Charging of Ultrafine Particles in the Size Range Below 10 nm. J. Aerosol Sci., 27(6):931–949.
- Romay, F. J., Liu, B. Y. H., and Pui, D. Y. H. (1994). A Sonic Jet Corona Ionizer for Electrostatic Discharge and Aerosol Neutralization. Aerosol Sci. Technol., 20(1):31–41.
- Shimada, M., Han, B. W., Okuyama, K., and Otani, Y. (2002). Bipolar Charging of Aerosol Nanoparticles by a Soft X-Ray Photoionizer. J. Chem. Eng. Jpn., 35(8):786–793.
- Steiner, G., Jokinen, T., Junninen, H., Sipilä, M., Petäjä, T., Worsnop, D., Reischl, G. P., and Kulmala, M. (2014). High-Resolution Mobility and Mass Spectrometry of Negative Ions Produced in a 241Am Aerosol Charger. Aerosol Sci. Technol., 48(3):261–270.
- Steiner, G., and Reischl, G. P. (2012). The Effect of Carrier Gas Contaminants on the Charging Probability of Aerosols Under Bipolar Charging Conditions. J. Aerosol Sci., 54:21–31.
- Stolzenburg, M., and McMurry, P. (1988). TDMAFIT User's Manual. Minneapolis, University of Minnesota.
- Stolzenburg, M. R., and McMurry, P. H. (2008). Equations Governing Single and Tandem DMA Configurations and a New Lognormal Approximation to the Transfer Function. Aerosol Sci. Technol., 42(6):421–432.
- Stommel, Y. G., and Riebel, U. (2004). A New Corona Discharge-Based Aerosol Charger for Submicron Particles with Low Initial Charge. J. Aerosol Sci., 35(9):1051–1069.
- Stommel, Y. G., and Riebel, U. (2005). A Corona-Discharge-Based Aerosol Neutralizer Designed for use with the SMPS-System. J. Electrost., 63(6–10):917–921.
- Vivas, M. M., Hontanon, E., and Schmidt-Ott, A. (2008). Design and Evaluation of a Low-Level (0.24 mu Ci) Radioactive Aerosol Charger Based on Am-241. J. Aerosol Sci., 39(3):191–210.
- Wang, S. C., and Flagan, R. C. (1990). Scanning Electrical Mobility Spectrometer. Aerosol Sci. Technol., 13(2):230–240.
- Wang, X., Kaufman, S. L., Sem, G. J., Hama, N., Sakurai, H., Stolzenburg, M., and McMurry, P. (2007). Experimental and Numerical Studies of Particle Transmission Efficiency Through Aerosol Neutralizers. 2007 AAAR Annual Conference.
- Whitby, K. T., and Peterson, C. M. (1965). Electrical Neutralization and Particle Size Measurement of Dye Aerosols. Ind. Eng. Chem. Fundam., 4(1):66–72.
- Wiedensohler, A. (1988). An Approximation of the Bipolar Charge-Distribution for Particles in the Sub-Micron Size Range. J. Aerosol Sci. 19(3):387–389.
- Wiedensohler, A., Birmili, W., Nowak, A., Sonntag, A., Weinhold, K., Merkel, M., Wehner, B., Tuch, T., Pfeifer, S., Fiebig, M., Fjaraa, A. M., Asmi, E., Sellegri, K., Depuy, R., Venzac, H., Villani, P., Laj, P., Aalto, P., Ogren, J. A., Swietlicki, E., Williams, P., Roldin, P., Quincey, P., Huglin, C., Fierz-Schmidhauser, R., Gysel, M., Weingartner, E., Riccobono, F., Santos, S., Gruning, C., Faloon, K., Beddows, D., Harrison, R., Monahan, C., Jennings, S. G., O'Dowd, C. D., Marinoni, A., Horn, H. G., Keck, L., Jiang, J., Scheckman, J., McMurry, P. H., Deng, Z., Zhao, C. S., Moerman, M., Henzing, B., de Leeuw, G., Loschau, G., and Bastian, S. (2012). Mobility Particle Size Spectrometers: Harmonization of Technical Standards and Data Structure to Facilitate High Quality Long-Term Observations of Atmospheric Particle Number Size Distributions. Atmos. Meas. Tech., 5(3):657–685.
- Wiedensohler, A., Lutkemeier, E., Feldpausch, M., and Helsper, C. (1986). Investigation of the Bipolar Charge-Distribution at Various Gas Conditions. J. Aerosol Sci., 17(3):413–416.
- Yun, K. M., Lee, S. Y., Iskandar, F., Okuyama, K., and Tajima, N. (2009). Effect of X-Ray Energy and Ionization Time on the Charging Performance and Nanoparticle Formation of a Soft X-Ray Photoionization Charger. Adv. Powder Technol., 20(6):529–536.