Abstract
The impact of exhaust gas recirculation (including three levels: 0, 10%, and 30%) on engine combustion characteristics, gaseous emissions, and particulate properties (i.e., oxidative reactivity, carbonaceous compositions, size distribution, and nanostructure) was studied on a common rail diesel engine operating at low engine load. The results showed that the lack of oxygen with EGR prolongated ignition delay and the premixed portion of combustion started to rise significantly. Higher EC (accumulation mode) with larger particle size could be observed with increasing EGR from 0 to 30%, which is attributed to the promotion of soot formation with less available oxygen and the inhibition of soot oxidation with low in-cylinder temperature with increasing EGR. The soot nanostructure observation showed that soot changed from smooth surface under 0 EGR to rugose surface under 10% EGR. Moreover, the amorphous core turned larger with increasing EGR. With increasing EGR to 30%, the amorphous core appeared to include the whole primary soot particle. The increase of accessible carbons on the edge sites correlates with the high reactivity with increasing EGR. Through the quantitative analysis of the correlation between the combustion parameters and particle properties, we speculated that in this work, the engine at low load producing very little to no conventional soot or soot-EC coupling with low combustion temperature and short residence time with increasing EGR lead to the soot exhibiting less carbonization level (short fringe length and large fringe curvature) and result in higher reactivity.
Copyright 2015 American Association for Aerosol Research
1. INTRODUCTION
Diesel engine emissions have gained considerable attention due to their health risk and environmental concerns, especially particulate matter and nitrogen oxides (NOx). Numerous investigators have been engaged in controlling diesel emissions by developing emission control technique. The introduction of diesel particulate filters (DPF) in diesel engine is proved to be very efficient for capturing the particles (Johnson et al. 1994). Generally, more than 99% particulate matter (PM) can be removed through DPF. When using a DPF, the trapped particles (mainly composed of soot) must be intermittently removed by oxidation of particulates in order to avoid the filter being clogged, which is called DPF regeneration. DPF regeneration behavior largely depends on the exhaust temperature and soot oxidative activity. The shorter time and the lower temperature to oxidize a given mass of soot are helpful for DPF regeneration. In other words, high reactivity will be more desirable. Therefore, the subject of soot reactivity has recently received considerable attention. It is accepted that soot reactivity is related to the physico-chemical properties of the soot, which is governed by the fuel properties and engine-operating conditions.
The effects of fuel properties on the soot physico-chemical properties and oxidative activity have been the subject of several previous studies. Compared with conventional petroleum diesel, oxygenated diesel, and oxygenated diesel fuel additive are proved to produce soot with high oxidation reactivity (Song et al. Citation2006; Yehliu et al. Citation2012; Gill et al. Citation2012; Lu et al. Citation2012a; Xu et al. Citation2013). The relative amount of initial surface oxygen groups on the primary soot generated from oxygenated fuel have been suggested to explain the high reactivity of the soot (Song et al. Citation2006; Xu et al. Citation2013), while the high reactivity for the soot is attributed to its amorphous nanostructure. The oxidation rate of soot samples for diesel lower than that for biodiesel has been observed by Lu et al. (Citation2012a), which is attributed to smaller primary particle size, more disorder nanostructureand larger volatile fraction. It is observed that soot from biodiesel exhibits a more amorphous nanostructure which enhances the soot oxidation rate. Yehliu et al. (Citation2012) examined the effect of fuel formulation on the nanostructure and reactivity of diesel soot. The authors demonstrated that there is no relation between the surface oxygen content and the soot reactivity, while the soot oxidative reactivity is dominated by the disorder of the carbonaceous nanostructure and consequent increase of accessible carbons on the edge sites. These literature reviews, as well as some work on flame experiment (Vander Wal and Tomasek Citation2003, Citation2004), proved that a strong structure-reactivity relationship presents in soot from diesel engine fueled with various fuels although the chemical composition of the soot (e.g., oxygen-related surface groups and volatile organic component) is also the effective factor on the reactivity.
Besides the fuel formulation, engine operating conditions (e.g., speed, air-fuel ratio and injection strategies) also affect soot characteristics (Lu et al. Citation2012b; Yehliu et al. Citation2013; Xu et al. Citation2014). Lu et al. (Citation2012b) found that the soot exhibits disorder structure at low engine load and high engine speed due to lower in-cylinder residence time. Yehliu et al. (Citation2013) demonstrated the effect of injection timing on soot reactivity and nanostructure and showed that the rate constant of the sample for retarding injection timing (retard 2 CAD) is 2.3 times that for advancing injection timing (advanced 2 CAD), and the soot generated at advancing injection timing may more oxidize and form higher crystalline order, which thus results in a low reactivity. The effects of injection pressure on soot reactivity were recently investigated by Xu et al. (Citation2014). They found that smaller primary soot particles with shorter and flatter graphene layer segments present under higher injection pressure conditions, and soot oxidative reactivity increases with injection pressure. As shown in these literatures and some work on flame experiment (Vander Wal and Tomasek Citation2004; Alfè et al. Citation2010), the in-cylinder conditions (e.g., temperature-time history and the local air-to-fuel ratio) during the soot formation and oxidation would be major responsibility for the distinction.
Exhaust gas recirculation (EGR) is an effective technique to reduce NOx emission from diesel engines because it is able to lower both flame temperature and oxygen concentration in the combustion chamber (Ladommatos et al. Citation1996; Machacon et al. Citation1997; Schubiger et al. Citation2001; Sluder et al. Citation2004; Musculus et al. Citation2013). But the use of EGR has adverse effect on soot emissions from diesel engines. Among a lot of studies of the effects of EGR on soot emissions, most focused on the total mass of soot (Kreso et al. Citation1998; Desantes et al. Citation2000; Nitu et al. Citation2002; Goma et al. Citation2011). Nonetheless, there is very little concern on the effect of EGR on soot nanostructure and oxidative reactivity. Al-Qurashi et al. (Citation2008, Citation2011, Citation2012) recently studied the oxidative reactivity of the soot generated under 0 and 20% EGR and found that highly reactive soot can be achieved via EGR. According to Al-Qurashi et al. (Citation2008, Citation2011, Citation2012), it is speculated that the core of the soot consists of a disordered fraction with high concentration of active sites, which favors oxygen attack and results in highly reactive soot with EGR. This suggested that the effect of EGR on soot regeneration is beneficial for its high oxidative reactivity, which is opposite to the conventional recognition that the application of EGR could lead to the soot emission increase (Ladommatos et al. Citation1996; Machacon et al. Citation1997). However, an opposite result of high EGR rate providing less oxidation reactive soot comparing to the soot of low EGR rate was observed by Iwata et al. (Citation2014) recently. This evokes us to confirm the exact effect of EGR on soot oxidative reactivity. In this article, a detailed analysis of particulate properties (e.g., oxidative reactivity, carbonaceous components, size distribution, and nanostructure) with engine combustion diagnosis has been conducted at low engine load. To the knowledge of the authors, the present study is a unique investigation on the detailed soot structural information depending on EGR level.
2. EXPRIMENTAL SECTION
2.1. Test Engine, Fuel, and Operating Conditions
The experiment was conducted on a 2002 model-year, 4-cylinder diesel engine equipped with turbocharger and high pressure common rail injection system. The main specifications of the engine are listed in . The engine used in this study was detailed in the previous literature (Xu et al. Citation2014). A simple description is shown here. The engine was coupled with an eddy-current dynamometer which was available for measuring and adjusting the engine speed and torque. In-cylinder pressure was acquired by a Kistler 6125B piezoelectric pressure transducer with a resolution of 0.5 crank angle and recorded by a combustion analyzer (D2T, France). For each measuring point, the pressure trace was obtained by averaging the recorded pressure data of 200 consecutive cycles. The heat release rate (HRR) and the gas mean temperature (GMT) were calculated basing on the first law of thermodynamics as well as the perfect gas equation of state according to the experimental in-cylinder pressure and averaged over 200 cycles. The calculation options for GMT included intake pressure and intake temperature based on the software request. The original diesel engine was equipped with a low pressure loop EGR. The exhaust was introduced into the intake pipe using the back pressure created by the throttle valve in the exhaust pipe. The exhaust was cooled by means of water cooled EGR cooler before it passed into the intake air. A diesel particulate filter coated with platinum group metals (CDPF) was integrated into the EGR loop to remove the exhaust particles from being recycled back into the intake and avoiding destroying turbocharger. Different EGR rates could be achieved by manual opening/closing EGR valve. An open access electronic control unit (ECU) was used to control the common rail pressure, the fuel delivery per cycle and the start of injection.
Table 1 Engine specifications
The current work was carried out by using a commercial diesel fuel, which contained 47 ppm sulfur and meets the 50 ppm fuel sulfur limit of Chinese Phase IV Emission Regulation for Diesel Vehicle. The lubricating oil is a 15 W-40 full mineral oil with a measured sulfur content of 2687 ppm. Three engine operating conditions are listed in . The soot samples were collected at an engine speed of 1450 rpm and torque of 112 N m (25% load). The soot samples were also collected at three EGR rates (0, 10%, and 30%). The fuel injection pressure was set at 80 MPa, and the injection timing was kept constant at top dead center.
Table 2 Parameters of the engine operating at different engine loads
In order to ensure the repeatability and comparability of the measurements, the temperatures of intake air, coolant, and lubricant oil were kept in the ranges of 40 ± 2°C, 80 ± 2°C, and 90 ± 2°C, respectively. Data were recorded after the engine had reached the steady state under each operating condition. Moreover, each operating condition was repeated twice to ensure that the results were repeatable within the experimental uncertainties. The standard errors for NOx, HC, and CO are less than 2.5 ppm and 0.4 g/Kw h for brake-specific fuel consumption (BSFC).
2.2. Particle Sample Collection and Characterization
The schematic diagram of the dilution and sampling system is shown in . The hot diesel exhaust particles were drawn through an insulated and heated stainless steel tube into the primary diluter where they were diluted with a steady flow of clean cooled air filtered by a high efficiency particulate filter (HEPA). The insulated sampling line was heated by a temperature controller to maintain a surface temperature of 190°C to prevent thermophoretic deposition of semi-volatile compounds on the tube wall. The particle samples were collected on the quartz fiber filter (Whatman Model 1851-047) for 30–60 min after passing through a primary diluter with dilution ratio of 5–6. A scanning mobility particle sizer (SMPS, TSI Model 3034) was used in measuring the size distribution of the particles in the range from 10 to 487 nm. A small fraction of diluted sample was further diluted in a second diluter, which meets the concentration and temperature limit of SMPS.
All quartz filters were pre-baked at 550°C for 8 h and stored in baked aluminum foil prior to deployment. The particle samples were analyzed by thermogravimetric analysis instrument (TGA) for soot oxidative reactivity, high resolution transmission electron microscopy (HRTEM) for soot nanostructure, and thermo/optical carbon analyzer for organic carbon/element carbon (OC/EC) components.
TGA (TA Model Q5000IR) was used to measure weight changes of each sample via a method of temperature programmed oxidation. The air flow rate was 100 mL/min and the temperature was raised to 800°C with a heating rate of 20 °C/min.
The PM carbonaceous compositions were measured via a thermo/optical carbon analyzer (DRI Model 2001). With this method, the oven temperature was stepwise heated to 140°C, 280°C, 480°C, and 580°C in the helium environment, which produced OC1, OC2, OC3, and OC4 thermocarbon fractions, respectively. Then, 2% oxygen and 98% helium were introduced into the system, and the oven was further heated to 580°C, 740°C, and 840°C for producing EC1, EC2, and EC3, respectively. The calibration of OC4 and EC1 by Pyrolyzed OC, namely POC, is need.
The particle samples were ultrasonically dispersed in ethanol for 10 min in the preparation for HRTEM observation. The ethanol solution containing particle samples was dripped onto a copper grid with a lacey carbon film. The substrate was then dried in an infrared oven. The similar ultrasonic dispersion method has been conducted by several groups (Vander Wal and Mueller et al. Citation2006; Alfè et al. Citation2009; Liati et al. Citation2013). They suggested that the soot aggregates are mechanically broken and results in the corresponding information of losing radius of gyration, number density, and fractal dimension. While the individual particles can be better studied by TEM because in some cases the particles collected directly on TEM grids failed to be identified individually. Although there is no comment on the effect of ultrasonic dispersion on micro-structure of the particles, the studies about the ultrasonic dispersion of carbon nanotubes in the ethanol (Yoshio et al. Citation2011) and texanol (Darsono et al. Citation2008) indicated that ultrasonic dispersion evidently affects the aggregation, while slightly affects the nanostructure of the rod-like structure of single nanotubes. A field emission HRTEM (JOEL Model JEM-2100F) operated on 200 kV with a point-to-point resolution of 0.23 nm was employed to acquire HRTEM image. Above 500,000× magnification was applied to observe the primary soot nanostructure. HRTEM images were digitized and analyzed using the image processing software, and the detailed description of the method can be referred in Lu et al. (Citation2012a). The images were analyzed by using commercial image processing software Image-Pro Plus 6.0 (Media Cybernetics). Parameters of fringe length and tortuosity were quantified to describe the nanostructures of particles under different operating conditions. Fringe length is acquired by measuring the physical extent of the atomic carbon layer planes as seen in a HRTEM image, and tortuosity is the ratio of the actual fringe length to the straight-line distance between the endpoints of the carbon layer (fringe). For each testing point, there were about 20–30 HRTEM images of different primary particles selected for the nanostructure parameter statistics.
3. RESULTS AND DISCUSSIONS
3.1. Combustion Characteristics and Gaseous Emission
The in-cylinder pressure, heat release rate (HRR), and gas mean temperature (GMT) are shown in . The corresponding combustion parameters and gaseous emission are listed in . It is shown that the ignition delay (ID) as well as the HRR remains unchanged for EGR rate up to 10%,a sharp increase of ID is presented and consequently an evident enhancement of the peak value of HRR appears when EGR increases from 10% to 30%. A similar result has been observed by Schubiger et al. (Citation2001). They showed that the combustion is dominated by the premixed control at low engine load, and the premixed portion of combustion remains unchanged for the EGR below 10%, while the premixed portion increases with increasing EGR rate for the EGR above 10%. Using an optically accessible diesel engine, Huestis et al. (Citation2007) showed that the increase of EGR promotes the pre-mixed controlled combustion at low-to-moderate EGR level. Charge dilution decreases the peak in-cylinder pressure due to the higher heat capacity of the diluent gases as well as slower reaction rates during the premixed combustion, which results in low in-cylinder GMT. For example, for 0, 10%, and 30% EGR, the air fuel ratios were 57.6, 38.2, and 33.3 and GMT were 1587, 1554, 1512 K, respectively. With increasing EGR rate, the temperature of the burned gas zone drops. This is due to higher heat capacity of the recirculated gas compared to fresh air, thereby reducing the combustion temperature. In the case of dilution by EGR, the oxygen concentration is also decreased, which further slows NOx formation kinetics. (Huestis et al. Citation2007; Schubiger et al. Citation2001; Musculus et al. Citation2013). For example, NOx emission decreases more than 90% as EGR increases from 0 to 30%. The reduction in air fuel ratio reduces the availability of oxygen amount for fuel combustion, and CO emission eventually increases (Deepak et al. Citation2006). For over 10% EGR, CO emission increases rapidly, and this may be due to incomplete combustion as a result of high amount of EGR inside the combustion chamber. Therefore, it is clear that the reduction in the oxygen concentration is main cause of increase in all the incomplete combustion products. The BSFC remains unchanged for EGR below 10%, while a sharp rise with increasing EGR from 10% to 30% can be observed, and the BSFCs, at 0, 10%, and 30% EGR rates, are 265.3, 266.8, and 276.2 g/kWh, respectively. Under low-load conditions, very low NOx and PM emissions can be obtained at high EGR rates, because the combustion is delayed due to high dilution. This is accompanied with an increase of BSFC and incomplete combustion products, e.g., CO and HC emissions (Sluder et al. Citation2004; Musculus et al. Citation2013).
3.2. Soot Oxidative Reactivity
TGA was used to investigate soot mass variation via a method of temperature-programmed oxidation. The normalized nonvolatile substance mass loss curves are presented in . The nonvolatile substance lost their mass rapidly in the temperature range of 550–650°C. As the temperature is high enough, the mass loss curves turn level which indicates the soot sample loses almost all the mass. shows the corresponding differential thermal gravity (DTG) curves, which are used to describe the ignition temperature of the different samples. Here Tmax is defined as the temperature where soot oxidation rate is maximized. It is clear that the application of EGR exhibits evident effect on soot thermal stability. For example, Tmax are 587°C, 580°C, and 565°C for 0, 10%, and 30% EGR, respectively. The similar results were shown by Al-Qurashi et al. (Citation2011; Citation2012), the authors hypothesized that the EGR dilution effect reduces the flame temperature and consequently reduces the degree of carbonization, which yields soot with high concentration of actives sites (high initial active surface area has been observed) and results in highly oxidative reactivity. Concerning the reduction potential in soot oxidative reactivity with EGR at low engine load, the analysis of carbonaceous components by thermo/optical carbon analyzer, size distribution by SMPS, and nanostructure by HRTEM combined with engine combustion diagnosis has been conducted as follows.
3.3. Particle Carbonaceous Components and Size Distribution
Diesel particle is dominated by carbonaceous components, including OC and EC. The EC (EC1-EC3) and OC (OC1-OC4) in the particle samples at different EGR rates were observed through thermo/optical carbon analyzer (in ). According to Watson et al. (Citation1994) and Han (Citation2007), EC1 is generally considered as the production of the direct fuel pyrolysis at relatively low combustion temperature, which is defined as char-EC, while EC2 and EC3 are formed via gas-to-particle conversion at high combustion temperature and EC2+EC3 is defined as soot-EC. Similarly, the OC composition can be also separated into two categories of volatile organic compound (OC1) and Hightemp-OC (OC2+OC3+OC4) according to the heating temperature.
FIG. 4. Effects of EGR on (a) specific emissions and (b) mass fractions of carbonaceous compositions.
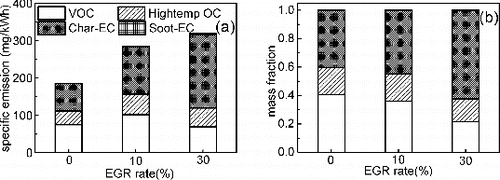
It is shown that an evident positive correlation between the BSFC and EC with increasing EGR. For example, the specific EC emission increases 71.4% and 164.3% with increasing EGR from 0 to 10% and 30%, respectively. As it was previously found that the onset of in-cylinder soot is delayed with decreasing intake oxygen concentration, the peak soot increases as the intake oxygen concentration decreases and the tail of the soot mass curves persists later in the combustion stroke (Huestis et al. Citation2007). The high EC emission with increasing EGR is the result of the suppression of soot oxidation with less available oxygen and the low in-cylinder temperature. Moreover, it is found that char-EC is dominated in the total EC, where is scarcely soot-EC in the sample in this work. Zhang et al. (Citation2011) and Lu et al. (Citation2012c) found that the low soot-EC emission presents at low engine load while high soot-EC presents at high engine load. It is speculated that the low in-cylinder combustion temperature under all operating conditions in this work allows the inhibition of the soot-EC formation.
It is shown that mass fraction of OC evidently decreases with increasing EGR, Kreso et al. (Citation1998) found that the OC fraction in the exhaust bulk particles collected from a Cummins M11 diesel engine exhibits 70% and 30% reduction as EGR increased from 0 to 16% at 75% and 25% loads, respectively. The soot reactivity is related to active sites, an evident positive correlation between OC fraction (adsorbed hydrocarbons) and oxidative reactivity is attributed to the opened-up pore area after OC fraction evaporating and results in more available active sites (Collura et al. Citation2005).
The effect of EGR on the particle number and surface size distribution is shown in . Increasing EGR from 0 to 30% leads to decrease of nucleation mode particles (diameter below 50 nm, NM), while increase accumulation mode particles (diameter above 50 nm, AM), especially for those particles with the diameter above 100 nm. The similar observations have been done by Kreso et al. (Citation1998) and Desantes et al. (2000). Because EGR reduces oxygen concentration in the cylinder as a result of the recirculation of combustion products, combustion deteriorated in cylinder at lower peak temperature, and soot nuclei enhanced under these conditions. With more available soot nuclei, the particle chains could grow longer aggregations (AM) in coagulation process. For example, the peak diameter of the particle number concentration size distribution increases about 40 nm with increasing EGR from 0 to 10% and 30%. The increase trend of AM is identical with the EC emission, which suggested that EC compounds are dominated in AM. The particle surface area size distribution is shown in . It is shown that the total particle surface area (TSA) holds no change with EGR below 10%, while TSA increases 1.5 times under 30% EGR. Generally, the active site, the key effective factor on particle oxidative reactivity, is high affinity for TSA and the initial active surface area. Khan (Citation1987) showed that the initial active surface area has positive relationship with soot reactivity. On the contrary, TSA showed an inverse relationship with soot reactivity.
3.4. Soot Nanostructure
The representative HRTEM images at different EGR rates are reported in . Both of the primary particles sampled for 0 and 10% EGR exhibit a shell-core structure, which is reported mostly in previous diesel soot researchers (Dobbins et al. Citation1996; Hurt et al. Citation2000; Song et al. Citation2006; Yehliu et al. Citation2012; Lu et al. Citation2012b; Xu et al. Citation2014). The distinct basic structure units (BSU) and surface roughness can be observed for 0 and 10% EGR, and the innermost amorphous part (core) constitutes a relatively large part of particle volume for 10% EGR soot. It is also observed that spherical particle for 0 EGR exhibits a very smooth outer surface with few irregularities, while the surface is dominated by irregularities for 10% EGR soot. A similar gross morphology of carbon black has been observed from a stoichiometric low temperature hydrocarbon flame (Su et al. Citation2004). The authors suggested that the smooth outer surface of the particles indicates a long reaction time to reach a minimum energy situation or a post synthetic oxidative episode to burn away the surface irregularities. Therefore, the surface roughness of the 10% EGR soot is possibly the result of the temperature effect. With increasing EGR to 30%, the amorphous core appears whole primary soot. The disordered carbon is the typical structure formed in low temperature atmosphere and immature soot in diesel engine exhaust (Lu et al. Citation2012b; Xu et al. Citation2014) and flame (Vander Wal and Tomasek Citation2004; Alfè et al. Citation2009). As explained by other studies (Vander Wal and Tomasek Citation2003; Müller et al. Citation2005; Xu et al. Citation2014), small graphene layer segments at low engine load with high EGR increase edge site carbon atoms whose reactivity is 10-100-fold higher than those of carbon atoms within basal plane. Therefore, high EGR increases the ratio of edge site to basal plane carbon atoms, which provides more opportunities for oxidants to react with high reactivity edge sit carbon atoms, and results in enhancement of soot reactivity.
The soot nanostructure feature (fringe length and fringe tortuosity) was quantified through an image processing method, and fringes shorter than 0.4 nm were discarded as artifacts in the image analysis process (Dobbins et al. Citation1996; Hurt et al. Citation2000). The histograms of fringe length and tortuosity for each sample are presented in and . The average fringe length decreases with increase of EGR, and the average value is 1.14, 1.07, and 0.97 nm at 0, 10%, and 30% EGR, respectively. On the contrary, larger fringe tortuosity is presented when EGR is increased. The fringe tortuosity is 1.25, 1.28, and 1.29 at 0, 10%, and 30% EGR, respectively. The graphene layer segment is formed during soot nucleation and growth processes. With addition of soot precursors to graphene layer, the segment can be extended and be grouped together within stacks. Both of fringe length and tortuosity, which are referred as indicators of soot carbonization level, are strongly dependent on synthesis conditions, i.e., temperature and reaction time (Vander Wal and Tomasek Citation2004; Song et al. Citation2006; Alfè et al. Citation2009; Yehliu et al. Citation2012). The effect of EGR on fringe length is speculated as follows: The low-intake oxygen concentration promotes the formation of soot precursor and the low combustion suppresses the oxidation of soot, which leads to the extended graphene segment. On the contrary, the onset of in-cylinder soot is delayed, and the shorter-resident time during the combustion suppresses the longer graphene segment by coagulation and growth processes. The competition of these two effects illustrates the relatively smaller fringe lengths with increasing EGR. With regard to fringe tortousity, as stated by Vander Wal and Tomasek (Citation2004), curvature or tortuosity rise from five-membered rings within the aromatic framework. The amount of curvature reflects the competitive pathways producing C5-containing and six-membered ring PAHs. Because of the higher thermodynamic stability of six-membered ring PAHs, low combustion temperature with the application of EGR may favor five-membered ring PAHs production through fuel pyrolysis, and hence results in more curvature in the soot nanostructure.
Carbonization rate has been recognized as a crucial effect factor on soot reactivity. Extended graphene layers with a short radius of curvature represent the soot with high carbonization level, which present high thermodynamic stability and less oxidative reactivity (Hurt et al. Citation2000; Dobbins et al. Citation1996). According to the hypothesis by Hurt et al. (Citation2000), there is a threshold molecular weight of soot precursor for a spontaneous disorder/order transition during pyrolysis, and it is expected that high temperature results in soot precursors with higher molecular weight. Therefore, it is speculated that EGR prevents the conditions leading to soot maturation since it decreases combustion temperature. Dobbins et al. (Citation1996) presented the correlation between the carbonization time and combustion temperature, and suggested that the time required for the carbonization of soot precursor materials increases exponentially with decreasing flame combustion temperature. It suggested that the carbonization level is dependent on the relevant timescale for the residence time of the soot in the flame and the duration of the whole combustion event. The soot nanostructure evolution dependent on temperature-time history has been widely studied by flame and engine experiment. A general recognition is that high temperature and long residence time promote the soot with order structure. It is shown that in-cylinder combustion temperature is generally less than 1300°C when the engine operates at low engine load in this work, which inhibits the formation of conventional soot or soot-EC. Therefore, it is assumed that the low in-cylinder temperature, relative short carbonization time and the low soot-EC concentration cause soot presenting more disorder structure with increasing EGR.
It is emphasized that a detailed particle properties, i.e., oxidative reactivity, carbonaceous compositions, size distribution, and nanostructure information has been studied in this study. In the meantime, a unique investigation on the detailed soot structural information depending on EGR level has been conducted in this study. It is shown that some adverse effect of EGR on particle emission, e.g., higher EC and accumulation mode particle presented with the application of EGR rises the risk of the DPF being clogged. However, a favorable structure characteristic for the enhancement of the particle oxidation reactivity has been observed, that means the particle exhibiting less carbonization level (short fringe length and large fringe curvature) with increasing EGR, Which is the result of low in-cylinder temperature with the application of EGR. We need point out that our results are suitable to the experimental condition in this study, although the identical results have also been observed by other groups (Al-Qurashi et al. Citation2008, Citation2011; Jung et al. Citation2013), even with the distinct EGR loop type and injection modes. It must be carefully to use this conclusion if the distinct experimental condition occurs, e.g., high engine load, after-treatment configuration, alternative fuels, etc.
4. CONCLUSIONS
In the present study, the impact of exhaust gas recirculation (EGR, including three levels: 0, 10%, and 30%) on engine combustion characteristics, gas emissions, and particle properties (i.e., oxidative reactivity, carbonaceous components, size distribution, and nanostructure) was studied on a common rail diesel engine operating at low engine load. Some main conclusions of the investigation were drawn as follows:
The results showed that the lack of oxygen with EGR prolongates ignition delay and the premixed portion of combustion starts to rise significantly. Consequently, the in-cylinder pressure and gas mean temperature decrease, hence result in the incomplete combustion products, e.g., increase of CO and HC emissions.
Higher EC (accumulation mode) with larger particle size could be observed with increasing EGR from 0 to 30%, which is attributed to the promotion of soot formation with less available oxygen and the inhibition of soot oxidation in low in-cylinder temperature with increasing EGR.
The soot nanostructure observation showed that soot changes from smooth surface under 0% EGR to rugosity surface under 10% EGR. Moreover, the amorphous core turns larger with increasing EGR. With a further increase EGR to 30%, the amorphous core appears whole primary soot. The increase of accessible carbons on the edge sites correlates with high reactivity with increasing EGR.
Through the quantitative analysis of correlation between the combustion parameters and particle properties, we speculated that in this work, the engine at low load producing very little to no conventional soot or soot-EC coupling with low combustion temperature and short-resident time with increasing EGR lead to the soot exhibiting less carbonization level (short fringe length and large fringe curvature) and result in higher reactivity.
FUNDING
The authors would like to thank Major Projects of the International Cooperation and Exchanges National Natural Science Foundation of China (No. 51210010) and Special Science and Technology Cooperation Projects of Chinese Mainland and Hong Kong of the Ministry of Science and Technology, China (No. 2014DFH90020) for financial support to this study.
REFERENCES
- Alfè, M., Apicella, B., Barbella, Rouzaud, R. J., Tregrossi, A., and Ciajolo, A. (2009). Structure–Property Relationship in Nanostructures of Young and Mature Soot in Premixed Flames. Proc. Combust. Inst., 32:697–704.
- Alfè, M., Apicella, B., Rouzaud, J., Tregrossi, A., and Ciajolo, A. (2010). The Effect of Temperature on Soot Properties in Premixed Methane Flames. Combust. Flame, 157:1959–1965.
- Al-Qurashi, K., Lueking, A., and Boehman, A. (2008). Impact of Exhaust Gas Recirculation (EGR) on the Oxidative Reactivity of Diesel Engine Soot. Combust. Flame,155:675–695.
- Al-Qurashi, K., Lueking, A., and Boehman, A. (2011). The Deconvolution of the Thermal, Dilution, and Chemical Effects of Exhaust Gas Recirculation (EGR) on the Reactivity of Engine and Flame Soot. Combust. Flame, 158:1696–1704.
- Al-Qurashi, K., Zhang, Y., and Boehman, A. (2012). Impact of Intake CO2 Addition and Exhaust Gas Recirculation on NOx Emissions and Soot Reactivity in a Common Rail Diesel Engine. Energy Fuels, 26:6098–6105.
- Collura, S., Chaoui, N. Azambre, B., Finqueneisel, G., Heintz, O., Krzton, A., and Weber, J. (2005). Influence of the Soluble Organic Fraction on the Thermal Behaviour, Texture and Surface Chemistry of Diesel Exhaust Soot. Carbon, 43:605–613.
- Darsono, N., Yoon, D., and Kim, J. (2008) Milling and Dispersion of Mlti-Walled Carbon Nanotubes in Texanol. Appl. Surf. Sci., 254:3412–3419.
- Deepak, A., Shailendra S. and Agarwal, A. K. (2006). Experimental Investigation of Control of NOx Emissions in Biodiesel-Fueled Compression Ignition Engine. Renew. Energ., 31:2356–2369.
- Desantes, J., Arregle, J. S., and Lejeune, M. M. (2000). Influence of The EGR Rate, Oxygen Concentration and Equivalence Fuel/Air Ratio on the Combustion Behavior and Pollutant Emissions of a Heavy-Duty Diesel Engine. SAE Technical Paper Series No. 2000-01-1813. SAE International, Warrendale, PA.
- Dobbins, R. A., Govatzidakis, G. J., Lu, W., Schwartzman, A. F., and Fletcher, R. A. (1996). Carbonization Rate of Soot Precursor Particles. Combust. Sci. Technol., 121(1-6):103–121.
- Gill, S., Tsolakis, A., Herreros, J., and York, A. (2012). Diesel Emissions Improvements Through the Use of Biodiesel or Oxygenated Blending Components. Fuel, 95:578–586.
- Goma, M., Alimin, A., and Kamarudin, K. (2011). Trade-off Between NOx, Soot and EGR Rate for an IDI Diesel Engine Fuelled with JB5. J. Appl. Sci., 11(11):1987–1993.
- Han, Y. (2007). Evaluation of the Thermal/Optical Reflectance Method for Discrimination Between Char- and Soot-EC. Chemosphere, 69:569–574.
- Huestis, E., Erickson, P. A., and Musculus., M. P. B. (2007). In-Cylinder and Exhaust Soot in Low-Temperature Combustion Using a Wide-Range of EGR in a Heavy-Duty Diesel Engine. SAE Technical Paper Series No. 2007-01-4017. SAE International, Warrendale, PA.
- Hurt, R. H., Crawford, G. P., and Shim H.-S. (2000). Equilibrium Nanostructure of Primary Soot Particles. Proc. Combust. Inst., 28(2):2539–2546.
- Iwata, H., Konstandopoulos, A., Nakamura, K., Ogyu, K., and Ohno, K. (2014) Experimental Study of Physical and Chemical Properties of Soot under Several EGR Conditions. SAE Technical Paper Series No. 2014-01-1593. SAE International, Warrendale, PA.
- Johnson, J. H., Bagley, S. T., Gratz, L. D., and Leddy, D. G. (1994). A Review of Diesel Particulate Control Technology and Emission Effects. SAE Technical Paper Series No. 940233. SAE International, Warrendale, PA.
- Jung, Y., Qi, D., and Bae, C. (2013) Assessment of Soot Particles in an Exhaust Gas for Low Temperature Diesel Combustion with High EGR in a Heavy Duty Compression Ignition Engine. SAE Technical Paper Series No. 2013-01-2572. SAE International, Warrendale, PA.
- Khan, M. R. (1987). Significance of Char Active Surface Area for Appraising the Reactivity of Low- and High-Temperature Chars. Fuel, 66 (12):1626–1634.
- Kreso, A., Johnson, J., Gratz, L., Bagley, S., and Leddy, D. (1998). A Study of the Effects of Exhaust Gas Recirculation on Heavy-Duty Diesel Engine Emissions. SAE Technical Paper Series No. 981422. SAE International, Warrendale, PA.
- Ladommatos, N., Balian, R., Horrocks, R., and Cooper, L. (1996). The Effect of Exhaust Gas Recirculation on Combustion and NOx Emissions in a High-Speed Direct-Injection Diesel Engine. SAE Technical Paper Series No. 960840. SAE International, Warrendale, PA.
- Liati, A., Eggenschwiler, P., Schreiber, D., Zelenay, V., Ammann, M. (2013)Variations in Diesel Soot Reactivity along the Exhaust After-treatment System, Based on the Morphology and Nanostructure of Primary Soot Particles. Combust. Flame, 160:671–681.
- Lu, T., Cheung, C. S., and Huang, Z. (2012a). Investigation on Particulate Oxidation from a DI Diesel Engine Fueled with Three Fuels. Aerosol Sci. Technol. 46:1349–1358.
- Lu, T., Cheung, C. S., and Huang, Z. (2012b). Effects of Engine Operating Conditions on the Size and Nanostructure Of Diesel Engine Particles. J. Aerosol Sci., 47:27–38.
- Lu, T., Huang, Z., Cheung, C. S., and Ma, J. (2012c). Size Distribution of EC, OC And Particle-Phase PAHs Emissions from a Diesel Engine Fueled With Three Fuels. Sci. Total. Environ., 438:33–41.
- Machacon, H., Shiga, S., Karasawa, and Nakamura, T. H. (1997). Effect of EGR on the Particulate and Its Component Emissions in a D.I. Diesel Engine. Bull. M.E.S.J., 25:77–83.
- Müller, J. O., Su, D. S., Jentoft, R. E., Kröhnert, J., Jentoft, F. C., and Schlögl R. (2005). Morphology-controlled Reactivity of Carbonaceous Materials towards Oxidation. Catal. Today, 102–103:259–265.
- Musculus, M., Miles, P., and Pickett, L. (2013) Conceptual Models for Partially Premixed Low-temperature Diesel Combustion. Prog. Energy Combus. Sci., 39:246–283.
- Nitu, B., Singh, I., Zhong, L., Badreshany, K., and Henein, N. (2002). Bryzik,W. Effect of EGR on Autoignition, Combustion, Regulated Emissions and Aldehydes in DI Diesel Engines. SAE Technical Paper Series No. 2002-01-1153. SAE International, Warrendale, PA.
- Schubiger, R., Bertola, A., and Boulouchos, K. (2001). Influence of EGR on Combustion and Exhaust Emissions of Heavy Duty DI-Diesel Engines Equipped with Common-Rail Injection Systems. SAE Technical Paper Series No. 2001-01-3497. SAE International, Warrendale, PA.
- Sluder, C., Wagner, R., Lewis, S., and Storey, J. (2004). Exhaust Chemistry of Low-NOX, Low-PM Diesel Combustion. SAE Technical Paper Series No. 2004-01-0114. SAE International, Warrendale, PA.
- Song, J., Alam, M., Boehman, A., and Kim, U. (2006). Examination of the Oxidation Behavior of Biodiesel Soot. Combust. Flame, 146:589–604.
- Su, D. S., Müller, J.O., Jentoft, R.E., Rothe, D., Jacob, E. and Schlögl., R. (2004). Fullerene-like Soot from EURO-IV Diesel Engine: Consequences for Catalytic Automotive Pollution Control. Top. Catal., 30/31:241–245.
- Vander Wal, R. L., and Mueller, C. J. (2006). Initial Investigation of Effects of Fuel Oxygenation on Nanostructure of Soot from a Direct-Injection Diesel Engine. Energ. Fuels, 20(6):2364–2369.
- Vander Wal, R. L., and Tomasek, A. J. (2003). Soot Oxidation: Dependence Upon Initial Nanostructure. Combust. Flame, 134(1):1–9.
- Vander Wal, R. L., and Tomasek, A. J. (2004). Soot Nanostructure: Dependence upon Synthesis Conditions. Combust. Flame 136:129–140.
- Watson, J., Chow, J., and Lowenthal, D. (1994). Differences in the Carbon Composition of Source Profiles for Diesel- and Gasoline-Powered Vehicles. Atmos. Environ., 28:2493–2505.
- Xu, Z., Li, X. L, Guan, C., and Huang, Z. (2013). Characteristics of Exhaust Diesel Particles from Different Oxygenated Fuels. Energ. Fuels, 27 (12):7579–7586.
- Xu, Z., Li, X. L., Guan, C., and Huang, Z. (2014). Effects of Injection Pressure on Diesel Engine Particle Physico-Chemical Properties. Aerosol. Sci. Technol. 48(2):128–138.
- Yehliu, K., Armas, O., Vander Wal, R. L., and Boehman, A. (2012). Impact of Fuel Formulation on The Nanostructure and Reactivity of Diesel Soot. Combust. Flame, 159:3597–3606.
- Yehliu K., Armas, O., Wal, R., and Boehman, A. (2013). Impact of Engine Operating Modes and Combustion Phasing on the Reactivity of Diesel Soot. Combust. Flame, 160:682–691.
- Yoshio, S., Tatami, J., Yamakawa, T., Wakihara, T., Komey, K., Meguro, T., Aramaki, K., and Yasuda, K. (2011) Dispersion of Carbon Nanotubes in Ethanol by a Bead Milling Process. Carbon, 494131–4137.
- Zhang, J., He, K., Shi, X., and Zhao, Y. (2011). Comparison of Particle Emissions from an Engine Operating on Biodiesel and Petroleum Diesel. Fuel, 90:2089–2097.